Recent Advances of Magnetic Nanomaterials in Bone Tissue Repair
- 1Department of Orthopedic, First Affiliated Hospital of Zhengzhou University, Zhengzhou, China
- 2Department of Pediatrics, Peking University Third Hospital, Beijing, China
- 3Department of Orthopedic, Peking University Third Hospital, Beijing, China
- 4Beijing National Laboratory for Molecular Sciences, State Key Laboratory of Polymer Physics & Chemistry, Institute of Chemistry, Chinese Academy of Sciences, Beijing, China
- 5University of Chinese Academy of Sciences, Beijing, China
- 6CED Education, North Carolina State University, Raleigh, NC, United States
- 7School of Chemistry and Materials Science, Ludong University, Yantai, China
The magnetic field has been proven to enhance bone tissue repair by affecting cell metabolic behavior. Magnetic nanoparticles are used as biomaterials due to their unique magnetic properties and good biocompatibility. Through endocytosis, entering the cell makes it easier to affect the physiological function of the cell. Once the magnetic particles are exposed to an external magnetic field, they will be rapidly magnetized. The magnetic particles and the magnetic field work together to enhance the effectiveness of their bone tissue repair treatment. This article reviews the common synthesis methods, the mechanism, and application of magnetic nanomaterials in the field of bone tissue repair.
Introduction
Bone is a natural complex of inorganic and organic materials (Abou Neel et al., 2016; Loi et al., 2016; Wang et al., 2020). The main component of the inorganic material is crystalline hydroxyapatite while the organic substance is mainly fibrous collagen (Loi et al., 2016). Bone has the ability to regenerate and repair itself. Although for bone defects caused by external damage or due to bone diseases, tumors, and abnormal bone growth, the self-repairing ability of the bone alone cannot achieve the purpose of healing (Loi et al., 2016; Michalski and McCauley, 2017). It is necessary to resort to medical materials including autologous bone tissue, allogeneic bone tissue, and bone tissue substitutes. Either method requires the regeneration of local bone tissue. In this process, external stimulations including stress stimulation, chemical stimulation, biological factor stimulation, magnetic field, and electric field are considered as necessary conditions (Abou Neel et al., 2016; Loi et al., 2016; Michalski and McCauley, 2017; Debnath et al., 2018).
The magnetic field has been proven to enhance bone tissue repair by affecting cell metabolic behavior (Iwasa and Reddi, 2018; He et al., 2019; Liu H. Y. et al., 2020). In recent research, iron is the most common material used with its para-magnetism (Luo et al., 2018; Xia et al., 2018; Yu et al., 2019). The unpaired electrons of the outermost layer spin to make the atom maintain a certain magnetic moment. This atomic magnetic moment is arranged along the magnetic field under the action of an external magnetic field, showing a weak magnetic force that conforms to the magnetic field (Iwasa and Reddi, 2018; Luo et al., 2018). This substance is called paramagnetic substance. Ferromagnetic substances have atomic magnetic moments composed of unpaired spin electrons (Iwasa and Reddi, 2018). In the absence of a magnetic field, the atomic magnetic moments are also neatly arranged, showing strong magnetism to the outside (Luo et al., 2018; Xia et al., 2018; He et al., 2019). Containing a small amount of paramagnetic iron, the bone is magnetically conductive (Luo et al., 2018; Xia et al., 2018; Yu et al., 2019).
Magnetic nanoparticles (MNPs) are used as biomaterials due to their unique magnetic properties and good biocompatibility (Aliramaji et al., 2017; Brett et al., 2017). Recently, they have been widely applied in drug transportation, magnetic hyperthermia, nuclear magnetic imaging, and biological separation (Li et al., 2016; Jia et al., 2019; Nejadnik et al., 2019). The magnetic particles are slowly deposited on the surface of the cell membrane under the action of the magnetic field. The cells engulf the magnetic particles through endocytosis. Entering the cell makes it easier to affect the physiological function of the cell (Theruvath et al., 2018; Xia et al., 2018). If a magnetic field is applied, each magnetic particle will become a magnetic source, which will enable the magnetic scaffold material to play the role of bone tissue repair therapy. Once the magnetic particles are exposed to an external magnetic field, they will be rapidly magnetized (Yang et al., 2019). The magnetic particles and the magnetic field work together to enhance the effectiveness of their bone tissue repair treatment (Yang et al., 2019; Zhao et al., 2019). Varieties of MNPs loaded with/without drugs have been applied in the medical industry, playing a very important role. Especially for bone tissue repair, MNPs have been proven efficient [Singh et al., 2012a; Maleki, 2014 (Eivazzadeh-Keihan et al., 2019 #160(Eivazzadeh-Keihan et al., 2019 #160)]. This article reviews the common synthesis methods, the mechanism and application of magnetic nanomaterials in the field of bone tissue repair.
Preparation of Magnetic Nanomaterials
Preparation methods have been well-developed with immeasurable application value. Various elemental compositions have been used in magnetic nanomaterials, including Fe3O4, Fe, Co, Ni, MgFe2O4, and Co Fe2O4 (Hamidian and Tavakoli, 2016; Chen et al., 2018; Wu et al., 2019; Xue et al., 2019). The most classic and common composition of magnetic nanomaterials is Fe3O4 (Hamidian and Tavakoli, 2016; Yu et al., 2016; Huang et al., 2018). Two main types of magnetic Fe3O4 preparation methods are the dry method and the wet method (Huang et al., 2018). Among them, the wet method is more commonly applied, mainly including the following techniques, the hydrothermal method, solvothermal method, chemical co-precipitation method, ball milling method, sol-gel method, and the atomic layer deposition method. In the synthesis of MNPs, with different preparation conditions, different preparation methods and different catalysts, turnover number (TON), and turnover frequency (TOF) could differ widely (Singh et al., 2012b; Maleki, 2014, 2018). In the preparation of MNPs for bone repair, with the help of different catalysts, the Suzuki reaction and Heck reaction of halogenated benzene can be carried out efficiently. The overall TON and TOF can reach more than 30,000 mol and 50,000 h−1, respectively (Maleki, 2014).
Organic-Based Method
MNPs can be prepared in sol-gel processes in aqueous solutions, such as co-precipitation methods, microwave synthesis, and hydrothermal reactions (Maleki, 2012, 2013); MNPs can also be prepared in organic solution reactions. Organic phase synthesis has been used to prepare MNPs based on Fe, Co, and Ni alloys, oxides, core/shell, and dumbbell structures (Maleki, 2013, 2014, 2018). The size, shape, and composition of MNPs are affected by one or more reaction parameters, such as reactant concentration, solvent polarity, and reaction temperature/time. Here, we focus on the synthesis of monodisperse MNPs (Table 1).
Hydrothermal/Solvothermal Method
The hydrothermal method is a widely used synthetic method of magnetic nanocomposites (Gan and Xu, 2019; Pandi et al., 2019; Saygili, 2019). The reaction is generally carried out in a reactor, which includes many advantages, such as simple operation and high reacting efficiency. The metastable phase and nano-morphology obtained by hydrothermal/solvothermal methods are difficult to obtain in other ways (Pandi et al., 2019). Generally, Fe3O4 is synthesized by the hydrothermal method using FeCl2, FeCl3, and NaOH at high temperatures in a high-pressure reactor. The reaction principle of the solvothermal method and hydrothermal method is similar, with differences in application of the ethylene glycol medium (Liu et al., 2016). The method is suitable for a wider temperature range as the solvent used has a higher boiling point than water (Pandi et al., 2019). With reducing solvents, products could be protected from oxidation during high-temperature preparation. Researchers have successfully prepared magnetic metal oxides, elemental metals, and alloys using hydrothermal/solvothermal methods (Liu et al., 2016; Wang X. et al., 2018; Saygili, 2019).
Co-precipitation Method
The most commonly used preparation method of Fe3O4 is the chemical co-precipitation method (Wan and Li, 2015; Nosrati et al., 2018; Darwish et al., 2019). An alkaline substance is added to the soluble iron salt and ferrous salt solution, creating a precipitate or hydrated precursor (Majidi et al., 2016). Followed by washing, drying, and burning procedures, magnetic nanoparticles would be synthesized. This method is easy to operate and can produce a large number of nanoparticles. The limitation of this method is the poor controllability of its particle size and distribution, because the kinetic factor is the only controllable factor in the grain growth process (Nosrati et al., 2018).
Ball Milling Method
Mechanical grinding is a method of crushing coarse particles (Fe3O4 with a general particle size of 10 mm) through strong plastic deformation to the nanometer level (Zhang et al., 2015; Yang et al., 2020). In the planetary ball mill, the coarse-grained material is refined mainly by the collision between the steel balls or between the steel balls and the inner wall of the grinding tank. Using the dry grinding process, nanoparticles can be prepared, but due to repeat crushing-cold welding, the final result is micron-sized particle aggregates with nanograin structures (Narayanaswamy et al., 2019). To obtain highly dispersed nanoparticles, a wet grinding process is required, which has a better grinding and crushing effect. Compared with metal, ceramic powder is more conducive to ball milling due to its brittleness. The physical ball milling method has a good reproducibility of particle size. With expensive equipment, long production cycle, and low efficiency, it is difficult to achieve industrial production (Amiri et al., 2019; Narayanaswamy et al., 2019).
Sol-Gel Method
The sol-gel method for preparing nano metal oxide is an ideal wet method. This method is based on the hydroxylation and condensation of molecular precursors in solution to form a “sol” of nanoparticles (Kayili and Salih, 2016; Lin et al., 2018a; Wang J. et al., 2018; Bao et al., 2020). Gao, prepared Fe3O4 nanoparticles under supercritical conditions by the sol-gel method (Gao et al., 2019). The results show that the particles have a monodisperse distribution with an average size of nearly 8 nm. Due to the small particle size and good dispersion, the authors suggested that the particles could be used in biomedical magnetotherapy. Lin et al. reported that monodisperse spherical Co-Cr-Ferrite nanomaterials can be easily prepared by co-condensation of Co(NO3)2·6H2O, chromium nitrate, Cr(NO3)3·9H2O, and iron nitrate using the sol-gel method. Co-Cr-Ferrite nanomaterials were prepared with excellent magnetic properties with stable monodisperse distribution (Lin et al., 2018a).
Atomic Layer Deposition Method
Recently, atomic layer deposition (ALD) has become an important thin film deposition technology, which is widely used in thin film deposition on the surface of various semiconductors, metal oxides, and polymers (Sanli et al., 2018; Li et al., 2019b; Ponti et al., 2020). This method has many advantages, including precise control of product thickness and excellent uniformity. It is a good method for synthesizing multi-component structure controllable composite materials. Alessandro Ponti synthesized SiO2-coated α-Fe2O3 nanofibers (NFs) with the ALD method (Ponti et al., 2020). It was used for electromagnetic wave absorption. The results showed that the obtained magnetic carbon nanowires have a uniform morphology, which has lower magnetic reflection loss and a higher frequency absorption range than simple carbon nanowires. This means better electromagnetic wave absorption capacity (Ponti et al., 2020).
Effect of Magnetic Field on Bone Cells
Different magnetic field strengths have different effects on cells, while medium-strength magnetic fields are the most widely used (Mirabello et al., 2016). Recent studies have found that a medium-strength magnetic field can promote the biomineralization process of mouse osteoblasts. The medium-strength magnetic field can promote attachment, proliferation, migration, and the differentiation of cells (Mirabello et al., 2016; Xia et al., 2018). It was confirmed to be related to changes of the calcium ions transport channel on the cell membrane (Rotherham et al., 2018). The magnetic field not only affects the biomineralization behavior in the early stage, but also affects the expression of osteocalcin genes and proteins in the later stage. The production of late stage proteins is proportional to the early stage of biomineralization. A magnetic field affects the structure and crystallinity of biomineralization products and changes the spatial structure of proteins in the cytoskeleton (Figure 1).
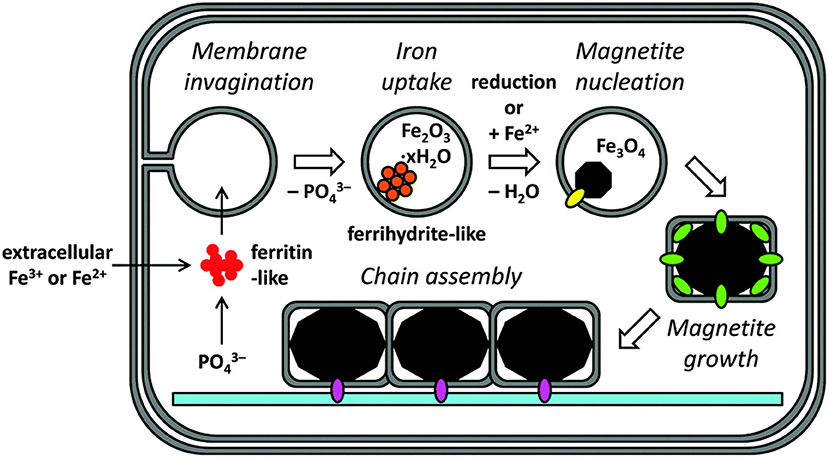
Figure 1. Biomineralizatimodel of magnetite in cellular magnetosomes. Reproduced with permission from Mirabello et al. (2016).
The magnetic field can be divided into static magnetic field and dynamic magnetic field according to whether the direction of the magnetic field changes (Hu et al., 2015; Abreu et al., 2016). The static magnetic field mainly uses permanent magnets and DC power supplies, while the dynamic magnetic field uses an alternating current (Jiang et al., 2016b). The static magnetic field is divided into four levels according to the strength, the weak magnetic field for <1 mT, the medium magnetic field for 1 mT-1T, the strong magnetic field for 1-5T, and the super magnetic field for > 5T (Mazzone et al., 2017; Wang Z. et al., 2017). Zhang et.al. applied 4 mT electric fields to bone cells. Testing the changes of 16 genes under different magnetic fields by RT-PCR, they found that different magnetic field strengths have different effects on different genes (Zhang et al., 2018a). When the magnetic field is strong, it can change the movement state of the cell (Filippi et al., 2019). Filippi conducted an experiment on osteoblast cells with a strong magnetic field (Filippi et al., 2019). It was found that the cells under the external magnetic field are arranged in the direction of the magnetic field, showing orientation. It was not affected by the orientation of the material surface. The influence of the magnetic field on the cell depends on two aspects (Mirabello et al., 2016). One is the type of cell, the other is the strength and duration of the external magnetic field. The action process of the magnetic field on the cell is shown in Figure 2. Firstly, the cell is attached to the surface of the material and placed on the magnet (Mazzone et al., 2017; Zhang et al., 2018a,b). The direction of the magnetic induction line is perpendicular to the cell. Animal experiments have shown that magnetic fields can promote the attachment, proliferation, differentiation, and expression of growth factors such as bone morphology proteins, accelerate bone fusion, and promote the formation of new bone (Wang Z. et al., 2017; Guerri et al., 2018; Filippi et al., 2019). The magnetic field can also promote the fusion of bone and implant and increase bone density and calcium content to accelerate the healing of bone damage.
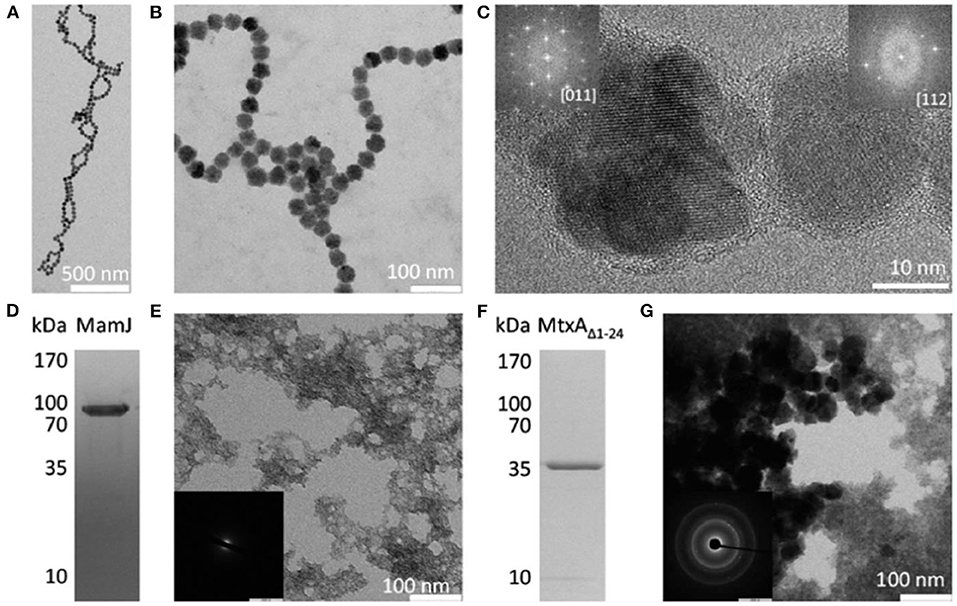
Figure 2. Precipitation products present in polypeptides and proteins showing the reflection with magnetite. (A–C) Magnetite particles formed in the presence of poly 1-arginine. The insert in (C) the FFT of the particles, showing a reflection consistent with magnetite. (D) SDS-PAGE of MamJ. (E) Precipitation product in the presence of MamJ. (E) an electron diffraction pattern with no signs of crystallization. (F) MtxAΔ1−24 SDS-PAGE. (G) The precipitation product in front of MtxAΔ1−24. (G) an electron diffraction pattern showing the reflection consistent with magnetite. Reproduced, with permission, from Mirabello et al. (2016).
Effect of Magnetic Particles on Bone Repair
The magnetic nanoparticles have the ability to bind to the cell surface, which makes it possible to control and regulate the function of cells under the condition of an external magnetic field (Hurle et al., 2018; Fernandes et al., 2019; Parfenov et al., 2020). Qian et al. studied the effect of magnetic nanoparticles on bone marrow mesenchymal stem cells (Qian et al., 2018). The results show that magnetic nanoparticles are accumulated in mesenchymal stem cells at an average concentration of 20 pg per cell, which has no adverse effect on the proliferation and differentiation of bone marrow mesenchymal stem cells (Qian et al., 2018). Under the action of an external magnetic field, magnetic nanoparticles can significantly promote the proliferation of bone marrow mesenchymal stem cells. Dabrowska studied the effects of magnetic nanoparticles and an applied magnetic field on human mesenchymal stem cells (Ahn et al., 2018; Dabrowska et al., 2018). After 21 days of cultivation in vitro with induction medium, human mesenchymal stem cells have the ability to differentiate into osteoblasts, adipocytes, and chondrocytes under the action of magnetic nanoparticles with the magnetic field (Hu et al., 2013; Yang et al., 2018) (Figure 3). Cells cultured in vitro were collected and implanted in the skull bone defect of nude mice (Mortimer and Wright, 2017; Qian et al., 2018). Histological observation confirmed that there was obvious new bone formation 14 days after the cells were implanted, while no new bone formation was found in the control (no implanted cells). It showed that the combined action of magnetic nanoparticles and the magnetic field can promote bone repair (Tang et al., 2017; Yang et al., 2018). Magnetic nanoparticles have been proven to promote bone tissue regeneration mainly through the magnetic force generated by itself and the external magnetic field (Mortimer and Wright, 2017; Lin et al., 2018b; Fernandes et al., 2019). Fernandes et al. studied the effect of an external magnetic field on bone repair. They implanted calcium phosphate-magnetic nanoparticle composites under the back of a rat (Hurle et al., 2018; Qian et al., 2018; Fernandes et al., 2019). Under the condition of an external magnetic field, this composite material can significantly promote the proliferation and differentiation of cells and 30 days after the formation of new bone tissue. This shows that the magnetic field can promote bone repair (Tang et al., 2017; Ahn et al., 2018; Yang et al., 2018).
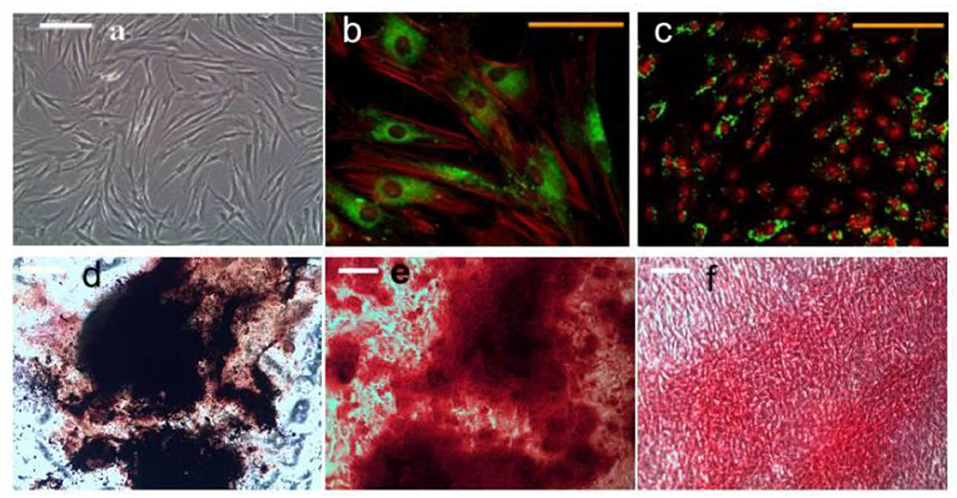
Figure 3. HMSCs treated with magneto mechanical stimulation combined with biochemical factors formed mineralized nodules. Alizarin red and von Kossa co-staining showed positive calcium and phosphorus in the nodules. (a) Undifferentiated hMSCs morphology; (b) Magnetic nanoparticles (MNP) For PDGFRα; (c) integrin ανβ3 hMSCs, (b,d) histological analysis of hMSCs after 21 days of osteogenic induction; (e) alizarin red s staining; (f) Sirius-red staining. Reproduced with permission from Hu et al. (2013).
In order to ensure the full effect, it is necessary to maintain the distribution of nanoparticles among MNPs. The proportion of mismatched atoms on the surface of the material increases as the particle size decreases, while this degree of mismatch is more pronounced at the nanometer scale (Maleki, 2013, 2014). Between nanoparticles, the effects of the chemical bonding force, van der Waals dispersion force, and Coulomb force are very strong. Particles are very easy to reunite. From the perspective of surface energy, most MNPs are hydrophobic with large surface energy (Singh et al., 2012a; Singh and Kim, 2013). In order to minimize the overall surface energy, these nanoparticles are prone to agglomeration, forming large agglomerates, which ultimately leads to an increase in particle size. According to the agglomeration mechanism of MNPs, the dispersion problem of nanometers is mainly to eliminate the problem of hard agglomeration (particles agglomerated in a face-to-face connection) between nanoparticles (Singh et al., 2012a). At present, widely used anti-agglomeration methods include organic solvent washing, freeze drying, anti-agglomeration under ultrasonic action, and surfactant anti-agglomeration. Among them, surfactant which is also known as surface modifier, is the most effective in preventing agglomeration. When MNPs reunite, degrade, or change in structure, their functions and bone repair effects under magnetic fields will change accordingly (Singh and Kim, 2013; Eivazzadeh-Keihan et al., 2019). Compared with the MNPs in good condition, the particles with structural changes mainly show that the responsiveness of the magnetic field stimulus decreases, the activity of bone cells decreases, and the bone repair function decreases after the action (Maleki, 2012; Singh et al., 2012b, 2014).
Magnetic Nanomaterial Bone Repair Application
Magnetic nanomaterials not only have the unique properties of nanoparticle materials, but also have magnetic responsiveness and superparamagnetism (Xia et al., 2018; Fernandes et al., 2019). They can gather and position under a constant magnetic field and absorb electromagnetic waves to generate heat under an alternating magnetic field (Li et al., 2016). Among them, magnetic iron oxide nanoparticles are widely used in magnetic stimulation to promote bone formation, drug loading, bone formation with stem cells, and bone formation with scaffolds (Yun et al., 2016a; Rotherham et al., 2018; Xia et al., 2018, 2019a; Zhao et al., 2019). Magnetic nanomaterials have shown good bone-promoting effects in many studies and have good application prospects (Lu et al., 2018; Fernandes et al., 2019).
Magnetic Nanoparticles Combined With Magnetic Field Stimulation
Bigham et al. grafted a magnetic Mg2SiO4-CoFe2O4 composite scaffold on the surface of warp-based apatite particles. Compared with the traditional HA particles, FeHA improves the survival rate of osteoblasts under the effect of an external magnetic field and affects the cell morphology (Bigham et al., 2019). Parfenov et al. used magnetic particles and apatite as bone tissue engineering scaffolds. Under the action of an external magnetic field, osteoblasts will show a positive effect on the proliferation and differentiation. Both the magnetic particles and the applied magnetic field have a synergistic effect on the cells (Parfenov et al., 2020) (Figure 4).
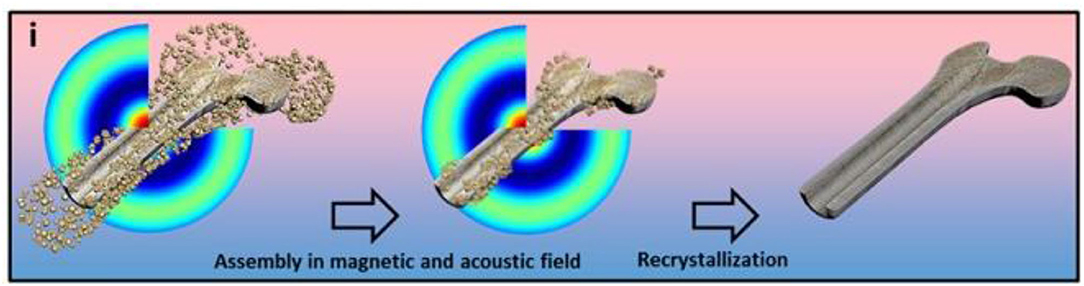
Figure 4. The schematic representation of the fabrication of a three-dimensional scaffold using a magnetoacoustics' field, including the assembly and recrystallization of the scaffold. Fabrication of calcium phosphate 3D scaffolds for bone repair using magnetic stimulation. Reproduced with permission from (Parfenov et al., 2020).
One disadvantage of magnetic particles is their easy agglomeration, which limits their application in the field of biomedical medicine (Luo et al., 2018; Rotherham et al., 2018). By using chemical co-precipitation method, MNPs were evenly dispersed into PVA nanofibers, and then made into a film by in-situ generation and an electrospinning method to obtain PVA nanofiber membranes containing MNPs with good dispersion (Manjua et al., 2019). Parfenov et al. grew osteoblasts on the surface of polystyrene, and applied a static magnetic field of 150 mT, which can promote the proliferation and differentiation of osteoblasts (Parfenov et al., 2020). Fernandes discovered that magnetic fields can promote gene expression in mouse neural stem cells (Fernandes et al., 2019). Rotherham found in their research that the magnetic field not only affects the synthesis of DNA and RNA of cells, but also affects the movement state and morphology of cells when the magnetic field strength is > 10T (Rotherham et al., 2018). Díaz prepared PLLA nanofiber membrane by electrospinning. When conducting in vitro cell culture experiments, it was found that the orientation of the nanofiber membrane itself had a contact guiding effect on osteoblasts (Díaz et al., 2019).
Magnetic Nanoparticle Drug Loading
“Drug targeted therapy” is a way to make drugs selectively enriched in the pathological site for treatment (Kumari et al., 2016; Shen et al., 2016; Jung et al., 2018). Compared with the traditional method of administration, it can reduce the dose of drugs, reduce the possible side effects of the drugs on healthy tissues, and greatly reduce the cost of drug treatment (Kumari et al., 2016; Qi et al., 2016; Sun et al., 2019). The structure of the magnetic microsphere loaded with release growth factors is shown in Figure 5, in which the magnet is served by nano-magnetic particles (Li et al., 2018).
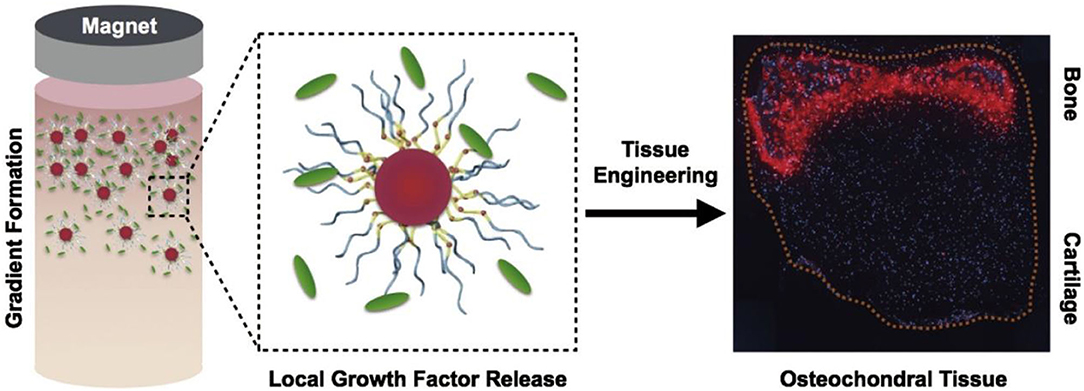
Figure 5. The use of magnetically aligned glycosylated cations to produce osteochondral tissue. The coupling of anions with heparin produces a glycosylated canopy that can effectively isolate and release growth factors, the key mineralized protein osteopontin (Iwasa & Reddi), which is specifically present at the bone end of tissues. Reproduced with permission from Li et al. (2018).
Antibiotics, growth factors, and microRNA and siRNA related to osteogenesis can improve the repair effect of bone defects (Jia et al., 2018; Li et al., 2018; Ferjaoui et al., 2019). However, these drugs cannot target specific sites or produce a direct repair effect. In recent years, people have been working on finding the best drug delivery system to reduce the adverse effects and toxicity of drugs, reduce the loss of drug efficacy, and improve the therapeutic effect (Jung et al., 2018; Lugert et al., 2019; Sun et al., 2019; Vangijzegem et al., 2019). Studies found that iron oxide nanoparticles can be used as a means of delivery, which can effectively carry drug molecules under the action of an external magnetic field and target specific parts of the body (Denyer et al., 2018). Suspension of drug molecules on magnetic carriers or dispersion on magnetic nanoparticles is a simple and direct magnetic targeted drug delivery route (Denyer et al., 2018). Recent studies have used solvent evaporation and lyophilization to prepare degradable polylactic acid-glycolic acid copolymer capsaicin-coated magnetic nanoparticles (Xia et al., 2018). This magnetic nanoparticle provides the continuous release of capsaicin. Under the action of an external magnetic field, the magnetic nanoparticles can reach specific locations and improve the therapeutic effect on specific locations (Xia et al., 2018). In addition to magnetic targeting, magnetic heating is also used to control the release of therapeutic drugs from thermally responsive drug carriers. Xia et al. coated iron oxide nanoparticles with bisphosphonate and dextran to obtain bisphosphonate/dextran/Fe3O4 nanoparticles, which were thermally decomposed using a radio frequency system. Devouring has a strong destructive effect (Xia et al., 2019a). In the other study, magnetic multi-walled carbon nanotubes, hydroxyapatite, and clodronate were synthesized into nanocomposites. It was found that clodronate can be continuously released from the system and inhibit the formation of osteoclasts (Swietek et al., 2019). The magnetic liposomes are obtained by embedding iron oxide nanoparticles into the liposome membrane. When an alternating magnetic field is applied externally, the generated heat will destroy the cell membrane, releasing the drug encapsulated in the liposome for therapeutic purposes. In addition to promoting osteogenic differentiation, iron oxide nanoparticles also have good bone conduction ability (Xia et al., 2018; Swietek et al., 2019). Currently in the field of bone regenerative medicine, iron oxide nanoparticle complexes are often used as carriers for the controlled release of drugs. Loading gentamicin in multifunctional magnetic mesoporous bioactive glass has been studied. The Fe3O4 nanoparticles in multifunctional magnetic mesoporous bioactive glass improved the continuous release of gentamicin, which is helpful to reduce the adhesion of bacteria and prevent biofilm formation (Wang et al., 2015). In addition to treating infection, the Fe3O4 nanoparticles in the composite can also promote the adhesion, proliferation, and osteogenic differentiation of bone marrow mesenchymal stem cells (Xia et al., 2018; Swietek et al., 2019). Nanomaterials have been used as carriers to deliver therapeutic drugs in cells, including proteins, growth factors, small molecule chemicals, and DNA/RNA (Cruz-Acuña et al., 2018; Kuai et al., 2018; Xia et al., 2018, 2019a). This magnetic drug delivery system will provide a useful support platform for bone defect repair.
Magnetic Nanoparticles With Stem Cells
In recent years, stem cell therapy has been proffered as a strategy for repairing bone defects, especially in repairing large area bone defects (Xia et al., 2018; Li et al., 2019a). At present, although stem cell transplantation has been successful in treating animal bone defect models, these traditional stem cell transplantations have not achieved similar results in clinical treatment (Xia et al., 2018). How to maintain long-term and effective stem cell characteristics is the key to successful treatment. Therefore, it is necessary for bone expansion to maintain a stable cell phenotype and reduce cell necrosis at the defect site after transplantation in vivo (Liu et al., 2018; Nejadnik et al., 2019). With the development of materials science and chemical biology, researchers have tried to use iron oxide nanoparticles as a tool for the research and control of stem cells for many years (Li et al., 2019a). Iron oxide nanoparticles can be combined with an external magnetic field to affect cell adhesion, proliferation, movement and distribution, and stem cell osteogenic differentiation. In addition, iron oxide nanoparticles can be used to label cells for in vivo tracking and monitoring (Son et al., 2015; Nejadnik et al., 2019). Liu et al. found the mechanism of Fe3O4/BSA particles uptake into stem cells, promoting the osteogenesis under the action of an external magnetic field (Liu et al., 2016) (Figure 6).
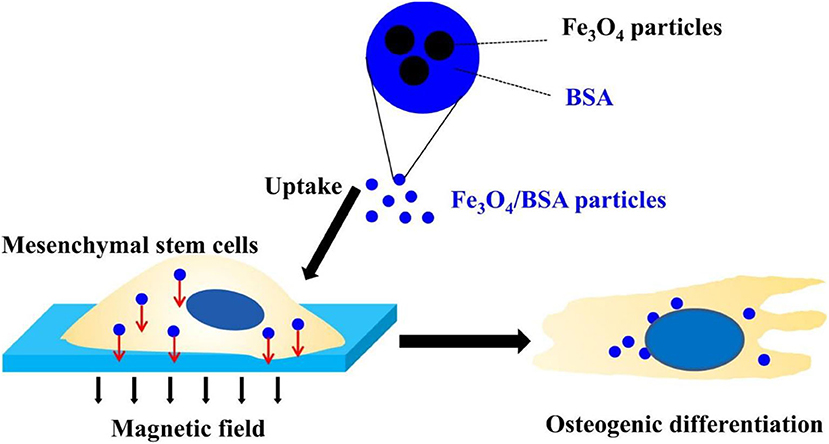
Figure 6. Fe3O4/BSA particles significantly promote the osteogenesis of mesenchymal stem cells under the action of an external magnetic field, while magnetic particles and an external magnetic field alone do not affect the differentiation of stem cells. Reproduced with permission from Liu et al. (2016).
One of the applications of iron oxide nanoparticles in stem cell therapy is to magnetically target stem cells to a suitable location (Khan et al., 2018; da Silva et al., 2019; Jia et al., 2019). In some studies, an in vitro magnetic targeting system was developed to attract rabbit bone marrow mesenchymal stem cells. It was found that this technology significantly promoted cells penetration and bone formation labeled with iron oxide nanoparticles into porous hydroxyapatite ceramics transplanted into rabbit ulnar defects (Li et al., 2019a). Similarly, Khan et al. also reported that a magnetite cationic liposome (MCLS) with Fe3O4 nanoparticles as the core and columnar neodymium magnets were used to expand mesenchymal stem cells (Khan et al., 2018). After culturing, the density and number of mesenchymal stem cells were significantly higher than those of conventional cultured cells. It revealed that iron oxide nanoparticles can attract stem cells to homing. They can also promote the differentiation of mesenchymal stem cells in vitro and in vivo (Khan et al., 2018). Son et al. used magnetically labeled mesenchymal stem cells to study a magnetic targeting system for repairing severe chronic bone defects using external magnetic devices (Son et al., 2015). It was found that transplanting with enough stem cells can completely repair severe chronic bone defects. In addition to the strategy of promoting bone regeneration by controlling stem cells, iron oxide nanoparticles themselves, especially under the stimulation of an external magnetic field, can induce the osteogenic differentiation of stem cells (Son et al., 2015). Jia et al. prepared polydextrose sorbitol carboxymethyl ether coated iron oxide nanoparticles and studied their effects on bone marrow mesenchymal stem cells (Jia et al., 2019). The results showed that iron oxide nanoparticles were structurally stable in bone marrow mesenchymal stem cells promoting the osteogenic differentiation of bone marrow mesenchymal stem cells. Gene microarray analysis and bioinformatics analysis showed that iron oxide nanoparticles can activate the classic mitogen-activated proline kinase (MAPK) signaling pathway (Sweeney et al., 2018; Xia et al., 2018). Under a static magnetic field, Fe3O4 particles can significantly promote the expression of alkaline phosphatase, type I collagen and osteocalcin at the mRNA, and protein levels in mesenchymal stem cells (Liu et al., 2018; Xia et al., 2018). The magnetic effect of the nanoparticles with mesenchymal stem cells have preliminarily demonstrated that iron oxide nanoparticles have great potential in bone regeneration and repair.
Magnetic Nanoparticles and Tissue Engineering Scaffolds
Tissue engineering is a method of rebuilding functional tissue at the damaged site, usually including seed cells, growth factors, and three-dimensional biodegradable scaffolds (Cruz-Acuña et al., 2018; Kuai et al., 2018; Luo et al., 2018; Cojocaru et al., 2019; Li et al., 2020). The construction of a tissue engineering complex is of great significance to promote tissue regeneration or healing. The current problem is that cells usually stay on the surface of the material and cannot enter the scaffold. Recent studies have found that cells can be driven to the center of the three-dimensional scaffold with the help of magneto-mechanical drive. Parfenov et al. reported a technique of using magnetic force to seed cells, using a porcine acellular common carotid artery stent (Eivazzadeh-Keihan et al., 2020; Parfenov et al., 2020). The porcine acellular common carotid artery stent was immersed in a suspension of magnetically labeled cells. Attached to the porcine acellular common carotid artery stent, it was found with the absorption of iron oxide nanoparticles, the cell adhesion gradually increased. In addition, some studies have found that iron oxide nanoparticles coated with chitosan can enhance the depth of human osteoblasts into the 3D scaffolds. It can take advantage of the magnetic force to increase the interaction between cells and shorten the cell proliferation cycle (Eivazzadeh-Keihan et al., 2019; Parfenov et al., 2020). The application of this magnetic technology has important value in promoting bone tissue repair and regeneration. It could also provide an important theoretical basis for researchers and clinicians (Mahdavinia et al., 2018; Manjua et al., 2019; Parfenov et al., 2020). In recent years, based on the application of magnetic tissue engineering, multi-layer cell membranes have been developed, which will greatly promote the repair of bone tissue. In addition, some studies have found that magnetic tissue engineering can not only induce the formation of multifunctional stem cell membranes, but also promote the formation of repairing blood vessels [(Kim et al., 2014) (Singh et al., 2014#170 (Singh et al., 2014#170; Yun et al., 2016b)]. The application of magnetic tissue engineering can provide a new method for bone tissue engineering. Chen et al. studied the mechanism of ion assembly electrospinning scaffold on cells (Chen et al., 2018) (Figure 7). They found that magnetization promotes osteogenic differentiation of stem cells due to the local magnetic field.
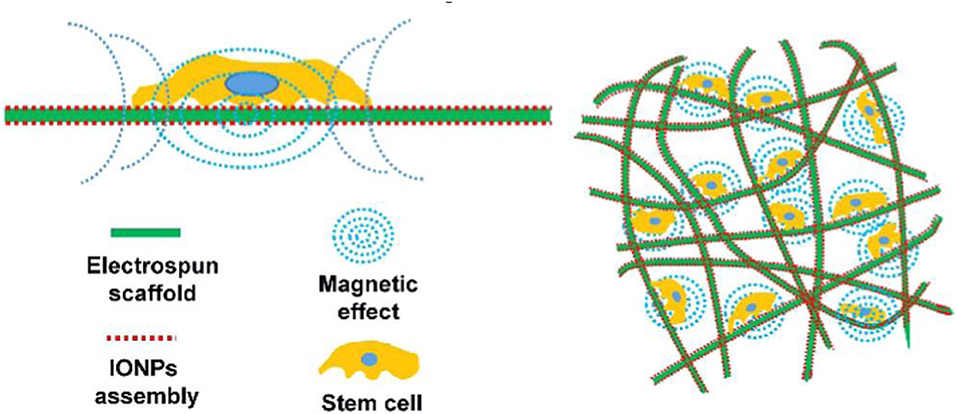
Figure 7. The mechanism of ion assembly electrospinning scaffold on cells is shown. The key lies in the local magnetic field. Magnetization promotes osteogenic differentiation of stem cells. Reproduced with permission from Chen et al. (2018).
The magnetic scaffold can attract growth factors and stem cell migration in the body through magnetic drive, promoting bone repair and regeneration (Díaz et al., 2018; Xia et al., 2018). At present, the role of magnetic scaffolds in promoting cell proliferation and new bone tissue growth has been confirmed (Lu et al., 2018). The scaffold has a wide range of components, mainly including biological macromolecules, synthetic polymers, polyethylene glycol, and inorganic materials (Dashnyam et al., 2014; Cruz-Acuña et al., 2018; Kuai et al., 2018; Luo et al., 2018; Cojocaru et al., 2019; Liu Z. Y. et al., 2020).
It was found that magnetic hydroxyapatite scaffolds loaded with superparamagnetic iron oxide nanoparticles (SPIONs) hydroxyapatite can significantly improve the adhesion and proliferation of bone cells (Yun et al., 2016b; Díaz et al., 2018; Xia et al., 2018). In addition, some studies have found that under the action of a static magnetic field, the magnetic composite scaffold material can obviously promote the proliferation, differentiation, and extracellular matrix secretion of MC3T3-E1 cells (Díaz et al., 2018; Luo et al., 2018). Similarly, Fernandes et al. prepared magnetic nanocomposite scaffolds of iron oxide nanoparticles and polycaprolactone (Fernandes et al., 2019). They found that compared with pure polycaprolactone scaffolds, the magnetic nanocomposite scaffolds had significant advantages in cell adhesion, alkaline phosphatase activity, and expression of genes related to osteogenesis (Fernandes et al., 2019). In addition, the iron oxide nanoparticle nanofiber scaffold can promote bone regeneration of radial segmental defects. Gao et al. applied nano-deformation of IO-OA/PLGA nanocomposites under SMF (Gao et al., 2019). They found that the magnetic mechanical stimulation led to the enhanced osteogenic differentiation of MC3T3-E1 cells (Gao et al., 2019) (Figure 8).
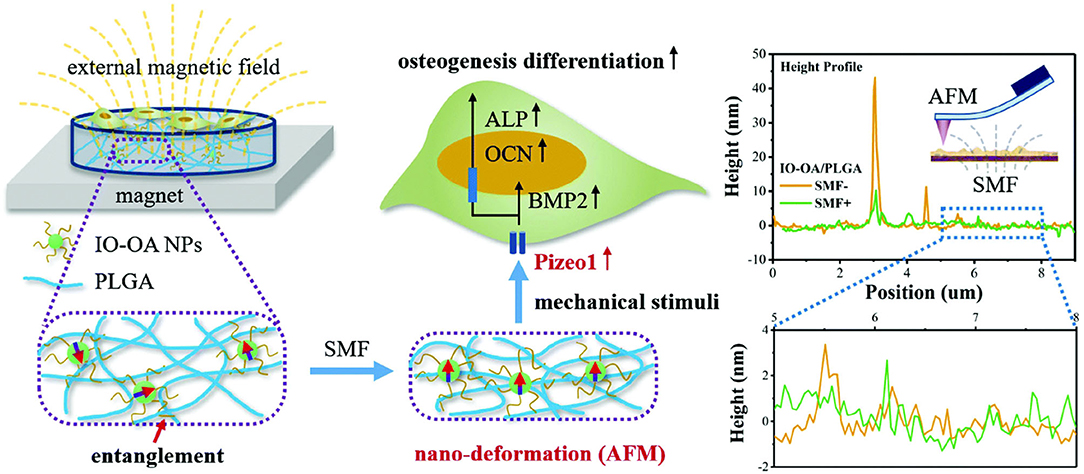
Figure 8. Schematic diagram shows that the magnetic mechanical stimulation caused by the nano-deformation of IO-OA/PLGA nanocomposites under SMF leads to the enhanced osteogenic differentiation of MC3T3-E1 cells. Reproduced with permission from Gao et al. (2019).
The potential mechanism of iron oxide nanoparticles to enhance bone formation in vivo and in vivo is still being explored (Yun et al., 2016a; Luo et al., 2018; Xia et al., 2019b). Xia et al. found in vivo experiments that compared with the pure hydroxyapatite scaffold, the concentration of calcium ions and G protein-coupled receptors on the magnetic hydroxyapatite scaffold increased (Xia et al., 2019b). It was found that functional protein enriched on the magnetic scaffolds can effectively enhanced the osteogenic activities of stem cells through WNT/β-catenin signaling, resulting in increased proliferation of MC3T3-E1 cells and accelerated osteogenic differentiation. Cojocaru et al. also studied the combined effect of Calcium phosphates composites with inclusions of magnetic nanoparticles for bone tissue engineering. They found that a static magnetic field and magnetic scaffold can promote osteogenic differentiation of mouse skull osteoblasts and enhance bone related genes expression and alkaline phosphatase activity (Cojocaru et al., 2019). Although more and more experimental data confirmed that iron oxide nanoparticles have obvious effects on the survival and differentiation of bone cells, especially under the external magnetic field. The interaction with bone cells and magnetic mechanical stimulation still needs further study. Bigham applied a Mg2SiO4-CoFe2O4 nanocomposite scaffold as a multifunctional magnetic scaffold to eradicate remaining bone cancerous tissues after surgery (Bigham et al., 2019) (Figure 9). It is a promising attempt for bone tissue repair and defect regeneration with magnetic nanomaterials along with a 3D scaffold. It is deemed to potentially help solve clinical bone repair problems in the future.
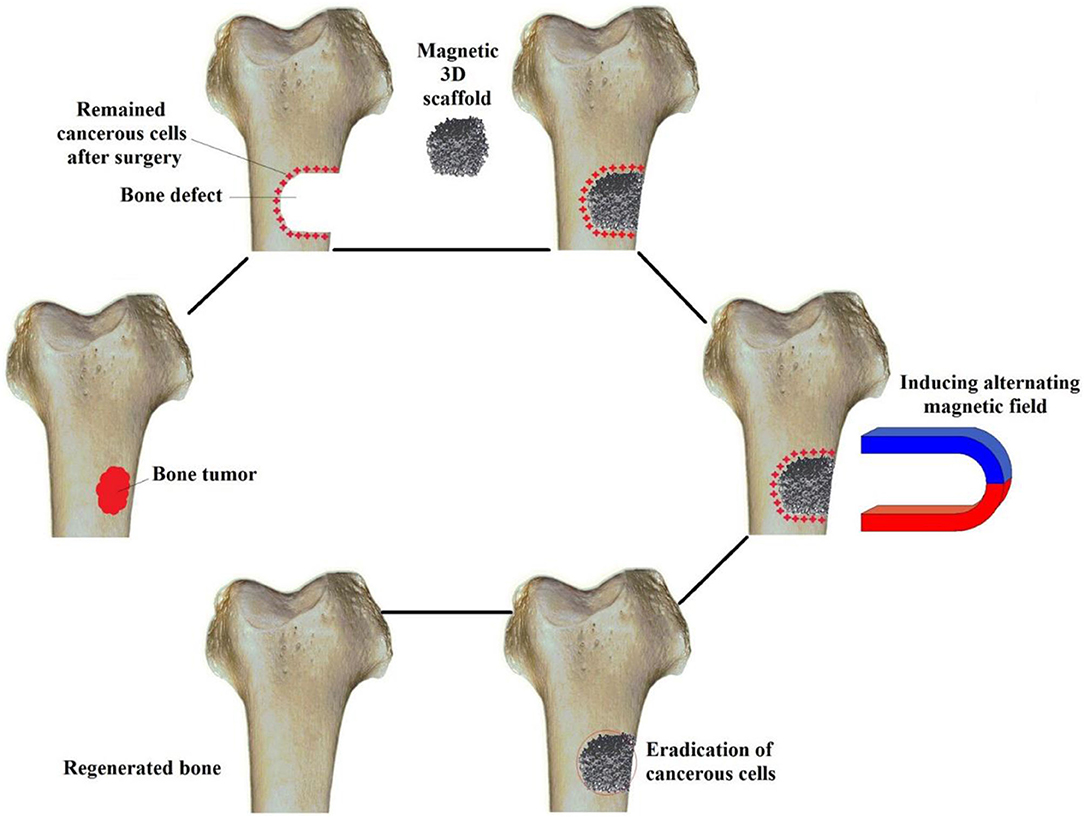
Figure 9. Schematic for applying a Mg2SiO4-CoFe2O4 nanocomposite scaffold as a multifunctional magnetic scaffold to eradicate remaining bone cancerous tissues after surgery and regenerate the defect. Reproduced with permission from Bigham et al. (2019).
Conclusion
This paper reviews advances on the application of magnetic nanomaterials in osteogenesis repair. The application of magnetic nanomaterials in bone regeneration has opened up a new way to repair bone defects. The unique properties of magnetic nanomaterials have accelerated their application in medicine, especially in terms of magnetism, magnetic fields can provide remote control of drug release and biomolecule activation, generating biological reactions including cell differentiation, tissue growth, and bone defect regeneration. The development of a magnetic field suitable for a bone repair application will facilitate the safe, convenient, and effective control of magnetic nanomaterials. Although the application of magnetic nanomaterials in bone regeneration has achieved initial success, the interaction mechanism between magnetic nanomaterials, magnetic fields, and osteoblasts is not yet clear, and the mechanism and principle of action need to be clarified in the future.
Author Contributions
DaF, QW, and TZ contributed equally to this reviewed paper. XL, DoF, and XW conceived and designed the content of the paper. DaF, QW, and BL collected the researched literatures, arranged the outline of collected documents, and wrote the articles. HW, BL, YW, and ZL made important suggestions and helped revising the paper. All authors reviewed and commented on the entire manuscript.
Funding
This research was funded by the Ministry of Science and Technology of China (2016YFB1101501), National Natural Science Foundation of China (51973226) and Key scientific research projects of higher education institutions in Henan Province (20A320069).
Conflict of Interest
The authors declare that the research was conducted in the absence of any commercial or financial relationships that could be construed as a potential conflict of interest.
References
Abou Neel, E. A., Aljabo, A., Strange, A., Ibrahim, S., Coathup, M., Young, A. M., et al. (2016). Demineralization-remineralization dynamics in teeth and bone. Int. J. Nanomed. 11, 4743–4763. doi: 10.2147/ijn.S107624
Abreu, M. C., Ponzoni, D., Langie, R., Artuzi, F. E., and Puricelli, E. (2016). Effects of a buried magnetic field on cranial bone reconstruction in rats. J. Appl. Oral Sci. 24, 162–170. doi: 10.1590/1678-775720150336
Ahn, S. Y., Chang, Y. S., Sung, S. I., and Park, W. S. (2018). Mesenchymal stem cells for severe intraventricular hemorrhage in preterm infants: phase I dose-escalation clinical trial. Stem Cells Transl. Med. 7, 847–856. doi: 10.1002/sctm.17-0219
Aliramaji, S., Zamanian, A., and Mozafari, M. (2017). Super-paramagnetic responsive silk fibroin/chitosan/magnetite scaffolds with tunable pore structures for bone tissue engineering applications. Mater. Sci. Eng. C Mater. Biol. Appl. 70, 736–744. doi: 10.1016/j.msec.2016.09.039
Amiri, M., Eskandari, K., and Salavati-Niasari, M. (2019). Magnetically retrievable ferrite nanoparticles in the catalysis application. Adv. Colloid Interface Sci. 271:101982. doi: 10.1016/j.cis.2019.07.003
Bao, W. R., Li, M. L., Yang, Y. Y., Wan, Y., Wang, X., Bi, N., et al. (2020). Advancements and frontiers in high performance of natural hydrogels for cartilage tissue engineering. Front. Chem. 8:53. doi: 10.3389/fchem.2020.00053
Bigham, A., Aghajanian, A. H., Behzadzadeh, S., Sokhani, Z., Shojaei, S., Kaviani, Y., et al. (2019). Nanostructured magnetic Mg(2)SiO(4)-CoFe(2)O(4) composite scaffold with multiple capabilities for bone tissue regeneration. Mater. Sci. Eng. C Mater. Biol. Appl. 99, 83–95. doi: 10.1016/j.msec.2019.01.096
Bodenstein, T., and Eichhofer, A. (2019). Magnetic anisotropy in trigonal planar Fe(ii) bis(trimethylsilyl)amido complexes of the type [Fe{N(SiMe3)2}2L]-experiment and theory. Dalton Trans. 48, 15699–15712. doi: 10.1039/c9dt01702j
Brett, E., Zielins, E. R., Luan, A., Ooi, C. C., Shailendra, S., Atashroo, D., et al. (2017). Magnetic nanoparticle-based upregulation of B-cell lymphoma 2 enhances bone regeneration. Stem Cells Transl. Med. 6, 151–160. doi: 10.5966/sctm.2016-0051
Carlotto, S., Finetti, P., de Simone, M., Coreno, M., Casella, G., Sambi, M., et al. (2019). Comparative experimental and theoretical study of the Fe L2,3-edges X-ray absorption spectroscopy in three highly popular, low-spin organoiron complexes: [Fe(CO)5], [(eta(5)-C5H5)Fe(CO)(mu-CO)]2, and [(eta(5)-C5H5)2Fe]. Inorg. Chem. 58, 5844–5857. doi: 10.1021/acs.inorgchem.9b00226
Chen, Q., Liu, D., Wu, C., Yao, K., Li, Z., Shi, N., et al. (2018). Co-immobilization of cellulase and lysozyme on amino-functionalized magnetic nanoparticles: an activity-tunable biocatalyst for extraction of lipids from microalgae. Bioresour. Technol. 263, 317–324. doi: 10.1016/j.biortech.2018.04.071
Cojocaru, F. D., Balan, V., Popa, M. I., Lobiuc, A., Antoniac, A., Antoniac, I. V., et al. (2019). Biopolymers - Calcium phosphates composites with inclusions of magnetic nanoparticles for bone tissue engineering. Int. J. Biol. Macromol. 125, 612–620. doi: 10.1016/j.ijbiomac.2018.12.083
Cruz-Acuña, M., Halman, J. R., Afonin, K. A., Dobson, J., and Rinaldi, C. (2018). Magnetic nanoparticles loaded with functional RNA nanoparticles. Nanoscale 10, 17761–17770. doi: 10.1039/c8nr04254c
da Silva, H. R., Mamani, J. B., Nucci, M. P., Nucci, L. P., Kondo, A. T., Fantacini, D. M. C., et al. (2019). Triple-modal imaging of stem-cells labeled with multimodal nanoparticles, applied in a stroke model. World J. Stem Cells 11, 100–123. doi: 10.4252/wjsc.v11.i2.100
Dabrowska, S., Del Fattore, A., Karnas, E., Frontczak-Baniewicz, M., Kozlowska, H., Muraca, M., et al. (2018). Imaging of extracellular vesicles derived from human bone marrow mesenchymal stem cells using fluorescent and magnetic labels. Int. J. Nanomed. 13, 1653–1664. doi: 10.2147/ijn.S159404
Darwish, M. S. A., Kim, H., Lee, H., Ryu, C., Lee, J. Y., and Yoon, J. (2019). Synthesis of magnetic ferrite nanoparticles with high hyperthermia performance via a controlled co-precipitation method. Nanomaterials 9:1176. doi: 10.3390/nano9081176
Dashnyam, K., Perez, R. A., Singh, R. K., Lee, E. J., and Kim, H. W. J. R. A. (2014). Hybrid magnetic scaffolds of gelatin–siloxane incorporated with magnetite nanoparticles effective for bone tissue engineering. RSC Adv. 4, 40841–40851. doi: 10.1039/C4RA06621A
Debnath, S., Yallowitz, A. R., McCormick, J., Lalani, S., Zhang, T., Xu, R., et al. (2018). Discovery of a periosteal stem cell mediating intramembranous bone formation. Nature 562, 133–139. doi: 10.1038/s41586-018-0554-8
Denyer, S., Bhimani, A. D., Papastefan, S., Kheirkhah, P., Aguilar, T., Zakrzewski, J., et al. (2018). Magnetic kyphoplasty: a novel drug delivery system for the spinal column. PLoS ONE 13:e0201402. doi: 10.1371/journal.pone.0201402
Díaz, E., Valle, M. B., Ribeiro, S., Lanceros-Mendez, S., and Barandiarán, J. M. (2018). Development of magnetically active scaffolds for bone regeneration. Nanomaterials 8:678. doi: 10.3390/nano8090678
Díaz, E., Valle, M. B., Ribeiro, S., Lanceros-Mendez, S., and Barandiarán, J. M. (2019). A new approach for the fabrication of cytocompatible PLLA-magnetite nanoparticle composite scaffolds. Int. J. Mol. Sci. 20:4664. doi: 10.3390/ijms20194664
Eivazzadeh-Keihan, R., Chenab, K. K., Taheri-Ledari, R., Mosafer, J., Hashemi, S. M., Mokhtarzadeh, A., et al. (2020). Recent advances in the application of mesoporous silica-based nanomaterials for bone tissue engineering. Mater. Sci. Eng. C Mater. Biol. Appl. 107:110267. doi: 10.1016/j.msec.2019.110267
Eivazzadeh-Keihan, R., Maleki, A., de la Guardia, M., Bani, M. S., Chenab, K. K., Pashazadeh-Panahi, P., et al. (2019). Carbon based nanomaterials for tissue engineering of bone: building new bone on small black scaffolds: a review. J. Adv. Res. 18, 185–201. doi: 10.1016/j.jare.2019.03.011
Ferjaoui, Z., Jamal Al Dine, E., Kulmukhamedova, A., Bezdetnaya, L., Soon Chang, C., Schneider, R., et al. (2019). Doxorubicin-loaded thermoresponsive superparamagnetic nanocarriers for controlled drug delivery and magnetic hyperthermia applications. ACS Appl. Mater. Interfaces 11, 30610–30620. doi: 10.1021/acsami.9b10444
Fernandes, M. M., Correia, D. M., Ribeiro, C., Castro, N., Correia, V., and Lanceros-Mendez, S. (2019). Bioinspired three-dimensional magnetoactive scaffolds for bone tissue engineering. ACS Appl. Mater. Interfaces 11, 45265–45275. doi: 10.1021/acsami.9b14001
Filippi, M., Dasen, B., Guerrero, J., Garello, F., Isu, G., Born, G., et al. (2019). Magnetic nanocomposite hydrogels and static magnetic field stimulate the osteoblastic and vasculogenic profile of adipose-derived cells. Biomaterials 223:119468. doi: 10.1016/j.biomaterials.2019.119468
Gan, H., and Xu, H. (2019). A novel aptamer-based online magnetic solid phase extraction method for simultaneous determination of urinary 8-hydroxy-2'-deoxyguanosine and monohydroxylated polycyclic aromatic hydrocarbons. Talanta 201, 271–279. doi: 10.1016/j.talanta.2019.04.004
Gao, L., Tang, Y., Wang, C., Yao, L., Zhang, J., Gao, R., et al. (2019). Highly-efficient amphiphilic magnetic nanocomposites based on a simple sol-gel modification for adsorption of phthalate esters. J. Colloid Interface Sci. 552, 142–152. doi: 10.1016/j.jcis.2019.05.031
Guerri, S., Mercatelli, D., Aparisi Gómez, M. P., Napoli, A., Battista, G., Guglielmi, G., et al. (2018). Quantitative imaging techniques for the assessment of osteoporosis and sarcopenia. Quant. Imaging Med. Surg. 8, 60–85. doi: 10.21037/qims.2018.01.05
Hamidian, H., and Tavakoli, T. (2016). Preparation of a new Fe3O4/starch-g-polyester nanocomposite hydrogel and a study on swelling and drug delivery properties. Carbohydr. Polym. 144, 140–148. doi: 10.1016/j.carbpol.2016.02.048
He, Y., Yu, L., Liu, J., Li, Y., Wu, Y., Huang, Z., et al. (2019). Enhanced osteogenic differentiation of human bone-derived mesenchymal stem cells in 3-dimensional printed porous titanium scaffolds by static magnetic field through up-regulating Smad4. Faseb J 33, 6069–6081. doi: 10.1096/fj.201802195R
Hollingsworth, R. L., Beattie, J. W., Grass, A., Martin, P. D., Groysman, S., and Lord, R. L. (2018). Reactions of dicobalt octacarbonyl with dinucleating and mononucleating bis(imino)pyridine ligands. Dalton Trans. 47, 15353–15363. doi: 10.1039/c8dt03405b
Hu, B., El Haj, A. J., and Dobson, J. (2013). Receptor-targeted, magneto-mechanical stimulation of osteogenic differentiation of human bone marrow-derived mesenchymal stem cells. Int. J. Mol. Sci. 14, 19276–19293. doi: 10.3390/ijms140919276
Hu, J., Zhang, T., Xu, D., Qu, J., Qin, L., Zhou, J., et al. (2015). Combined magnetic fields accelerate bone-tendon junction injury healing through osteogenesis. Scand. J. Med. Sci. Sports 25, 398–405. doi: 10.1111/sms.12251
Huang, J., Liu, W., Liang, Y., Li, L., Duan, L., Chen, J., et al. (2018). Preparation and biocompatibility of diphasic magnetic nanocomposite scaffold. Mater. Sci. Eng. C Mater. Biol. Appl. 87, 70–77. doi: 10.1016/j.msec.2018.02.003
Hurle, K., Weichhold, J., Brueckner, M., Gbureck, U., Brueckner, T., and Goetz-Neunhoeffer, F. (2018). Hydration mechanism of a calcium phosphate cement modified with phytic acid. Acta Biomater. 80, 378–389. doi: 10.1016/j.actbio.2018.09.002
Iwasa, K., and Reddi, A. H. (2018). Pulsed Electromagnetic fields and tissue engineering of the joints. Tissue Eng. Part B Rev. 24, 144–154. doi: 10.1089/ten.TEB.2017.0294
Jia, G., Han, Y., An, Y., Ding, Y., He, C., Wang, X., et al. (2018). NRP-1 targeted and cargo-loaded exosomes facilitate simultaneous imaging and therapy of glioma in vitro and in vivo. Biomaterials 178, 302–316. doi: 10.1016/j.biomaterials.2018.06.029
Jia, Y., Zhang, P., Sun, Y., Kang, Q., Xu, J., Zhang, C., et al. (2019). Regeneration of large bone defects using mesoporous silica coated magnetic nanoparticles during distraction osteogenesis. Nanomedicine 21:102040. doi: 10.1016/j.nano.2019.102040
Jiang, B., Hom, W. L., Chen, X., Yu, P., Pavelka, L. C., Kisslinger, K., et al. (2016a). Magnetic hydrogels from alkyne/cobalt carbonyl-functionalized ABA triblock copolymers. J. Am. Chem. Soc. 138, 4616–4625. doi: 10.1021/jacs.6b01271
Jiang, P., Zhang, Y., Zhu, C., Zhang, W., Mao, Z., and Gao, C. (2016b). Fe(3)O(4)/BSA particles induce osteogenic differentiation of mesenchymal stem cells under static magnetic field. Acta Biomater. 46, 141–150. doi: 10.1016/j.actbio.2016.09.020
Jung, K. O., Jo, H., Yu, J. H., Gambhir, S. S., and Pratx, G. (2018). Development and MPI tracking of novel hypoxia-targeted theranostic exosomes. Biomaterials 177, 139–148. doi: 10.1016/j.biomaterials.2018.05.048
Kayili, H. M., and Salih, B. (2016). Fast and efficient proteolysis by reusable pepsin-encapsulated magnetic sol-gel material for mass spectrometry-based proteomics applications. Talanta 155, 78–86. doi: 10.1016/j.talanta.2016.04.014
Khan, M. R., Dudhia, J., David, F. H., De Godoy, R., Mehra, V., Hughes, G., et al. (2018). Bone marrow mesenchymal stem cells do not enhance intra-synovial tendon healing despite engraftment and homing to niches within the synovium. Stem Cell Res. Ther. 9:169. doi: 10.1186/s13287-018-0900-7
Kim, J.-J., Singh, R. K., Seo, S.-J., Kim, T.-H., Kim, J.-H., Lee, E.-J., et al. (2014). Magnetic scaffolds of polycaprolactone with functionalized magnetite nanoparticles: physicochemical, mechanical, and biological properties effective for bone regeneration. Rsc Adv. 4, 17325–17336. doi: 10.1039/C4RA00040D
Kuai, J. H., Wang, Q., Zhang, A. J., Zhang, J. Y., Chen, Z. F., Wu, K. K., et al. (2018). Epidermal growth factor receptor-targeted immune magnetic liposomes capture circulating colorectal tumor cells efficiently. World J. Gastroenterol. 24, 351–359. doi: 10.3748/wjg.v24.i3.351
Kumari, P., Ghosh, B., and Biswas, S. (2016). Nanocarriers for cancer-targeted drug delivery. J. Drug Target 24, 179–191. doi: 10.3109/1061186x.2015.1051049
Lan, X., Mochida, T., Funasako, Y., Takahashi, K., Sakurai, T., and Ohta, H. (2017). Thermochromic magnetic ionic liquids from cationic nickel(II) complexes exhibiting intramolecular coordination equilibrium. Chemistry 23, 823–831. doi: 10.1002/chem.201604420
Li, C., Armstrong, J. P., Pence, I. J., Kit-Anan, W., Puetzer, J. L., Correia Carreira, S., et al. (2018). Glycosylated superparamagnetic nanoparticle gradients for osteochondral tissue engineering. Biomaterials 176, 24–33. doi: 10.1016/j.biomaterials.2018.05.029
Li, D. W., Zhou, J., Zhang, M. M., Ma, Y. Z., Yang, Y. Y., Han, X., et al. (2020). Long-term delivery of alendronate through injectable Tetra-PEG hydrogel to promote osteoporosis therapy. Biomater. Sci. 8, 3138–3146. doi: 10.1039/D0BM00376J
Li, X., Wei, J., Aifantis, K. E., Fan, Y., Feng, Q., Cui, F. Z., et al. (2016). Current investigations into magnetic nanoparticles for biomedical applications. J. Biomed. Mater. Res. A 104, 1285–1296. doi: 10.1002/jbm.a.35654
Li, X., Wei, Z., Lv, H., Wu, L., Cui, Y., Yao, H., et al. (2019a). Iron oxide nanoparticles promote the migration of mesenchymal stem cells to injury sites. Int. J. Nanomed. 14, 573–589. doi: 10.2147/ijn.S184920
Li, Y., Liang, T., Wang, R., He, B., Gong, Y., and Wang, H. (2019b). Encapsulation of Fe3O4 between copper nanorod and thin TiO2 film by ALD for lithium-ion capacitors. ACS Appl. Mater. Interfaces 11, 19115–19122. doi: 10.1021/acsami.9b03454
Lin, J., Zhang, J., Sun, H., Lin, Q., Guo, Z., Yang, H., et al. (2018a). Structural and magnetic property of Cr(3+) substituted cobalt ferrite nanomaterials prepared by the Sol-Gel method. Materials 11:2095. doi: 10.3390/ma11112095
Lin, W. C., Chuang, C. C., Wang, P. T., and Tang, C. M. (2018b). A comparative study on the direct and pulsed current electrodeposition of cobalt-substituted hydroxyapatite for magnetic resonance imaging application. Materials 12:116. doi: 10.3390/ma12010116
Liu, H. Y., Wang, X., Cao, Y. X., Yang, Y. Y., Yang, Y. T., Gao, Y. F., et al. (2020). Freezing-tolerant, highly sensitive strain and pressure sensors assembled from ionic conductive hydrogels with dynamic cross-links. ACS Appl. Mater. Interfaces 12, 25334–25344. doi: 10.1021/acsami.0c06067
Liu, S. J., Wang, L. J., Qiao, Y., Zhang, H., Li, L. P., Sun, J. H., et al. (2018). A promising magnetic resonance stem cell tracer based on natural biomaterials in a biological system: manganese(II) chelated to melanin nanoparticles. Int. J. Nanomed. 13, 1749–1759. doi: 10.2147/ijn.S157508
Liu, Y., Cui, T., Wu, T., Li, Y., and Tong, G. (2016). Excellent microwave-absorbing properties of elliptical Fe2O2 nanorings made by a rapid microwave-assisted hydrothermal approach. Nanotechnology 27:165707. doi: 10.1088/0957-4484/27/16/165707
Liu, Z. Y., Liu, J. H., Cui, X., Wang, X., Zhang, L. C., and Tang, P. F. (2020). Recent advances on magnetic sensitive hydrogels in tissue engineering. Front. Chem. 8:124. doi: 10.3389/fchem.2020.00124
Loi, F., Córdova, L. A., Pajarinen, J., Lin, T. H., Yao, Z., and Goodman, S. B. (2016). Inflammation, fracture and bone repair. Bone 86, 119–130. doi: 10.1016/j.bone.2016.02.020
Lu, J. W., Yang, F., Ke, Q. F., Xie, X. T., and Guo, Y. P. (2018). Magnetic nanoparticles modified-porous scaffolds for bone regeneration and photothermal therapy against tumors. Nanomedicine 14, 811–822. doi: 10.1016/j.nano.2017.12.025
Lugert, S., Unterweger, H., Mühlberger, M., Janko, C., Draack, S., Ludwig, F., et al. (2019). Cellular effects of paclitaxel-loaded iron oxide nanoparticles on breast cancer using different 2D and 3D cell culture models. Int. J. Nanomed. 14, 161–180. doi: 10.2147/ijn.S187886
Luo, C., Yang, X., Li, M., Huang, H., Kang, Q., Zhang, X., et al. (2018). A novel strategy for in vivo angiogenesis and osteogenesis: magnetic micro-movement in a bone scaffold. Artif. Cells Nanomed. Biotechnol. 46, 636–645. doi: 10.1080/21691401.2018.1465947
Mahdavinia, G. R., Soleymani, M., Etemadi, H., Sabzi, M., and Atlasi, Z. (2018). Model protein BSA adsorption onto novel magnetic chitosan/PVA/laponite RD hydrogel nanocomposite beads. Int. J. Biol. Macromol. 107, 719–729. doi: 10.1016/j.ijbiomac.2017.09.042
Majidi, S., Sehrig, F. Z., Farkhani, S. M., Goloujeh, M. S., and Akbarzadeh, A. (2016). Current methods for synthesis of magnetic nanoparticles. Artif. Cells Nanomed. Biotechnol. 44, 722–734. doi: 10.3109/21691401.2014.982802
Maleki, A. (2012). Fe3O4/SiO2 nanoparticles: an efficient and magnetically recoverable nanocatalyst for the one-pot multicomponent synthesis of diazepines. Tetrahedron 68, 7827–7833. doi: 10.1016/j.tet.2012.07.034
Maleki, A. (2013). One-pot multicomponent synthesis of diazepine derivatives using terminal alkynes in the presence of silica-supported superparamagnetic iron oxide nanoparticles. Tetrahed. Lett. 54, 2055–2059. doi: 10.1016/j.tetlet.2013.01.123
Maleki, A. (2014). One-pot three-component synthesis of pyrido 2 ',1 ': 2,3 imidazo 4,5-c isoquinolines using Fe3O4@SiO2-OSO3H as an efficient heterogeneous nanocatalyst. Rsc Adv. 4, 64169–64173. doi: 10.1039/c4ra10856f
Maleki, A. (2018). Green oxidation protocol: Selective conversions of alcohols and alkenes to aldehydes, ketones and epoxides by using a new multiwall carbon nanotube-based hybrid nanocatalyst via ultrasound irradiation. Ultrason Sonochem. 40, 460–464. doi: 10.1016/j.ultsonch.2017.07.020
Manjua, A. C., Alves, V. D., Crespo, J. G., and Portugal, C. A. M. (2019). Magnetic responsive PVA hydrogels for remote modulation of protein sorption. ACS Appl. Mater. Interf. 11, 21239–21249. doi: 10.1021/acsami.9b03146
Mazzone, D. G., Raymond, S., Gavilano, J. L., Ressouche, E., Niedermayer, C., Birk, J. O., et al. (2017). Field-induced magnetic instability within a superconducting condensate. Sci. Adv. 3:e1602055. doi: 10.1126/sciadv.1602055
Michalski, M. N., and McCauley, L. K. (2017). Macrophages and skeletal health. Pharmacol. Ther. 174, 43–54. doi: 10.1016/j.pharmthera.2017.02.017
Mirabello, G., Lenders, J. J., and Sommerdijk, N. A. (2016). Bioinspired synthesis of magnetite nanoparticles. Chem. Soc. Rev. 45, 5085–5106. doi: 10.1039/c6cs00432f
Mortimer, C. J., and Wright, C. J. (2017). The fabrication of iron oxide nanoparticle-nanofiber composites by electrospinning and their applications in tissue engineering. Biotechnol. J. 12:7. doi: 10.1002/biot.201600693
Narayanaswamy, V., Obaidat, I. M., Kamzin, A. S., Latiyan, S., Jain, S., Kumar, H., et al. (2019). Synthesis of graphene Oxide-Fe(3)O(4) based nanocomposites using the mechanochemical method and in vitro magnetic hyperthermia. Int. J. Mol. Sci. 20:3368. doi: 10.3390/ijms20133368
Nejadnik, H., Tseng, J., and Daldrup-Link, H. (2019). Magnetic resonance imaging of stem cell-macrophage interactions with ferumoxytol and ferumoxytol-derived nanoparticles. Wiley Interdiscip. Rev. Nanomed. Nanobiotechnol. 11:e1552. doi: 10.1002/wnan.1552
Nosrati, H., Salehiabar, M., Manjili, H. K., Danafar, H., and Davaran, S. (2018). Preparation of magnetic albumin nanoparticles via a simple and one-pot desolvation and co-precipitation method for medical and pharmaceutical applications. Int. J. Biol. Macromol. 108, 909–915. doi: 10.1016/j.ijbiomac.2017.10.180
Pandi, K., Viswanathan, N., and Meenakshi, S. (2019). Hydrothermal synthesis of magnetic iron oxide encrusted hydrocalumite-chitosan composite for defluoridation studies. Int. J. Biol. Macromol. 132, 600–605. doi: 10.1016/j.ijbiomac.2019.03.115
Parfenov, V. A., Mironov, V. A., Koudan, E. V., Nezhurina, E. K., Karalkin, P. A., Pereira, F. D., et al. (2020). Fabrication of calcium phosphate 3D scaffolds for bone repair using magnetic levitational assembly. Sci. Rep. 10:4013. doi: 10.1038/s41598-020-61066-3
Peng, S., Wang, C., Xie, J., and Sun, S. (2006). Synthesis and stabilization of monodisperse Fe nanoparticles. J. Am. Chem. Soc. 128, 10676–10677. doi: 10.1021/ja063969h
Ponti, A., Raza, M. H., Pantò, F., Ferretti, A. M., Triolo, C., Patanè, S., et al. (2020). Structure, defects, and magnetism of electrospun hematite nanofibers silica-coated by atomic layer deposition. Langmuir 36, 1305–1319. doi: 10.1021/acs.langmuir.9b03587
Qi, H., Liu, C., Long, L., Ren, Y., Zhang, S., Chang, X., et al. (2016). Blood exosomes endowed with magnetic and targeting properties for cancer therapy. ACS Nano 10, 3323–3333. doi: 10.1021/acsnano.5b06939
Qian, W., Qian, M., Wang, Y., Huang, J., Chen, J., Ni, L., et al. (2018). Combination glioma therapy mediated by a dual-targeted delivery system constructed using OMCN-PEG-Pep22/DOX. Small 14:e1801905. doi: 10.1002/smll.201801905
Rotherham, M., Henstock, J. R., Qutachi, O., and El Haj, A. J. (2018). Remote regulation of magnetic particle targeted Wnt signaling for bone tissue engineering. Nanomedicine 14, 173–184. doi: 10.1016/j.nano.2017.09.008
Sanli, U. T., Jiao, C., Baluktsian, M., Grévent, C., Hahn, K., Wang, Y., et al. (2018). 3D Nanofabrication of high-resolution multilayer fresnel zone plates. Adv. Sci. 5:1800346. doi: 10.1002/advs.201800346
Saygili, H. (2019). Hydrothermal synthesis of magnetic nanocomposite from biowaste matrix by a green and one-step route: characterization and pollutant removal ability. Bioresour. Technol. 278, 242–247. doi: 10.1016/j.biortech.2019.01.103
Schwab, M. M., Himmel, D., Kacprzak, S., Radtke, V., Kratzert, D., Weis, P., et al. (2018). Synthesis, characterisation and reactions of truly cationic Ni(I) -phosphine complexes. Chemistry 24, 918–927. doi: 10.1002/chem.201704436
Shen, B., Ma, Y., Yu, S., and Ji, C. (2016). Smart multifunctional magnetic nanoparticle-based drug delivery system for cancer thermo-chemotherapy and intracellular imaging. ACS Appl. Mater. Interfaces 8, 24502–24508. doi: 10.1021/acsami.6b09772
Singh, R. K., El-Fiqi, A. M., Patel, K. D., and Kim, H.-W. (2012a). A novel preparation of magnetic hydroxyapatite nanotubes. Mater. Lett. 75, 130–133. doi: 10.1016/j.matlet.2012.01.129
Singh, R. K., and Kim, H.-W. (2013). Inorganic nanobiomaterial drug carriers for medicine. Tissue Eng. Regene. Med. 10, 296–309. doi: 10.1007/s13770-013-1092-y
Singh, R. K., Kim, T. H., Patel, K. D., Knowles, J. C., and Kim, H. W. (2012b). Biocompatible magnetite nanoparticles with varying silica-coating layer for use in biomedicine: physicochemical and magnetic properties, and cellular compatibility. J. Biomed. Mater. Res. A 100, 1734–1742. doi: 10.1002/jbm.a.34140
Singh, R. K., Patel, K. D., Kim, J. J., Kim, T. H., Kim, J. H., Shin, U. S., et al. (2014). Multifunctional hybrid nanocarrier: magnetic CNTs ensheathed with mesoporous silica for drug delivery and imaging system. ACS Appl. Mater. Interfaces 6, 2201–2208. doi: 10.1021/am4056936
Son, B., Kim, H. D., Kim, M., Kim, J. A., Lee, J., Shin, H., et al. (2015). Physical stimuli-induced chondrogenic differentiation of mesenchymal stem cells using magnetic nanoparticles. Adv. Healthc. Mater. 4, 1339–1347. doi: 10.1002/adhm.201400835
Sun, M., Fan, X., Meng, X., Song, J., Chen, W., Sun, L., et al. (2019). Magnetic biohybrid micromotors with high maneuverability for efficient drug loading and targeted drug delivery. Nanoscale 11, 18382–18392. doi: 10.1039/c9nr06221a
Sweeney, S. K., Manzar, G. S., Zavazava, N., and Assouline, J. G. (2018). Tracking embryonic hematopoietic stem cells to the bone marrow: nanoparticle options to evaluate transplantation efficiency. Stem Cell Res. Ther. 9:204. doi: 10.1186/s13287-018-0944-8
Swietek, M., BroŽ, A., Tarasiuk, J., Wroński, S., Tokarz, W., Kozieł, A., et al. (2019). Carbon nanotube/iron oxide hybrid particles and their PCL-based 3D composites for potential bone regeneration. Mater. Sci. Eng. C Mater. Biol. Appl. 104:109913. doi: 10.1016/j.msec.2019.109913
Tahir, M. N., Herzberger, J., Natalio, F., Kohler, O., Branscheid, R., Mugnaioli, E., et al. (2016). Hierachical Ni@Fe2O3 superparticles through epitaxial growth of gamma-Fe2O3 nanorods on in situ formed Ni nanoplates. Nanoscale 8:9548–9555. doi: 10.1039/c6nr00065g
Tang, X., Guo, W., Yang, R., Yan, T., Tang, S., and Li, D. (2017). Acetabular reconstruction with femoral head autograft after intraarticular resection of periacetabular tumors is durable at short-term followup. Clin. Orthop. Relat. Res. 475, 3060–3070. doi: 10.1007/s11999-017-5505-4
Theruvath, A. J., Nejadnik, H., Muehe, A. M., Gassert, F., Lacayo, N. J., Goodman, S. B., et al. (2018). Tracking cell transplants in femoral osteonecrosis with magnetic resonance imaging: a proof-of-concept study in patients. Clin. Cancer Res. 24, 6223–6229. doi: 10.1158/1078-0432.Ccr-18-1687
Vangijzegem, T., Stanicki, D., and Laurent, S. (2019). Magnetic iron oxide nanoparticles for drug delivery: applications and characteristics. Expert Opin. Drug Deliv. 16, 69–78. doi: 10.1080/17425247.2019.1554647
Wan, C., and Li, J. (2015). Synthesis of well-dispersed magnetic CoFe2O4 nanoparticles in cellulose aerogels via a facile oxidative co-precipitation method. Carbohydr. Polym. 134, 144–150. doi: 10.1016/j.carbpol.2015.07.083
Wang, G., Ceylan, Y. S., Cundari, T. R., and Dias, H. V. R. (2017). Heterobimetallic Silver-iron complexes involving Fe(CO)5 ligands. J. Am. Chem. Soc. 139, 14292–14301. doi: 10.1021/jacs.7b08595
Wang, H., Zhao, S., Zhou, J., Zhu, K., Cui, X., Huang, W., et al. (2015). Biocompatibility and osteogenic capacity of borosilicate bioactive glass scaffolds loaded with Fe(3)O(4) magnetic nanoparticles. J. Mater. Chem. B 3, 4377–4387. doi: 10.1039/c5tb00062a
Wang, J., Zhang, Q., Shao, X., Ma, J., and Tian, G. (2018). Properties of magnetic carbon nanomaterials and application in removal organic dyes. Chemosphere 207, 377–384. doi: 10.1016/j.chemosphere.2018.05.109
Wang, X., Gao, P. Y., Yang, Y. Y., Guo, H. X., and Wu, D. C. (2018). Dynamic and programmable morphology and size evolution via a living hierarchical self-assembly strategy. Nat. Commun. 9:2772. doi: 10.1038/s41467-018-05142-3
Wang, X., Yang, Y. Y., Shi, Y., and Jia, F. (2020). Editorial: smart hydrogels in tissue engineering and regenerative medicine. Front. Chem. 8:245. doi: 10.3389/fchem.2020.00245
Wang, Z., Liu, X., Ni, S. Q., Zhang, J., Zhang, X., Ahmad, H. A., et al. (2017). Weak magnetic field: apowerful strategy to enhance partial nitrification. Water Res. 120, 190–198. doi: 10.1016/j.watres.2017.04.058
Wu, J., Cheng, X., and Yang, G. (2019). Preparation of nanochitin-contained magnetic chitosan microfibers via continuous injection gelation method for removal of Ni(II) ion from aqueous solution. Int. J. Biol. Macromol. 125, 404–413. doi: 10.1016/j.ijbiomac.2018.11.212
Xia, Y., Chen, H., Zhao, Y., Zhang, F., Li, X., Wang, L., et al. (2019a). Novel magnetic calcium phosphate-stem cell construct with magnetic field enhances osteogenic differentiation and bone tissue engineering. Mater. Sci. Eng. C Mater. Biol. Appl. 98, 30–41. doi: 10.1016/j.msec.2018.12.120
Xia, Y., Guo, Y., Yang, Z., Chen, H., Ren, K., Weir, M. D., et al. (2019b). Iron oxide nanoparticle-calcium phosphate cement enhanced the osteogenic activities of stem cells through WNT/β-catenin signaling. Mater. Sci. Eng. C Mater. Biol. Appl. 104:109955. doi: 10.1016/j.msec.2019.109955
Xia, Y., Sun, J., Zhao, L., Zhang, F., Liang, X. J., Guo, Y., et al. (2018). Magnetic field and nano-scaffolds with stem cells to enhance bone regeneration. Biomaterials 183, 151–170. doi: 10.1016/j.biomaterials.2018.08.040
Xue, X., Lu, R., Liu, M., Li, Y., Li, J., and Wang, L. (2019). A facile and general approach for the preparation of boronic acid-functionalized magnetic nanoparticles for the selective enrichment of glycoproteins. Analyst 144, 641–648. doi: 10.1039/c8an01704b
Yang, F., Lu, J., Ke, Q., Peng, X., Guo, Y., and Xie, X. (2018). Magnetic mesoporous calcium sillicate/chitosan porous scaffolds for enhanced bone regeneration and photothermal-chemotherapy of osteosarcoma. Sci. Rep. 8:7345. doi: 10.1038/s41598-018-25595-2
Yang, Q., Zhou, M., Yang, M., Zhang, Z., Yu, J., Zhang, Y., et al. (2020). High-yield production of few-layer graphene via new-fashioned strategy combining resonance ball milling and hydrothermal exfoliation. Nanomaterials 10:667. doi: 10.3390/nano10040667
Yang, W., Zhu, P., Huang, H., Zheng, Y., Liu, J., Feng, L., et al. (2019). Functionalization of novel theranostic hydrogels with kartogenin-grafted USPIO nanoparticles to enhance cartilage regeneration. ACS Appl. Mater. Interfaces 11, 34744–34754. doi: 10.1021/acsami.9b12288
Yu, C., Geng, J., Zhuang, Y., Zhao, J., Chu, L., Luo, X., et al. (2016). Preparation of the chitosan grafted poly (quaternary ammonium)/Fe3O4 nanoparticles and its adsorption performance for food yellow 3. Carbohydr. Polym. 152, 327–336. doi: 10.1016/j.carbpol.2016.06.114
Yu, K., Liang, B., Zheng, Y., Exner, A., Kolios, M., Xu, T., et al. (2019). PMMA-Fe(3)O(4) for internal mechanical support and magnetic thermal ablation of bone tumors. Theranostics 9, 4192–4207. doi: 10.7150/thno.34157
Yun, H. M., Ahn, S. J., Park, K. R., Kim, M. J., Kim, J. J., Jin, G. Z., et al. (2016a). Magnetic nanocomposite scaffolds combined with static magnetic field in the stimulation of osteoblastic differentiation and bone formation. Biomaterials 85, 88–98. doi: 10.1016/j.biomaterials.2016.01.035
Yun, H. M., Kang, S. K., Singh, R. K., Lee, J. H., Lee, H. H., Park, K. R., et al. (2016b). Magnetic nanofiber scaffold-induced stimulation of odontogenesis and pro-angiogenesis of human dental pulp cells through Wnt/MAPK/NF-kappaB pathways. Dent Mater. 32, 1301–1311. doi: 10.1016/j.dental.2016.06.016
Zhang, H., Gan, L., Zhu, X., Wang, J., Han, L., Cheng, P., et al. (2018a). Moderate-intensity 4mT static magnetic fields prevent bone architectural deterioration and strength reduction by stimulating bone formation in streptozotocin-treated diabetic rats. Bone 107, 36–44. doi: 10.1016/j.bone.2017.10.024
Zhang, J., Meng, X., Ding, C., and Shang, P. (2018b). Effects of static magnetic fields on bone microstructure and mechanical properties in mice. Electromagn. Biol. Med. 37, 76–83. doi: 10.1080/15368378.2018.1458626
Zhang, S., Li, L., Li, X., Zhang, J., Xu, K., Li, G., et al. (2020). Electroreductive 4-Pyridylation of electron-deficient alkenes with assistance of Ni(acac)2. Org. Lett. 22, 3570–3575. doi: 10.1021/acs.orglett.0c01014
Zhang, W., Li, X., Zou, R., Wu, H., Shi, H., Yu, S., et al. (2015). Multifunctional glucose biosensors from Fe2O2 nanoparticles modified chitosan/graphene nanocomposites. Sci. Rep. 5:11129. doi: 10.1038/srep11129
Keywords: magnetic nanomaterials, bone tissue repair, magnetic field, magnetic particles, stem cells, tissue engineering scaffolds
Citation: Fan D, Wang Q, Zhu T, Wang H, Liu B, Wang Y, Liu Z, Liu X, Fan D and Wang X (2020) Recent Advances of Magnetic Nanomaterials in Bone Tissue Repair. Front. Chem. 8:745. doi: 10.3389/fchem.2020.00745
Received: 30 May 2020; Accepted: 17 July 2020;
Published: 25 September 2020.
Edited by:
Raghvendra Singh Yadav, Tomas Bata University in Zlín, CzechiaReviewed by:
Ali Maleki, Iran University of Science and Technology, IranRajendra Kumar Singh, Institute of Tissue Regeneration Engineering (ITREN), South Korea
Copyright © 2020 Fan, Wang, Zhu, Wang, Liu, Wang, Liu, Liu, Fan and Wang. This is an open-access article distributed under the terms of the Creative Commons Attribution License (CC BY). The use, distribution or reproduction in other forums is permitted, provided the original author(s) and the copyright owner(s) are credited and that the original publication in this journal is cited, in accordance with accepted academic practice. No use, distribution or reproduction is permitted which does not comply with these terms.
*Correspondence: Xunyong Liu, xunyongliu@126.com; Dongwei Fan, fdw@bjmu.edu.cn; Xing Wang, wangxing@iccas.ac.cn
†These authors have contributed equally to this work