Apigenin as Tumor Suppressor in Cancers: Biotherapeutic Activity, Nanodelivery, and Mechanisms With Emphasis on Pancreatic Cancer
- 1Department of Basic Science, Faculty of Veterinary Medicine, University of Tabriz, Tabriz, Iran
- 2Student Research Committee, Shahrekord University of Medical Sciences, Shahrekord, Iran
- 3Department of Medical Genetics, Faculty of Medicine, Tabriz University of Medical Sciences, Tabriz, Iran
- 4Sabanci University Nanotechnology Research and Application Center (SUNUM), Istanbul, Turkey
- 5Centre for Micro-BioRobotics, Istituto Italiano di Tecnologia, Pisa, Italy
- 6Department of Medical Nanotechnology, Faculty of Advanced Technologies in Medicine, Iran University of Medical Sciences, Tehran, Iran
- 7Department of Pharmacy, Abdul Wali Khan University Mardan, Mardan, Pakistan
- 8Department of Analytical Chemistry, Faculty of Chemistry, University of Kashan, Kashan, Iran
- 9Department of Medical Biotechnology, Faculty of Medicine, Arak University of Medical Science, Arak, Iran
- 10Research Center for Biochemistry and Nutrition in Metabolic Diseases, Institute for Basic Sciences, Kashan University of Medical Sciences, Kashan, Iran
Pancreatic cancer is the most lethal malignancy of the gastrointestinal tract. Due to its propensity for early local and distant spread, affected patients possess extremely poor prognosis. Currently applied treatments are not effective enough to eradicate all cancer cells, and minimize their migration. Besides, these treatments are associated with adverse effects on normal cells and organs. These therapies are not able to increase the overall survival rate of patients; hence, finding novel adjuvants or alternatives is so essential. Up to now, medicinal herbs were utilized for therapeutic goals. Herbal-based medicine, as traditional biotherapeutics, were employed for cancer treatment. Of them, apigenin, as a bioactive flavonoid that possesses numerous biological properties (e.g., anti-inflammatory and anti-oxidant effects), has shown substantial anticancer activity. It seems that apigenin is capable of suppressing the proliferation of cancer cells via the induction of cell cycle arrest and apoptosis. Besides, apigenin inhibits metastasis via down-regulation of matrix metalloproteinases and the Akt signaling pathway. In pancreatic cancer cells, apigenin sensitizes cells in chemotherapy, and affects molecular pathways such as the hypoxia inducible factor (HIF), vascular endothelial growth factor (VEGF), and glucose transporter-1 (GLUT-1). Herein, the biotherapeutic activity of apigenin and its mechanisms toward cancer cells are presented in the current review to shed some light on anti-tumor activity of apigenin in different cancers, with an emphasis on pancreatic cancer.
Introduction
Pancreatic Cancer: A Brief Representation
Pancreatic cancer (PC) is the fourth leading cause of malignancy-associated mortality with <5% 5-years survival. Clinical strategies for the management of this cancer have recently been developed; however, the mortality rate is still mostly unaltered (Siegel et al., 2018). This high rate of mortality is attributed to the aggressive nature of this cancer as well as a lack of efficient therapy methods (Tempero et al., 2017). The high metastatic ability of PC cells, and also, their uncontrolled growth have led to some difficulties in the effective treatment of this life-threatening disorder.
Although chemotherapy and surgery are the most common methods in cancer therapy, growing evidence demonstrates that the aforementioned strategies are only effective in a few numbers of patients. Consequently, radiotherapy is also used to enhance the efficacy of chemotherapy. However, it seems that combination chemotherapy with other anti-tumor agents would be the best strategy for cancer treatment. This is due to the fact that cancer cells are able to acquire resistance toward both radiotherapy and chemotherapy; accordingly, combination chemotherapy facilitates the disruption molecular pathways involved in cancer resistance. Subsequently, the effectiveness of chemotherapy is ameliorated and its clinical trial findings would be more satisfactory. Finding a suitable anti-tumor agent in combination with chemotherapy is of importance in poly-chemotherapy. High anti-tumor activity, multi-targeting, and minimal toxicity are some of the most important properties of an ideal anti-tumor agent (Lee et al., 2019b,c, 2020; Tan and Norhaizan, 2019; Banik et al., 2020; Patra et al., 2020).
To date, a wide variety of strategies were employed in suppressing chemoresistance, and malignant behavior of PC cells. Among them, plant derived-natural products are of importance in PC due to their excellent anti-tumor activity, and capability of enhancing sensitivity in PC cells into chemotherapy (Cheng et al., 2018; Yan et al., 2018). In light of this, much attention was directed toward using plant derived-natural compounds as potential anti-tumor agents for use in combination chemotherapy, and for suppressing malignant behavior and the proliferation of cancer cells (Abotaleb et al., 2020; Liskova et al., 2020; Varghese et al., 2020). In respect to the fact that a variety of molecular pathways are involved in the progression and proliferation of PC cells such as Wnt (Xu et al., 2020), Nrf 2 (Krajka-Kuzniak et al., 2020), long non-coding RNAs (lncRNAs) (Yin et al., 2020), and microRNAs (miRs) (Wang et al., 2020) its effective therapy relies on using anti-tumor compounds with the capability of the induction of onco-suppressor pathways, and the inhibition of oncogene ones. Notably, naturally occurring compounds are capable of modulating molecular pathways and mechanisms. It seems that Akt is an oncogene pathway involved in the proliferation and viability of PC cells. The administration of curcumin, as a naturally occurring nutraceutical compounds, remarkably reduces Akt expression by suppressing its upstream modulator epidermal growth factor (EGF), leading to a decrease in growth and malignant behavior of the PC cells (Li et al., 2019). The curcumin analogs have demonstrated more inhibitory effects on the proliferation of pancreatic cancer cells due to their enhanced bioavailability (Nagaraju et al., 2019). Besides, phytochemicals are able to interfere with metastasis and the invasion of PC cells by suppressing epithelial-to-mesenchymal transition (EMT) (Hoca et al., 2019). It is worth mentioning that plant derived-natural compounds are beneficial in enhancing the sensitivity of PC in chemotherapy (Zhou et al., 2019). These studies are in line with the potentiality of herbal-based products in PC therapy. Herein, we aim to explore the anti-tumor activity of apigenin, as a natural compound, on different cancers with a special focus on PC.
Apigenin: Chemical Structure and Biological Functions
Apigenin (4′,5,7-trihydroxyflavone) is a plant-derived material belonging to the flavone category that is the aglycone of several naturally occurring glycosides. The molecular formula and molecular weight of apigenin are C15H10O5 and ~270 g/mol, respectively. Flavones and several of their synthetic derivatives are well-known for their biological and therapeutic activities, including anti-oxidant, anti-inflammatory, anti-cancer, ant-genotoxic, anti-allergic, neuroprotective, cardioprotective, and antimicrobial (Catarino et al., 2015). Apigenin is a yellow crystalline solid and its main non-pharmaceutical application is its use to dye wool. Compared to other structurally related flavonoids, apigenin was a useful and health promoting agent in recent years due to its low toxicity and significant effects on normal vs. cancer cells (Gupta et al., 2001). The solid therapeutic potential of apigenin against various diseases was proven through evidence achieved by numerous studies. The prior art has not been able to provide a robust proof to indicate that apigenin increases the negative metabolic responses in vivo when consumed as part of a normal diet. However, the results of some investigations in Swiss mice proposed the oxidative stress-induced liver damage, which may be due to the stimulation of multiple genes via apigenin at higher doses (Singh et al., 2012). The strong anti-oxidant and anti-inflammatory activities of apigenin are a substantial reason for its possible cancer preventive effects (Singh et al., 2012). Encouraging metal chelation, scavenging free radicals, and triggering phase II detoxification enzymes in cell cultures as well as in vivo tumor models are also functions of apigenin (Middleton et al., 2000). More importantly, apigenin significantly contributes in the prevention of cancer by inducing apoptosis in different cell lines as well as animal models (Kaur et al., 2008).
Pharmacokinetics of Apigenin: A Brief Explanation
Owing to outstanding pharmacological activities of apigenin, a number of studies have exploited the pharmacokinetics of apigenin to demonstrate its absorption, metabolism, distribution, and excretion. Such findings are beneficial for directing further studies to use an optimal dose of apigenin in disease therapy (Wang et al., 2019a). It was reported that after the consumption of polyphenols, 5–10% of apigenin may be absorbed (Cardona et al., 2013). The gastrointestinal tract (GIT) is involved in the absorption of apigenin before its arrival in blood circulation and the liver. Upon aglycone apigenin administration, its immediate absorption occurs in the intestine (based on a perfused rat intestinal model) (Liu and Hu, 2002). It is worth mentioning that different parts of the intestine have various absorption routes for apigenin. For instance, passive and active carrier-mediated saturable mechanisms contribute to the absorption of apigenin in the duodenum and jejunum, while its absorption occurs in the ileum and colon via passive transportation (Zhang et al., 2012). However, there are conflicting data about the rate of apigenin absorption. Although one study is in line with the fact that apigenin has a low absorption rate after oral administration (appearing in blood circulation after 24 h) (Gradolatto et al., 2005), another research confirms its high absorption rate (appearing in blood circulation after 3.9 h) (Chen et al., 2007). Consequently, more studies should be conducted to show the absorption rate of apigenin. In terms of distribution, various studies were performed and it was reported that apigenin is distributed in different organs of the body including the kidney, intestine, and liver. Moreover, half of apigenin intake appeared in urine and feces (Liu and Hu, 2002; Gradolatto et al., 2005; Cai et al., 2007; Wan et al., 2007).
Increasing evidence demonstrates that the metabolism of apigenin consists of two major phases. The phase I metabolism of apigenin occurs in the liver, and at the presence of liver enzymes such as cytochrome P450 with collaboration of nicotinamide adenine dinucleotide phosphate (NADPH) and flavin-containing monooxygenase (FMO) (Cardona et al., 2013; Tang et al., 2017). Enteric and enterohepatic cycling participate in the biotransformation of apigenin in phase II metabolism (Chen et al., 2007). Glucuronidation and sulfation are essential for phase II metabolism (Tang et al., 2017). During metabolism, apigenin is bio-transformed into metabolites including luteolin (Lut) and sulfated and glucuronidated conjugates (Chen et al., 2003; Gradolatto et al., 2005). Regarding the excretion, apigenin appeared in both urine and feces with more concentration in urine. The age and sex of rats are crucial factors that affect the excretion of apigenin. Furthermore, it was shown that metabolism and the excretion of apigenin occur in a slow process, confirming accumulation of apigenin in the body (Gradolatto et al., 2005).
Chemistry of Apigenin: An Overview
Apigenin and its derivatives are found in several sorts of plants, e.g., fruits, vegetables, nuts, citrus, tea, chamomile, thyme, celery, and celeriac in its glycoside form (Figure 1) (Yan et al., 2017; Wang et al., 2019b). Among glycoside forms, apigenin-7-O-glucoside is the major one. In terms of solubility, apigenin is not soluble in water and non-polar solvents (e.g., silicon fluid), while it is soluble in organic solvents such as dimethylsulfoxide (DMSO) (Li et al., 1997; Zhang et al., 2012; Lakshmanan et al., 2015; Wang et al., 2017). It was demonstrated that glycoside and acylated derivatives of apigenin have more solubility in water, compared to apigenin (Shukla and Gupta, 2010). Such changes in the hydrophilicity of apigenin affects its absorption and bioavailability. It was shown that when apigenin is attached into β-glycosides, it possesses the highest bioavailability among other forms (Patel et al., 2007). The excellent therapeutic and biological activities of apigenin are due to the presence of glucosides that promote the stability of apigenin (Gurung et al., 2013). Regarding its storage, it is recommended that apigenin be stored at −20°C, since it is unstable at room temperature (Patel et al., 2007). It is worth mentioning that the degradation of natural compounds rely on the presence of special structures. For instance, hydroxyl groups enhance degradation, while sugar moiety and hydroxyl groups reduce the rate of degradation (Biesaga, 2011).
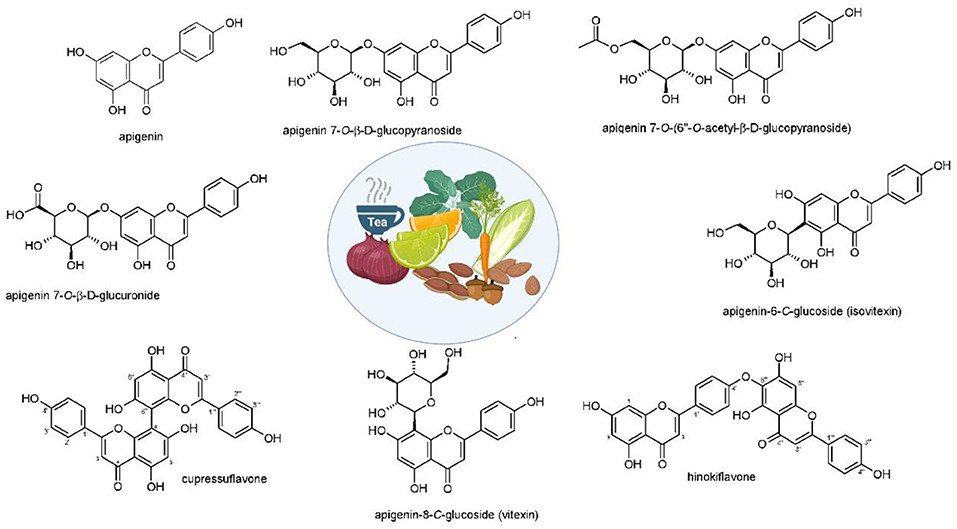
Figure 1. Structures and sources of apigenin and its glycosidic, glucuronide, acetylated, and methyl ester derivatives. Reprinted with modification from Salehi et al. (2019).
In respect to the poor bioavailability of apigenin resulted from its slow absorption and metabolism, several studies were conducted on developing nanocarriers for enhancing its bioavailability and improving its therapeutic effects. To date, various types of nanoparticles including liposomes and polymeric nanoparticles were developed for the delivery of apigenin (Karim et al., 2017; Pápay et al., 2017a,b; Telange et al., 2017; Alshehri et al., 2019). Notably, they were able to remarkably enhance therapeutic effects of apigenin and, accordingly, its ability in the treatment of different disorders. In section Apigenin-loaded nanovehicles, we specifically discuss role of nanoparticles in promoting anti-tumor activity of apigenin against cancer cells along with enhancing the efficacy of chemotherapy.
Apigenin for Cancer Therapy
In addition to anti-inflammatory and anti-oxidant effects, apigenin possesses a significant anti-cancer property in different types of cancer cells, such as breast cancer (Perrott et al., 2017), liver cancer (Qin et al., 2016), PC (Johnson and de Mejia, 2013), prostate cancer (Shukla et al., 2014a), lung cancer (Pan et al., 2013), and colon cancer (Lee et al., 2014). In this section, we provide discussions about the anti-tumor activity of apigenin, and its efficacy in negatively affecting both the proliferation and migration of cancer cells.
Carcinogenesis is known as a multistage procedure that is accompanied by a series of genetic and epigenetic alterations, resulting in the initiation, promotion, and development of cancer (Farhood et al., 2019; Mortezaee et al., 2019a; Woo et al., 2019). Cancer treatment strategies include eradicating tumor cells by stimulating cell apoptosis or preventing cancer cell proliferation by inducing cell cycle arrest (Mortezaee et al., 2019b,c,d). With these remedies, cancer turns into a chronic disease and the survival of patients can be prolonged. Encouraging apoptosis or autophagy, regulating cell cycle, preventing tumor cell migration and invasion, and triggering the patient's immune response were suggested as current strategies (Najafi et al., 2018, 2019a,b; Hashemi Goradel et al., 2019). So far, all of these antitumor activities of apigenin within diverse types of tumors were reported in vitro and in vivo models. Table 1 represents an overview of anti-cancer features of apigenin and the involved signaling pathways.
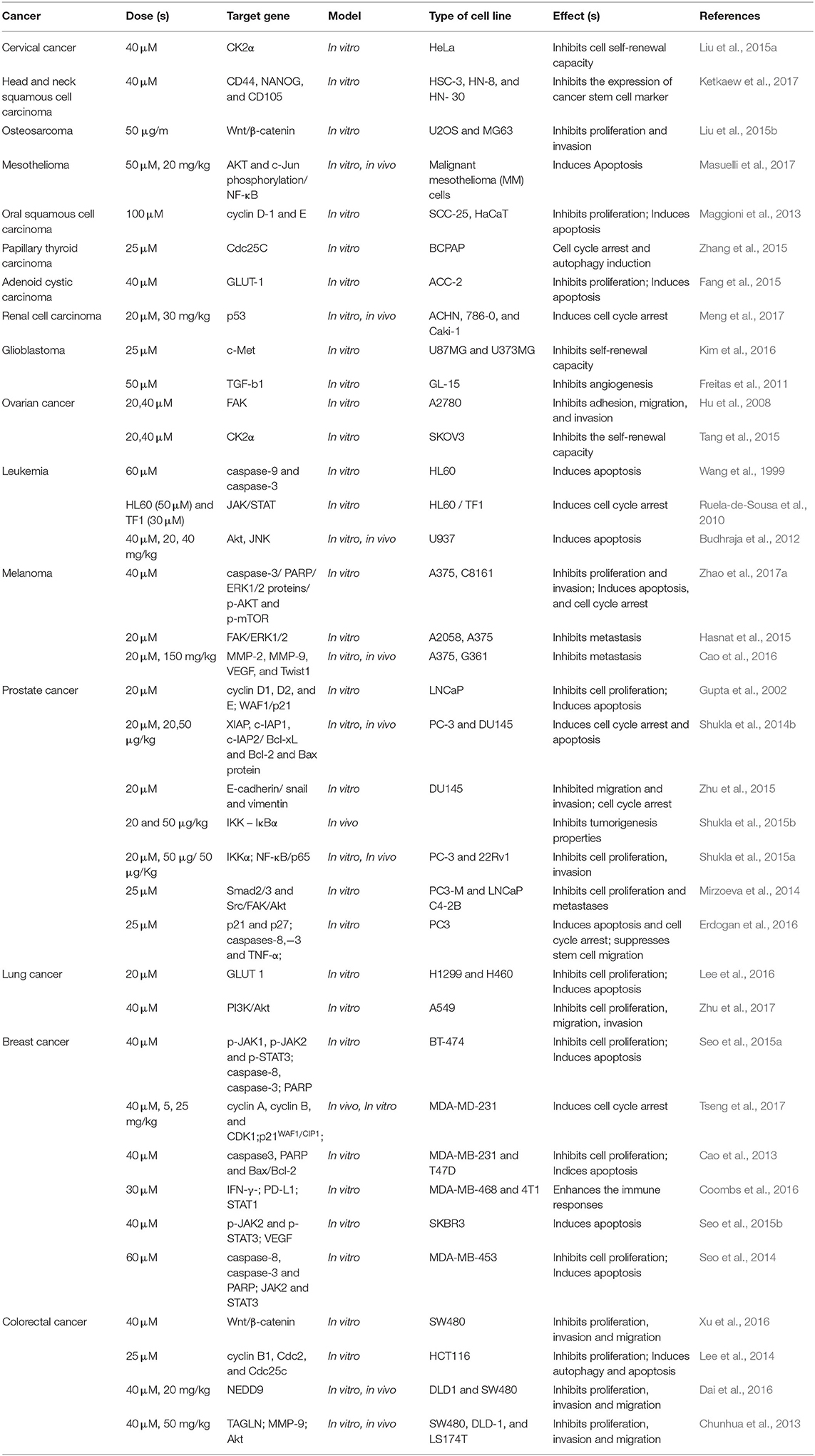
Table 1. Selected in vitro and in vivo studies on the therapeutic effects of apigenin in various cancers.
Apigenin in Induction of Cell Cycle Arrest
Another important and key feature of cancer is its uncontrolled and rapid cell division (Farhood et al., 2020). Therefore, targeting the growth of cancer cells is pivotal for suppressing cancer. One of the abnormalities in cancer cells is proliferation without paying attention to checkpoints of cell cycle. Notably, phytochemicals have demonstrated great potential in the activation of checkpoints and the induction of cell cycle arrest in cancer cells to limit their growth (Farooqi et al., 2019; Aggarwal et al., 2020). Based on the documentation presented, one of the prominent roles of apigenin is to modulate the cell cycle and block the cellular phase at the G2/M or G0/G1 checkpoint, which hinders cancer cell proliferation. In a study which was conducted to determine the effect of apigenin in human colorectal carcinoma HCT116 cells, it was shown that treatment with this flavone (0–50 μM) potentially inhibits cell growth through inducing cell arrest at the G2/M phase; it is associated with the suppression of the expression level of both cyclin B1 as well as both Cdc2 and Cdc25c which are cyclin B1 activating partners, and also an increase of the expression level of cell cycle inhibitors, p53 and p21WAF1/CIP1 (Lee et al., 2014). Also, in experiments performed by the Western blot technique, it was found that the expression levels of cyclin A, cyclin B, and cyclin-dependent kinase-1 (CDK1) were repressed by apigenin treatment in human breast cancer cell line MDA-MB-231. Based on the findings, apigenin (0–40 μM) led to the up-regulation of p21WAF1/CIP1 and enhanced the interaction of p21WAF1/CIP1 with a nuclear proliferating cell antigen (PCNA) preventing cell cycle development at the G2/M stage (Tseng et al., 2017).
The inhibitory effect of apigenin on the cell cycle can be related to its impact on genetic materials. Synthesis of genetic materials is a critical step for the proliferation of cancer cells, and any impairment or damage in DNA can lead to growth inhibition (Gourley et al., 2019). Apigenin follows a same method in suppressing the proliferation of cancer cells. It seems that in a time- and dose-dependent manner, apigenin (0–80 μM) causes DNA damage and encourages G2/M phase cell cycle arrest through ataxia telangiectasia mutated (ATM) modulation (Meng et al., 2017).
Cancer cells need high energy in order to grow and glucose transporter-1 (GLUT-1) participates in cancer proliferation by providing high energy via enhancing glucose uptake. Inhibition of GLUT-1 is a promising strategy in cancer therapy, and phytochemicals have demonstrated great potential in this way (Zambrano et al., 2019). Considering the functions of apigenin (10–160 μM) in adenoid cystic carcinoma (ACC), it causes G2/M-phase arrest, and ACC-2 cell growth and proliferation inhibition in a dose- and time-dependent manner via lessening the expression level of GLUT-1 (Fang et al., 2015).
As it was mentioned earlier, DNA damage is induced by apigenin to trigger cell cycle arrest. One of the ways to stimulate DNA damage is by providing oxidative stress. Enhancing the generation of reactive oxygen species (ROS) is associated with oxidative stress that subsequently, induces DNA damage in cancer cells, and inhibits their proliferation (Shuai et al., 2019; Srinivas et al., 2019). Through apigenin (12.5–100 μM) treatment in human papillary thyroid carcinoma BCPAP cells, G2/M cell cycle arrest occurred by down-regulating the Cdc25c expression level and also, the accumulation of ROS produced were stimulated which triggered DNA damage (Zhang et al., 2015).
Moreover, cell cycle arrest at the G0/G1 or S checkpoints can be induced by apigenin. Apigenin leads to G1 arrest of cell cycle progression in human prostate cancer LNCaP cells. The expression level of some proteins like cyclin D1, D2, and E and their activating partners CDK2, 4, and 6 were reduced noticeably, while the expression of p21WAF1/CIP1 and p27KIP1 were boosted simultaneously by apigenin treatment (10 μM). The induction of p21WAF1/CIP1 seems to be transcriptionally up-regulated and it was dependent on p53 (Gupta et al., 2002). In addition, cell cycle arrest at G0/G1 as well as G2/M checkpoints were triggered through treatment with apigenin (100 μM) in an oral squamous cell carcinoma cell line SCC-25. Also, it was related to the decreased expression of cyclin D1 and E, and the inactivation of CDK1 (Maggioni et al., 2013).
Notably, cancer cells are able to acquire resistance to chemotherapy-mediated cell cycle arrest. Administration of apigenin is beneficial in suppressing chemoresistance, and sensitizing cancer cells in chemotherapy-mediated cell cycle arrest. It is held that apigenin (1–200 μM) is able to induce cell cycle arrest at the G2/M phase. Notably, in this study, it was demonstrated that apigenin induces cell cycle arrest in a dose-dependent manner, so that the highest concentration of apigenin (200 μM) induces cell cycle arrest at the S phase and the highest inhibitory effect on the proliferation of imatinib-resistant cancer cells (Solmaz et al., 2014). Taking everything into account, the results presented reveal that apigenin may regulate cell cycle progression in a dose-dependent and/or cell line specific manner (Figure 2).
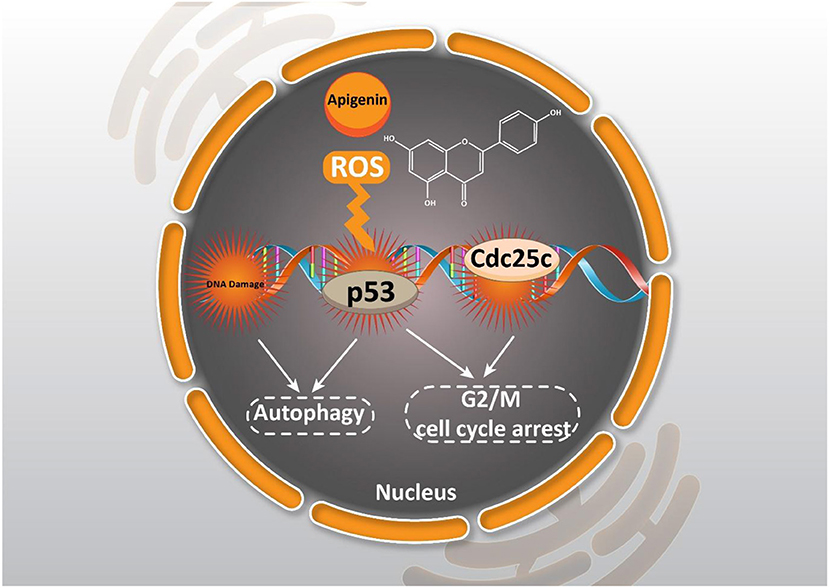
Figure 2. Effect of apigenin on autophagy in cancer. Apigenin affecting ROS generation, DNA damage, and cell cycle arrest could induce autophagy and cell cycle arrest.
Apigenin and Programmed Cell Death
Apoptosis, a type of programmed cell death, involves energy-dependent cascade events and diverse distinct morphological characteristics (Elmore, 2007; Mortezaee et al., 2019d). There are two main cascades involved in the apoptosis process: the extrinsic (death receptor) pathway as well as the intrinsic (mitochondrial) pathway (Chong et al., 2020). Apoptosis is a vital procedure in which undesirable cells are removed under physiological circumstances (Sun et al., 2020). An important feature of cancer cells that distinguishes them from normal cells is their escape from apoptosis (Deng et al., 2020). Therefore, one of the imperative strategies to fight cancer cells and to treat cancer is to stimulate apoptosis of these cells, in which they target apoptotic pathways with chemotherapeutic agents (Liu et al., 2020; Maruszewska and Tarasiuk, 2020). It was revealed that apigenin can be considered as an influential factor in inducing apoptosis through the intrinsic or extrinsic pathway of human cancer cells.
The intrinsic apoptotic pathway is regulated via the Bcl-2 family of proteins, such as Bcl-2, Bcl-xL, Bcl-w, and Mcl-1, which suppress this pathway, while Bad, Bak, Bax, Bid, and Bim cause apoptosis (Billard, 2012; Vela and Marzo, 2015; Zheng et al., 2016). Pro-apoptotic protein up-regulation and/or pro-survival members down-regulation are the functions of apigenin (20 μM), in that way the intrinsic apoptotic cascade is induced. An apoptosis event was caused through the treatment of the androgen-refractory human prostate cancer cell lines PC-3 and DU145 and also, it led to a decrease in cell feasibility triggered by a decline in Bcl-2 and Bcl-xL and an enhancement in the active form of the Bax protein, attended by dose-dependent prevention of XIAP, c-IAP1, c-IAP2, and survivin proteins (Shukla et al., 2014b). In addition, apigenin treatment (0–100 μM) in human promyelocytic leukemia HL-60 cells leads to a diminution in mitochondrial outer membrane potential, releasing cytochrome c from the mitochondria into the cytosol, and encouraging both procaspase-9 processing and cell apoptosis through the intrinsic apoptotic pathway (Wang et al., 1999). Furthermore, apigenin has also been reported to induce apoptosis by altering the ratio of pro-apoptotic to pro-survival mitochondrial proteins. Ratio of Bax / Bcl-2 in favor of cell apoptosis is improved in prostate cancer cells by the means of apigenin (10 μM) (Gupta et al., 2002). Obviously, apigenin alone is capable of inducing mitochondrial-dependent apoptosis in various kinds of cancer cells (Figure 3) (Das et al., 2012; Lim et al., 2016; Wang and Zhao, 2017).
Moreover, it seems that apigenin is advantageous in boosting chemotherapy-mediated cell apoptosis via affecting mitochondrial proteins. It is said that the administration of apigenin (20 μM) enhances the expression of the pro-apoptotic factor Bim, while it decreases expression of Mcl-1. So, co-administration of apigenin with a Bcl-2 inhibitor Navitoclax promotes mitochondria-mediated cell apoptosis (Shao et al., 2013). In addition to its role in inducing an intrinsic apoptotic pathway, apigenin has a function in inducing the apoptosis process in cells through an extrinsic apoptotic pathway or even both the extrinsic and intrinsic pathways. To further examine the role of apigenin in human breast cancer BT-474 cells, Seo et al. carried out a series of experiments and it was reported that apigenin treatment neither affected the levels of Bcl-2 and Bax nor declined the mitochondrial membrane potential. On the other hand, extrinsic, caspase-dependent apoptosis created by up-regulating the levels of cleaved caspase-8 and cleaved caspase-3 are induced through this compound treatment (20, 40, and 80 μM) (Seo et al., 2015a). Chen et al., studied the effects of apigenin (0–160 μM) on non-small cell lung cancer (NSCLC) cells and pointed out that in a p53-dependent manner, the levels of death receptor 4 (DR4) and death receptor 5 (DR5) were up-regulated. Thus, sensitizing NSCLC cells to TRAIL-induced apoptosis. Furthermore, it was revealed that exposing lung cancer cells to apigenin (0–160 μM) induces apoptosis via the up-regulation of pro-apoptotic factors Bad and Bax, and the down-regulation of anti-apoptotic factors Bcl-xl and Bcl-2 (Chen et al., 2016). In addition, a good example of the role of apigenin in both intrinsic and extrinsic apoptosis pathways is observed in human keratinocytes and organotypic keratinocytes, which increases UVB-induced apoptosis through both pathways. Bax localization and cytochrome c release were altered by apigenin (0, 10, and 20 μmol/L). Overexpression of the pro-survival protein Bcl-2 and the dominant-negative form of Fas-associated death domain protected against apigenin-induced apoptosis (Figure 4) (Abu-Yousif et al., 2008).
Autophagy is a process known as type 2 non-apoptotic cell death (Hazari et al., 2020). The sequestration of cytoplasmic material into vacuoles for bulk degradation by lysosomal enzymes is the feature of this regulated mechanism (Galluzzi and Green, 2019). In other words, a cell digests its own cytoplasmic materials within lysosomes and results in the decomposition of macromolecules through this conserved dynamic process (Korolchuk and Rubinsztein, 2011; Chaabane et al., 2013; Yang and Klionsky, 2020). To date, there is a growing body of evidence to suggest that the association between autophagy and cancer is complicated and contradictory (Wen and Klionsky, 2019; Galluzzi and Kroemer, 2020). Autophagy performs various functions in the body; during starvation, this mechanism serves as a cell survival pathway by preparing recycled metabolic substrates as well as keeping energy homeostasis (Sameiyan et al., 2019). Besides, it results in cell death, in association with an apoptosis pathway or as a backup mechanism (Nazim et al., 2020). Apigenin-induced autophagy was first detected in erythroleukemia TF1 cells. Apigenin exposure (0–200 μM) leads to the onset of autophagy lacking apoptosis (Ruela-de-Sousa et al., 2010). Since then, more evidence demonstrated that autophagy could be triggered by apigenin and also under diverse conditions, it acts as tumor suppressive or tumor protective (Sung et al., 2016; Salmani et al., 2017).
Investigating the impact of apigenin (20 μM) on human keratinocytes, Tong et al. reported that autophagy was induced through AMPK activation by this chemo-preventive bioflavonoid (Tong et al., 2012). Cao et al. performed a similar series of experiments to show that in human breast cancer T47D and MDA-MB-231 cells treated with apigenin (0–80 μM), both apoptosis and autophagy pathways were triggered through the accumulation of acidic vesicular organelles (AVOs) and LC3-II, a marker of Atg5/Atg7 dependent autophagy. In addition, further studies have revealed that apigenin-induced apoptosis is significantly enhanced during treatment with the 3-methyladenine (MA) autophagy inhibitor. It shows that autophagy triggered by apigenin performs a tumor protective role in apigenin-caused cytotoxicity (Cao et al., 2013). Similarly, Lee et al. demonstrated that in human colon cancer HCT116 cells, apigenin (0–50 μM) simultaneously induces both apoptosis as well as autophagy. Autophagy played a cell protective role in apigenin-induced cell apoptosis as well (Lee et al., 2014).
Beclin-1 is able to regulate the dynamic autophagy procedure through the formation of autophagosomes (Liang et al., 2019; Vega-Rubín-de-Celis, 2019). In various kinds of cancers including solid Ehrlich carcinoma, Beclin-1 is regularly down-regulated. Gaballah et al. published a paper in which they described that combining 5-FU with apigenin (100 mg/kg/day) pointedly improved Beclin-1 compared to the vehicle-treated control mice (Gaballah et al., 2017). Furthermore, according to the study of Wang et al. autophagy is induced in macrophages during apigenin treatment (10, 25, and 50 μM), as evidenced by the further regulation of Beclin-1, Atg5, Atg7, and the presence of LC3-II. Further, based on experiments, inhibition of autophagy by 3-MA pretreatment remarkably boosted apigenin-induced apoptosis. Also, signifying that the autophagy caused by apigenin protected macrophages from apigenin-induced cytotoxicity (Wang et al., 2015).
In contrast, through investigations into human papillary thyroid carcinoma BCPAP cells, it was discovered that treatment with apigenin (12.5, 25, and 50 μM) leads to autophagic cell death following p62 degradation, Beclin-1 accretion as well as LC3 protein conversion. Interestingly, additional examination demonstrated that apigenin-induced cytotoxicity was significantly protected via co-treatment with 3-MA, which indicated that apigenin-induced autophagy here is more likely to be a tumor suppressor (Zhang et al., 2015). Together, according to cancer cell types, autophagy has a diverse role in apigenin-induced cytotoxicity.
In most reports, the function of apigenin-triggered autophagy is to mediate the acquired resistance of cancer cells versus cell apoptosis, evidenced as improved cell apoptosis encouraged by apigenin when in cotreatment with autophagy inhibitors. Under this circumstance, the autophagy performs cytoprotective tasks in apigenin-induced cytotoxicity in cancer cells. In contrast, in human papillary thyroid carcinoma BCPAP cells, autophagy operates as an executioner through encouraging autophagic cell death (Zhang et al., 2015).
As it was mentioned, apigenin is capable of inducing both autophagy and apoptosis, as major arms of programmed cell death (PCD). However, apigenin-mediated autophagy results in the enhanced survival of cancer cells, and their resistance into chemotherapy. This is due to the dual role of autophagy in cancer cells. Increasing evidence demonstrates that autophagy works like a double-edged sword in cancer cells, and it may function as a pro-survival or pro-death mechanism (Huang et al., 2019b; Wang et al., 2019b). In the case of apigenin, autophagy induction is correlated with an increase in the survival of cancer cells, and its down-regulation by autophagy inhibitors can pave the road to effective cancer therapy.
Apigenin and Cancer Metastasis
Tumors are divided into benign and malignant types based on a number of criteria including location and growth characteristics, and tissue origin. Lack of migration is a prominent feature of benign tumor cells. Unlike malignant tumor cells which are highly unstable and capable of metastasizing and attacking other tissues to cause more lesions, benign tumor cells grow only at the primary site of the tumor and cause lesions and can be eliminated through clinical surgery. Most patients die with varying grades of tumor metastasis, not in the clinical practice of the primary disease. Currently, metastases, accompanied by chemoresistance development as well as tumor recurrence, are still key obstructions in operative cancer treatment (Murugan, 2019; Zhang, 2019; Zhuang et al., 2019). It was explored that for in vitro cancer cells and in vivo animal models, apigenin can suppress cancer cell migration and invasion (Chien et al., 2019; Lee et al., 2019a; Tong et al., 2019).
Plant derived-natural compounds are able to target molecular signaling pathways involved in cancer growth and metastasis (Zhang et al., 2019; Liao et al., 2020). Accumulating data exhibit that the PI3K/Akt signaling pathway plays a significant role in cancer growth and metastasis. Inhibition of the PI3K/Akt signaling pathway inhibits the malignant behavior of cancer cells, and restricts their migration (Huang et al., 2019a; Zheng et al., 2019). Tumor cell invasion and migration in a dose-dependent manner are repressed via apigenin (0-20 μM) in prostate cancer DU145 cells (Zhu et al., 2015). Concerning the effect of apigenin on A375 and C8161 melanoma cell lines, it was found that 40 μM of this compound remarkably prevented cell migration and invasion through impacting the AKT/mTOR pathway (Zhao et al., 2017a). Also, an experiment of apigenin treatment (0–40 μM) in a human A549 lung cancer cell line revealed that this compound was able to arrest Akt phosphorylation and target the PI3K/AKT signaling pathway, leading to anti-migration and anti-invasion effects (Zhou et al., 2017). In a study conducted by Dai et al., it was shown that apigenin (0–50 μM) could inhibit cell migration, invasion, and metastasis through regulating the NEDD9/Src/AKT cascade in the colorectal cancer cell lines DLD1 and SW480 (Dai et al., 2016).
Moreover, in vitro study has shown that apigenin lowered the migration and invasion of cancer cells by decreasing FAK expression in human ovarian cancer A2780 cells. Also, further experiments have revealed the spontaneous metastasis suppression of A2780 cells implanted into the ovary of nude mice in vivo by apigenin treatment (0–40 μM) (Hu et al., 2008). Additionally, cell proliferation and migration were prohibited through apigenin exposure by up-regulating and down-regulating transgelin and MMP-9 expression, respectively via decreasing the phosphorylation of Akt. Therefore, tumor growth and metastasis to the liver and lung were repressed by apigenin treatment (Figure 5) (Lieben, 2017).
Apigenin-Loaded Nanovehicles
Overcoming poor bioavailability and low solubility of apigenin requires using carriers for targeted delivery of apigenin that remarkably enhances the anti-tumor activity of apigenin (Mahmoudi et al., 2019). Fortunately, different nanocarriers were designed for the delivery of apigenin and for reducing the survival and viability of cancer cells. On the other hand, as mentioned before, resistance of cancer cells to chemotherapy is an increasing challenge in the field of cancer therapy. Hence, using carriers for protecting and delivering of biotherapeutic agents not only ameliorates its anti-tumor activity but also sensitizes cancer cells to the inhibitory effects of chemotherapeutic agents (Mahmoudi et al., 2019; Jamaledin et al., 2020).
In light of this, several sorts of nanosized vehicles were exploited to encapsulate and liberate apigenin at the targeted site. Liposomes are ideal candidates for delivery of anti-tumor drugs with satisfactory results at preclinical experiments. Since liposomes contain a hydrophilic core and a hydrophobic bilayer, this nanocarrier can be applied for the delivery of both hydrophobic and hydrophilic drugs (Mickova et al., 2012; Sen and Mandal, 2013). In respect to the capability of liposomes in encapsulating several drugs, they can significantly improve the therapeutic effects of their cargo (Hu and Zhang, 2012; Gowda et al., 2013; Sen et al., 2014). It is noteworthy that liposomes were used for the co-delivery of apigenin and 5-fluorouracil in colorectal cancer therapy. Using liposomes is correlated with an increase in the cytotoxicity of apigenin and 5-fluorouracil against colorectal cancer cells (Sen et al., 2019). Apigenin- and 5-fluorouracil-loaded liposomes are able to efficiently inhibit angiogenesis and induce apoptosis in colorectal cancer cells, leading to a decrease in the proliferation and viability of colorectal cancer cells. It is worth mentioning that using liposomes for the delivery of apigenin and 5-fluorouracil significantly promotes the capability of these anti-tumor agents in the up-regulation of AMPK, and the inhibition of the Warbrug effect (Sen et al., 2019). The study demonstrates that such nanocarriers enhance the anti-tumor activity of apigenin and the efficacy of chemotherapy; in which, it exerts a more inhibitory effect on the proliferation of cancer cells by the induction of apoptosis, and by disrupting glycolysis metabolism, and promoting the efficacy of targeting molecular pathways. Apigenin-loaded liposomes have demonstrated great potential in disrupting the cell membrane of cancer cells which is an underlying mechanism for stimulation of cell cycle arrest at the G2/M phase (Banerjee et al., 2017). In addition to colorectal cancer, apigenin/tyroservatide-loaded liposomes were applied for lung cancer therapy. It was shown that these delivery systems are able to remarkably stimulate apoptosis and cell cycle arrest at the G2 phase in A549 cells (Figure 6) (Jin et al., 2017). This is due to providing targeted delivery and enhancing the accumulation of apigenin in cancer cells.
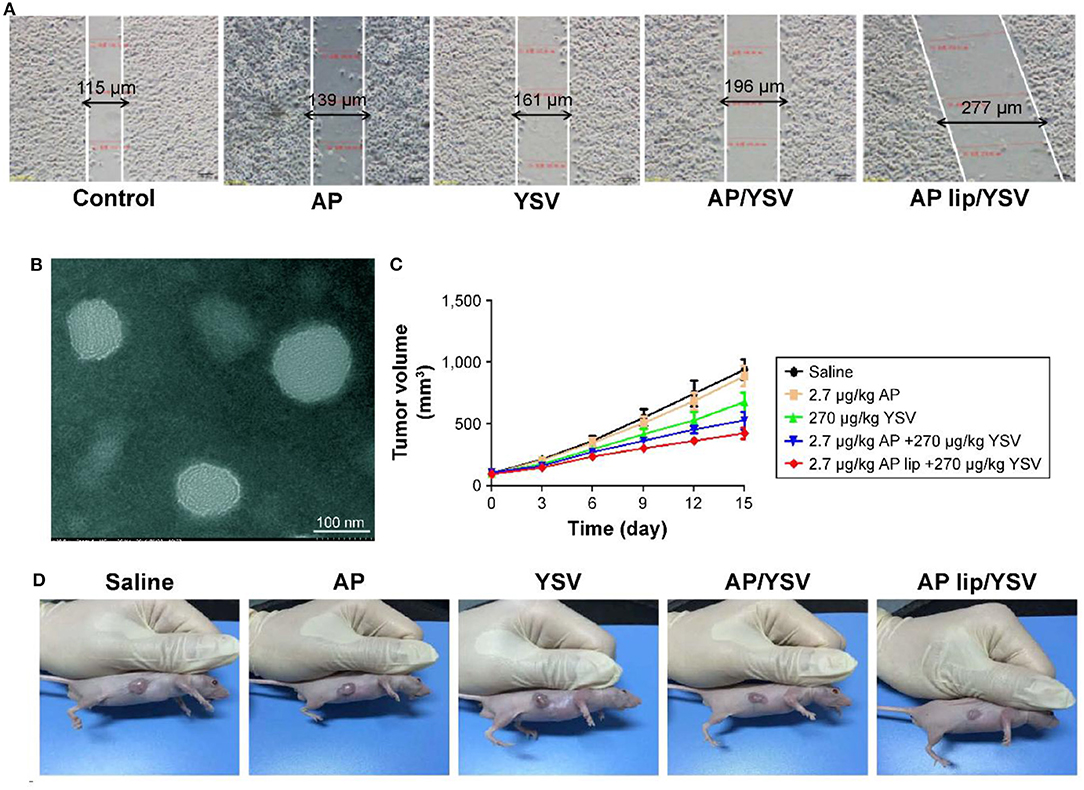
Figure 6. (A) Synergistic effects of apigenin-loaded TPGS liposomes and tyroservatide (YSV) in A549 cells. (B) Transmission electron microscopy (TEM) image of apigenin-loaded D-alpha-tocopheryl polyethylene glycol (TPGS) liposomes. Diagram of tumor volumes (C) and morphology (D) after 15 days. Reprinted with permission from Jin et al. (2017).
Apigenin-loaded nanoparticles were applied in the treatment of hepatocellular carcinoma that resulted in enhanced cytotoxicity of apigenin against cancer cells. This is due to increased availability of apigenin in blood circulation and accumulation in the liver (Bhattacharya et al., 2018). Interestingly, nanocarriers enhanced cytotoxicity of apigenin against cancer cells without affecting normal cells (high biocompatibility) (Jangdey et al., 2019).
Surface functionalization of nanoparticles significantly enhances their capability to target cancer cells. Surface modification can be directed toward targeting special receptors undergoing overexpression in cancer cells. For instance, a cluster of the differentiation protein CD44 shows high expression in cancer cells, and sodium hyaluronate can be used for surface modification of nanoparticles, and targeting CD44 in cancer cells (Dosio et al., 2016). Such a strategy was employed for the delivery of apigenin. It was reported that sodium hyaluronate nano-assemblies are capable of enhancing the accumulation of apigenin in lung cancer cells via targeting CD44 and providing receptor-mediated endocytosis (Zhao et al., 2017b). The surface decoration of nanocarriers using hyaluronic acid (HA) offers the possibility of targeting CD44 receptors. On the other hand, HA functionalization of nanovehicles can indeed change physicochemical properties, alter stability, toxicity, and influence nanoparticle biodistribution and efficiency in vitro and in vivo (Wang et al., 2016; Xu et al., 2017; Alves et al., 2019). In light of this, HA decorated-lipid nanoparticles were employed for the delivery of anti-tumor therapeutics (Figure 7) (Mahmoudi et al., 2019). The finding showed that co-delivery of apigenin and docetaxel impose synergistic anti-cancer effects toward A549 cells. In addition, surface coating lipid nanocarriers with hyaluronic acid enhanced the cellular uptake in comparison with pristine lipid NPs (Mahmoudi et al., 2019). Other carrier systems based on HA (e.g., chemically crosslinked hydrogel nanocomposites or in situ gel at body temperatures, known as thermosensitive hydrogel) are other valuable options to deliver biotherapeutics to the human body (Kim et al., 2018; Makvandi et al., 2019, 2020; Tan et al., 2019).
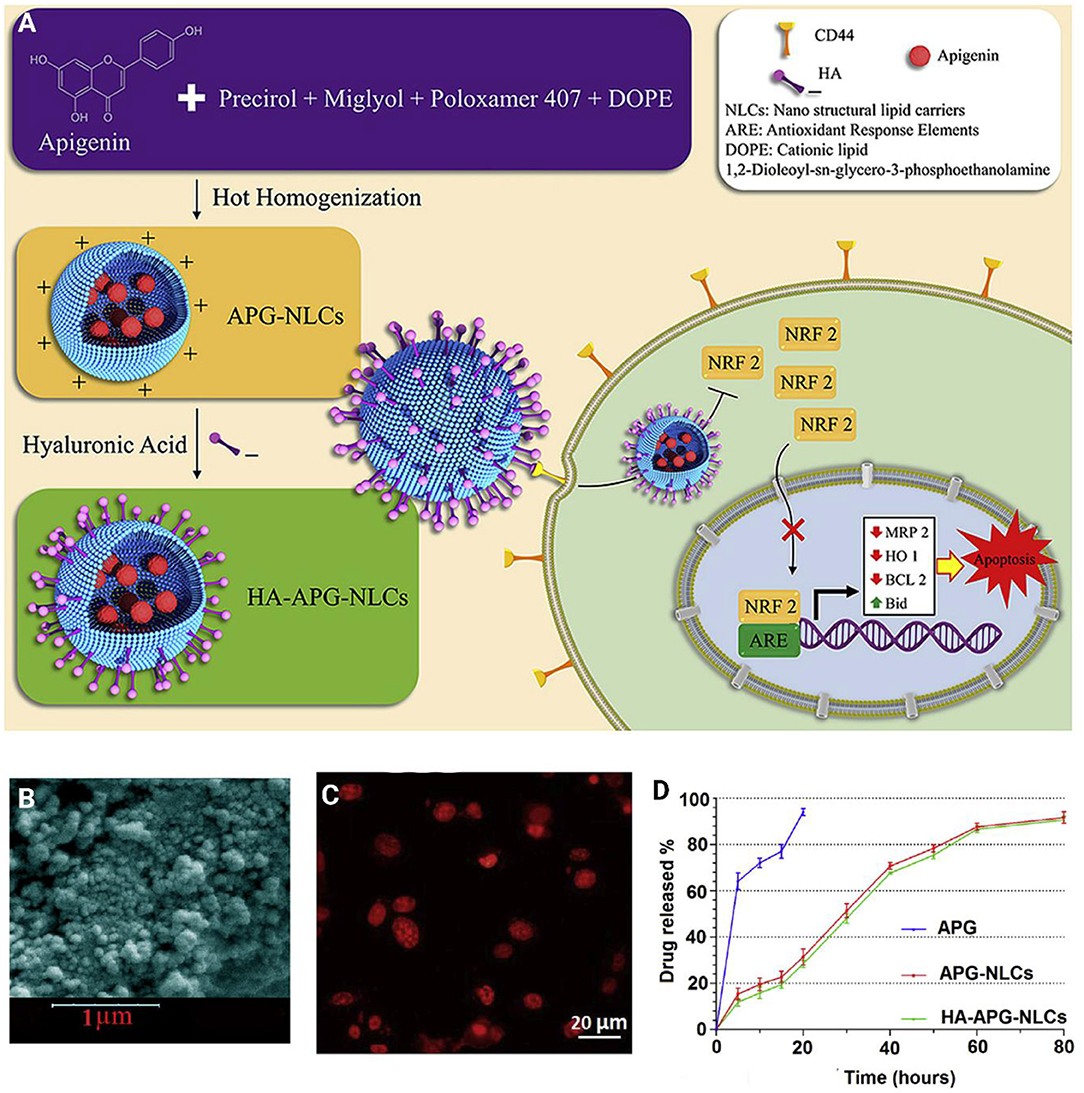
Figure 7. (A) Schematic illustration of hyaluronic acid-functionalized nanostructured lipid carriers (HA-NLCs) containing apigenin. (B) SEM images HA-NLCs. (C) In vitro internalization of Rhodamine B encapsulated apigenin-nanostructured lipid carriers. (D) In vitro drug release of apigenin solution (APG), APG encapsulated-NLCs, and HA-NLCs. Reprinted with permission from Mahmoudi et al. (2019).
Taking these findings into account, nanocarriers are promising candidates in the delivery of apigenin for cancer therapy. They are capable of exponentially improving the cytotoxicity of apigenin toward tumor cells. Besides, they promote bioavailability and cellular uptake of apigenin, leading to an increase in the therapeutic efficacy of apigenin (Das et al., 2013; Jangdey et al., 2017).
Apigenin and Pancreatic Cancer Therapy
Dietary cancer prevention and chemoprevention are two main diet-related prevention strategies in cancer studies (Singletary, 2000). Cancer chemoprevention uses synthetic, natural, or biologic chemicals in the pharmacologic intervention struggling against carcinogenesis (Tsao et al., 2004). However, dietary cancer prevention is defined as a modified pattern of food consumption along with lifestyle alteration that reduces cancer risk (Schatzkin and Kelloff, 1995; Singletary, 2000). Recently, food-based strategies for pancreatic cancer prevention have been studied using epidemiological evidence. These studies demonstrated an inverse relationship between vegetable and fruit consumption and the risk of pancreatic cancer development (Larsson et al., 2006; Polesel et al., 2010).
Apigenin is a flavone usually found in vegetables and fruit such as citrus (e.g., grapefruit) (Cirmi et al., 2016). The fruits and vegetables enriched in apigenin have shown an anti-cancer effect. The outcomes are primarily attributed to their anti-inflammatory and antioxidants effects (Madunić, Madunić et al., 2018). Apigenin has free radical scavenging and anti-inflammatory characteristics. It is also considered as an anti-cancer agent, reducing the proliferation of cancer cells, while having no effects on normal cells (Cirmi et al., 2016). Apigenin has shown growth inhibitory properties in breast cancer via apoptosis promotion via (a) activation of the caspase cascade; (b) blocking NF-κB and STAT3 signaling in breast cancer cells with HER2-overexpression; (c) eliminating both the PI3K and Akt kinase activity and modulation of the p14ARF-Mdm2-p53 pathway (Way et al., 2004; Agrawal et al., 2006; Choi and Kim, 2009; Seo et al., 2012). We have summarized anti-pancreatic cancer effects of apigenin and its glycosides below.
Molecular Pathways and Mechanisms
Apigenin, due to the stimulation of the production of reactive oxygen species (ROS) (Shukla and Gupta, 2008) in solid and hematological cancers, has shown some anti-cancer properties (Granato et al., 2017). By way of illustration, one of the most common strategies which is applied in both conventional and non-conventional radio- and chemo-therapies is stimulating the ROS generation with the aim of killing the cancer cells (Yang et al., 2018). Several factors are involved in balancing the level of the ROS in cells. NADPH-oxidases and mitochondrial complexes which produce ROS, enzymes which have intervener roles in antioxidant responses, e.g., superoxide dismutase (SOD) and catalase, and enzymes involved in detoxifying of ROS such as glutathione S-transferase (GST) are some well-known examples of these factors (Kumari et al., 2018). The regulation in the expression level of the anti-oxidant enzymes is mostly controlled by the transcription nuclear factor erythroid 2 like2 (NRF2) (Chatterjee et al., 2013). Therefore, redox resetting for surviving tumor cells in anticancer drug treatment conditions is usually induced by the tumor cells themselves (Liu et al., 2016). NRF2-induced pathways have important roles in the chemotherapeutic resistance of cancer cells (Rojo de la Vega et al., 2018).
Tumor suppressor P53 is a prominent factor in responses to anti-tumor therapies. The functional p53 pathway prevents tumor development and growth; therefore, the p53 gene is mutated in most of the tumors (Mantovani et al., 2019). The “gain of function” mutation which prompt tumor cells to grow, metastasize, and resist therapies is one of the most common mutations in P53 genes (Bellazzo et al., 2018). Tp53 mutations (mutp53) are very common in PDAC (Muller and Vousden, 2014). Mutp53 proteins usually would not become degraded due to obtaining a mis-folded conformation; therefore, hyper-stable proteins may accumulate in tumors (Alexandrova et al., 2015). The most well-known factors affording mutp53 stability are cellular chaperone heat shock proteins (HSP70 and HSP90) which bind to mutp53 and prevent the degradation of this protein with a double minute 2 homolog (MDM2) protein. The HSP90 expression level is increased in cancer cells in order to assist in cancer cell survival; hence, HSP90 may be a potential target in cancer therapy (Solarova et al., 2015). The heat shock factor 1 (HSF1) is the most predominant factor which controls the heat shock response. HSF1 by contacting mutp53 may enhance the HSF1-induced transcription program, which up-regulates heat shock proteins (HSPs) in a positive feed-forward loop that results in more maintenance of mutp53 (Li et al., 2014). There may be an interaction between NRF2 and HSF1 in which HSP and P62 are their mutual targets (Dayalan Naidu et al., 2015). Furthermore, mutp53 may cooperate with the NRF2 with the aim of moderating the NRF2-mediated anti-oxidant response (Lisek et al., 2018). It was shown that NRF2 induced pancreatic carcinogenesis in mice with k-ras mutations and mutp53 (Hamada et al., 2017).
Above all mutp53 is an essential target for anticancer therapy. Accordingly, several mutp53 approaches were examined in recent years such as applying strategies to mutp53 degradation and/or repetition of the wild-type p53 (mutp53 as a dominant negative effect prevents wild-type p53) (Schulz-Heddergott and Moll, 2018). One possible strategy is to inhibit mutp53 by autophagy (Garufi et al., 2014, 2015). Another one is to use the zinc effect as a modifier in the mutp53 protein conformation, or through natural compound capsaicin (Garufi et al., 2016). Mutp53 despite the wtp53 suppresses autophagy which in turn inhibits mutp53 degradation (Cordani et al., 2016). Realizing the interaction between mutp53 and autophagy is essential for an effective anticancer therapy. The effect of apigenin in the cytotoxicity of PaCa44 and Panc1 cancer cells with various p53 mutations was assessed (Moore et al., 2001). As confirmed by an experiment on the apigenin effect on the toxicity of two pancreatic cell lines, PaCa44 and Panc1, which harbor various p53 mutations, by Montani et al. (Gilardini Montani et al., 2019). It was shown that the cytotoxic effect of apigenin on the Panc1 cell line is higher in comparison to its effect on PaCa44 cell lines. It was shown that the stronger cytotoxic effect of apigenin (6, 12.5, 25, and 50 μM) is due to a decrease in HSP90 and mutp53 expression, higher amounts of intracellular ROS, and mTORC1 suppression. In this study, it was recommend that targeting mTOR-mutp53-NRF2-p62-HSP90 molecules may be useful for overcoming the chemo-resistance of PC to apigenin (Gilardini Montani et al., 2019).
It was shown that up-regulation of CK2 results in the development of lymphomas and leukemia (Landesman-Bollag et al., 1998; Channavajhala and Seldin, 2002; Seldin et al., 2008). CK2-dependent signaling pathways are inhibited by apigenin. Several biological activities were attributed to apigenin such as anti-oxidant, anti-carcinogenic, anti-proliferative, and anti-inflammatory activities (Patel et al., 2007). Recently, several experiments focused on applying apigenin as a chemo-preventive agent in various cancers (Mafuvadze et al., 2012). Apigenin may lead to changes in regulatory T cells and effector T cells growth of murine PC (Nelson et al., 2015).
From a dysregulation which was shown between phosphatase 1 (PP1) and Casein Kinase II (CK2), it was suggested that higher CK2 expression controls the stability of the Ikaros. Furthermore, it was shown that down-regulation of the Ikaros leads to a reduction in CD4+ and CD8+ T cell percentages but augmented CD4+CD25+ Tregs in tumor-bearing (TB) mice (Nelson et al., 2017).
In a study, naïve mice splenocytes were treated with apigenin in vitro when Panc02 cells were present which lead to an evaluation of the Ikaros expression, like the activity of the proteasome inhibitor MG132. TB mice cells were treated with apigenin. In vivo results showed a decrease in tumor size and an inhibition of the splenomegaly. Furthermore, apigenin (10 and 20 μM) treatment leads to the reestablishment in production of a few Ikaros isoforms, which are probably responsible for the mild prevention of the CDK2 function in splenocytes of the TB-apigenin mice. Complementary to the incomplete reestablishment of the Ikaros expression, the percentage of the CD4+ and CD8+ T cells have shown a considerable growth and the percentage of Tregs have fallen into a significant decline in TB-apigenin mice. Moreover, CD8+ T cells from TB-apigenin mice compared to TB mice have shown additional production of the IFN-γ and splenocytes of the TB-apigenin mice are more susceptible to allogeneic CD8+ T cell responses. These findings offer more support for the idea that Ikaros in a pancreatic cancer model is controlled by CK2, and these results have shown that apigenin is a potential therapy for murine PC (Nelson et al., 2017).
Recently it was shown that hypoxia-inducible factor-1 α (HIF-1α) is targeted by apigenin in several cancers such as, ovarian cancer, prostate cancer, and lung cancer (Osada et al., 2004; Liu et al., 2005). HIF-1α is a prominent transcription factor for the transcription of the genes which are responsible for tumor development and invasion under hypoxic conditions (Melillo, 2006). An increase in the expression level of HIF-1α is revealed in several cancers which is a contributory factor for drug resistance and higher mortality (Birner et al., 2001; Koukourakis et al., 2006).
Hypoxia stimulates some changes in cellular metabolism, resistance to apoptosis, and induces angiogenesis in PC cells lines (Garcea et al., 2006). Pancreatic carcinomas compared to benign tumors show higher levels of the expression of the HIF-1α protein, more tumor proliferation, and less tumor differentiation (Mabjeesh and Amir, 2007).
The HIF is a protein consisting of two αβ heterodimers. The HIF-1α expression is stimulated by hypoxic conditions. The HIF-1β is constantly expressed (Garcea et al., 2006). Interaction of the HIF-1 αβ heterodimer with the hypoxia response element (HERs) leads to the stimulation of transcriptional activity. In physiological conditions, HIF prolyl hydroxylases hydroxylated the two prolyl residues of the HIF-1α protein and subsequently interaction between this protein and the von Hippel-Lindau (VHL) E3 ubiquitin ligase complex leads to proteasomal destruction of HIF-1α (Ivan et al., 2001). On the other hand, under hypoxic conditions, as the HIF prolyl hydroxylases are inactivated, the HIF-1α, which is accumulated, dimerized with HIF-1β which leads to hypoxic response gene transcription (Garcea et al., 2006).
As a result of the increase in the expression level of the HIF-1α gene in pancreatic cancer, several down-stream genes are subsequently transcriptionally activated. Production of these down-stream genes are necessary for angiogenesis, for example, the vascular endothelial growth factor (VEGF) and also, for glycolysis, the GLUT-1 glucose transporter (Lin et al., 2016; Zambrano et al., 2019).
VEGF as a secreted protein is an inducer for tumor vessel growth. The poor survival of patients with PC, low survival after the surgery, and subsequently hepatic metastasis are directly proportional to the serum level of the VEGF (Karayiannakis et al., 2003). PC cells which were treated by VEGF show more development and knock-out of the VEGF in pancreatic tumors of animal models leads to a decrease in vascularity and growth (Inoue et al., 2002).
The up-regulation of the glucose transporter (GLUT-1) is directly proportional to the poor prognosis in several cancers including ovarian, gastric, breast, and colorectal carcinomas (Zambrano et al., 2019). Gene expression of the GLUT-1 is correlated to the cancer metastasis in PC (Ito et al., 2004). The Warburg effect which means augmented glucose consumption in cancer cells was shown in solid tumors such as pancreatic cancers (Mueckler, 1994). HIF-1α induces the glucose transporter GLUT-1 expression under hypoxic conditions (Chen et al., 2001). The correlation between HIF-1α and GLUT-1 in inducing cancer development suggests GLUT-1 as a potential cancer therapy. It was revealed that glucose transporter GLUT-1 is blocked by apigenin (0–100 μM) under normoxic conditions (Melstrom et al., 2008).
In one study, the effect of apigenin on hypoxia responsive genes in pancreatic cancer was performed by Melstrom et al. (153). The expression level of the VEGF, HIF-1α, and GLUT-1 was examined in S2-013 human PC cells and CD18 cells which were treated with apigenin (0–50 μM) by an enzyme-linked immunosorbent assay (ELISA), Western blot analysis, and real-time RT-PCR in both normoxic and hypoxic conditions. GLUT-1 expression undergoes up-regulation in PC cells in comparison to adjacent controls (P < 0.001). Expression of the VEGF, HIF-1α, and GLUT-1 protein is stimulated in S2-013 PC cells and CD18+ cells under hypoxic conditions. Hypoxia-induced up-regulation of these three proteins is inhibited by apigenin (50 μM). Furthermore, apigenin obstructed the expression of the GLUT-1 and VEGF mRNA under hypoxia conditions in the mentioned cell lines. In normoxic and hypoxic conditions, GLUT-1, HIF-1α, and VEGF mRNA transcription and protein production both are suppressed by apigenin. This suggests apigenin as a potential anti-cancer drug for the treatment of the PC (Melstrom et al., 2011). Table 2 illustrates the therapeutic effects of apigenin on PC cells.
Chemotherapy
Although a previous study offers an interesting strategy to inhibit the resistance of PC cells with apigenin, increasing evidence demonstrates that apigenin can be applied as a chemosensitizer. Naturally occurring dietary compounds were highlighted due to their positive effects on overcoming the resistance of tumor cells to apoptosis (Johnson and de Mejia, 2011). The natural flavonoid apigenin is a potential molecule for overcoming chemoresistance in pancreatic cancer (Johnson and de Mejia, 2013). Serine/threonine kinase glycogen synthase kinase-3β (GSK-3β) as a potential target for flavonoids is responsible for major upstream regulator of the NF-κB transcriptional activity. NF-κB enters the nuclei of the pancreatic cancer cells (Ougolkov et al., 2005). Conventional chemotherapeutic agents refuse apoptosis by stimulating the NF-κB pathway (Long et al., 2011). GSK-3β activity is inhibited by apigenin (Johnson et al., 2011). Furthermore, this flavonoid has an important role in suppressing pancreatic cancer cell proliferation in vitro. Apigenin (0, 10, 25, and 50 μM) activates apoptosis in pancreatic cancer cells by suppressing the GSK-3β/NF-κB signaling pathway (Figure 8) (Johnson and de Mejia, 2013).
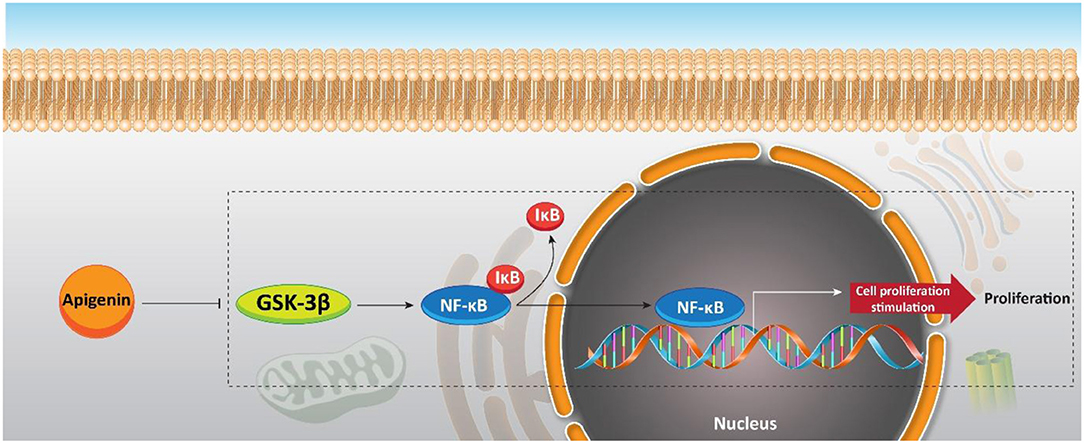
Figure 8. Effect of apigenin on NF-κB signaling, and its upstream mediator GSK-3β in sensitizing pancreatic cancer cells to chemotherapy.
According to the experiment conducted by Johnson et al. the ability of the flavonoid apigenin to assist chemotherapeutic drugs in inhibiting the proliferation of BxPC-3 pancreatic cells was examined (Johnson and Gonzalez de Mejia, 2013). An MTS cell proliferation assay with different concentrations of the chemotherapeutic drugs (0–50 μM) and concurrent pretreatment or treatment of flavonoids (0, 6, 24, and 42 h) were conducted. Simultaneous treatment through the chemotherapeutic drugs 5-fluorouracil (5-FU, 50 μM), flavonoid (13, 25, or 50 μM), or gemcitabine (Gem, 10 μM) for 60 h leads to mostly less-than-additive effects (p < 0.05). Pretreatment for 24 h with 13 μM of apigenin, followed by Gem for 36 h was ideal for suppressing the proliferation of the cells. Pretreatment of cells with 11–19 μM of apigenin for 24 h leads to 59–73% growth suppression when followed by Gem (10 μM, 36 h). Pretreatment of BxPC-3 human pancreatic cancer cells by low concentrations of apigenin or Lut assist chemotherapeutic drugs in their anti-proliferative properties (Figure 9) (Johnson and Gonzalez de Mejia, 2013).
Conclusion and Outlook
Since, there have not yet been any reports of apigenin having unfavorable metabolic reactions, involving it in the diet is recommended. Bioactive compounds such as apigenin take part in different metabolic pathways to exert their healing effects. The pharmacokinetic behavior of these bioactive compounds affects their bioactivity as well as tissue distribution. Naturally, apigenin occurs in dimeric forms linked through C–C or C–O–C bonds.
Various pharmacokinetic behaviors and healing effects have been found in flavonoid aglycones and their glycosides. So, C-glycosylation or O-glycosylation of apigenin may have effects on its metabolism, and in turn, show impacts on both its anti-oxidant potential and biological benefits. The decreased anti-oxidant potential of apigenin via O-glycosylation has been demonstrated in an in vitro assay (Cai et al., 2006). In another study, Angelino et al. investigated the bioavailability of the apigenin-C-glycosides. They reported no alteration in the absorption of vitexin-2-O-xyloside (VOX) that is an apigenin-8-C-glucoside in a rat model (Angelino et al., 2013). Besides being hydrolyzed to the mono-glycoside, reduced, and conjugated to make a bioavailable glucuronide, VOX also undertakes enterohepatic recirculation. Over the last few years, many studies pointed out the various pharmacological activities and nutraceutical potential of apigenin. For instance, its anti-oxidant properties are well-recognized and apigenin is also considered as a therapeutic agent for different conditions such as autoimmune disease, neurodegenerative disease, inflammation as well as some types of cancers (Salehi et al., 2019). In suppressing the proliferation of cancer cells, apigenin can induce apoptotic cell death via increasing ROS generation, the down-regulation of anti-apoptotic factors Bcl-2 and Bcl-xl as well as the up-regulation of apoptotic factors Bax and Bim. Besides, apigenin can induce cell cycle arrest at the G2/M and S phases. In suppressing metastasis of cancer cells, apigenin administration interferes with the PI3K/Akt/mTOR signaling pathway as well as the expression of MMP-9, as a factor involved in the progression and invasion of cancer cells.
In comparison to the other structurally related flavonoids, apigenin showed reduced intrinsic toxicity on normal cells. Despite its importance and useful effects, there is not enough literature on apigenin's beneficial health potential for humans. A good reason may be low solubility of apigenin in water (1.35 μg/mL) and its high permeability (Zhang et al., 2012). These may hamper the in vivo studies into apigenin. There are various strategies suggested to increase solubility, such as several delivery systems (nanosuspension, polymeric micelles, liposomes). These approaches, for example, show how solid dispersion could improve the low solubility of therapeutic agents. Furthermore, several injectable nanosized drug delivery systems have been devised, demonstrating that nanocapsules may be a good tactic to lengthen the pharmacological activity of apigenin. Indeed, high metabolic transformation and low bioavailability of some food components have been left as unsolved issues.
It was revealed that the administration of apigenin is beneficial in enhancing the sensitivity of PC cells to chemotherapy. Besides, apigenin affects molecular pathways such as HIF, GLUT-1, and VEGF to disrupt the proliferation and malignant behavior of PC cells. Also, additional molecular pathways and mechanisms (e.g., Wnt, miRs, lncRNAs, epithelial-to-mesenchymal transition) can be considered as down-stream targets of apigenin, and can be discussed in future studies. According to the information presented in clinicaltrials.gov, today just one study has examined the anti-tumor activity of apigenin in clinical trials. Its status is suspended (NCT00609310) and it was supposed to evaluate effect of apigenin on the recurrence of colorectal cancer cells. However, we are still at the beginning stage and there will be more studies in the future to investigate the anti-tumor activity of apigenin in clinical trials.
Author Contributions
HM was involved in the conception, design, statistical analysis, drafting of the manuscript, and supervised the study. MB, MA, ZB, KI, AZ, PM, HK, MD, and SM contributed to data collection and manuscript drafting. All authors approved the final version for submission.
Funding
The present study was funded by a grant from the Vice Chancellor for Research, Kashan University of Medical Sciences, in Iran.
Conflict of Interest
The authors declare that the research was conducted in the absence of any commercial or financial relationships that could be construed as a potential conflict of interest.
References
Abotaleb, M., Liskova, A., Kubatka, P., and Büsselberg, D. (2020). Therapeutic potential of plant phenolic acids in the treatment of cancer. Biomolecules 10:221. doi: 10.3390/biom10020221
Abu-Yousif, A. O., Smith, K. A., Getsios, S., Green, K. J., Van Dross, R. T., and Pelling, J. C. (2008). Enhancement of UVB-induced apoptosis by apigenin in human keratinocytes and organotypic keratinocyte cultures. Cancer Res. 68, 3057–3065. doi: 10.1158/0008-5472.CAN-07-2763
Aggarwal, V., Tuli, H. S., Tania, M., Srivastava, S., Ritzer, E. E., Pandey, A., et al. (2020). Molecular mechanisms of action of epigallocatechin gallate in cancer: recent trends and advancement. Semin. Cancer Biol. doi: 10.1016/j.semcancer.2020.05.011. [Epub ahead of print].
Agrawal, A., Yang, J., Murphy, R. F., and Agrawal, D. K. (2006). Regulation of the p14ARF-Mdm2-p53 pathway: an overview in breast cancer. Exp. Mol. Pathol. 81, 115–122. doi: 10.1016/j.yexmp.2006.07.001
Alexandrova, E. M., Yallowitz, A. R., Li, D., Xu, S., Schulz, R., Proia, D. A., et al. (2015). Improving survival by exploiting tumour dependence on stabilized mutant p53 for treatment. Nature 523, 352–356. doi: 10.1038/nature14430
Alshehri, S. M., Shakeel, F., Ibrahim, M. A., Elzayat, E. M., Altamimi, M., Mohsin, K., et al. (2019). Dissolution and bioavailability improvement of bioactive apigenin using solid dispersions prepared by different techniques. Saudi Pharm. J. 27, 264–273. doi: 10.1016/j.jsps.2018.11.008
Alves, C. G., de Melo-Diogo, D., Lima-Sousa, R., Costa, E. C., and Correia, I. J. (2019). Hyaluronic acid functionalized nanoparticles loaded with IR780 and DOX for cancer chemo-photothermal therapy. Eur. J. Pharmaceut. Biopharmaceut. 137, 86–94. doi: 10.1016/j.ejpb.2019.02.016
Angelino, D., Berhow, M., Ninfali, P., and Jeffery, E. H. (2013). Caecal absorption of vitexin-2-O-xyloside and its aglycone apigenin, in the rat. Food Funct. 4, 1339–1345. doi: 10.1039/c3fo60047e
Banerjee, K., Banerjee, S., and Mandal, M. (2017). Enhanced chemotherapeutic efficacy of apigenin liposomes in colorectal cancer based on flavone-membrane interactions. J. Colloid Interface Sci. 491, 98–110. doi: 10.1016/j.jcis.2016.12.025
Banik, K., Ranaware, A. M., Harsha, C., Nitesh, T., Girisa, S., Deshpande, V., et al. (2020). Piceatannol: a natural stilbene for the prevention and treatment of cancer. Pharmacol. Res. 153:104635. doi: 10.1016/j.phrs.2020.104635
Bellazzo, A., Sicari, D., Valentino, E., Del Sal, G., and Collavin, L. (2018). Complexes formed by mutant p53 and their roles in breast cancer. Breast Cancer 10, 101–112. doi: 10.2147/BCTT.S145826
Bhattacharya, S., Mondal, L., Mukherjee, B., Dutta, L., Ehsan, I., Debnath, M. C., et al. (2018). Apigenin loaded nanoparticle delayed development of hepatocellular carcinoma in rats. Nanomedicine 14, 1905–1917. doi: 10.1016/j.nano.2018.05.011
Biesaga, M. (2011). Influence of extraction methods on stability of flavonoids. J. Chromatogr. A 1218, 2505–2512. doi: 10.1016/j.chroma.2011.02.059
Billard, C. (2012). Design of novel BH3 mimetics for the treatment of chronic lymphocytic leukemia. Leukemia 26, 2032–2038. doi: 10.1038/leu.2012.88
Birner, P., Gatterbauer, B., Oberhuber, G., Schindl, M., Rossler, K., Prodinger, A., et al. (2001). Expression of hypoxia-inducible factor-1 alpha in oligodendrogliomas: its impact on prognosis and on neoangiogenesis. Cancer 92, 165–171. doi: 10.1002/1097-0142(20010701)92:1<165::AID-CNCR1305>3.0.CO;2-F
Budhraja, A., Gao, N., Zhang, Z., Son, Y.-O., Cheng, S., Wang, X., et al. (2012). Apigenin induces apoptosis in human leukemia cells and exhibits anti-leukemic activity in vivo. Mol. Cancer Therapeut. 11, 132–142. doi: 10.1158/1535-7163.MCT-11-0343
Cai, H., Boocock, D. J., Steward, W. P., and Gescher, A. J. (2007). Tissue distribution in mice and metabolism in murine and human liver of apigenin and tricin, flavones with putative cancer chemopreventive properties. Cancer Chemother. Pharmacol. 60, 257–266. doi: 10.1007/s00280-006-0368-5
Cai, Y. Z., Mei, S., Jie, X., Luo, Q., and Corke, H. (2006). Structure-radical scavenging activity relationships of phenolic compounds from traditional Chinese medicinal plants. Life Sci. 78, 2872–2888. doi: 10.1016/j.lfs.2005.11.004
Cao, H.-H., Chu, J.-H., Kwan, H.-Y., Su, T., Yu, H., Cheng, C.-Y., et al. (2016). Inhibition of the STAT3 signaling pathway contributes to apigenin-mediated anti-metastatic effect in melanoma. Sci. Rep. 6:21731. doi: 10.1038/srep21731
Cao, X., Liu, B., Cao, W., Zhang, W., Zhang, F., Zhao, H., et al. (2013). Autophagy inhibition enhances apigenin-induced apoptosis in human breast cancer cells. Chin. J. Cancer Res. 25, 212–222. doi: 10.3978/j.issn.1000-9604.2013.04.01
Cardona, F., Andrés-Lacueva, C., Tulipani, S., Tinahones, F. J., and Queipo-Ortuño, M. I. (2013). Benefits of polyphenols on gut microbiota and implications in human health. J. Nutr. Biochem. 24, 1415–1422. doi: 10.1016/j.jnutbio.2013.05.001
Catarino, D. M., Alves-Silva, J. M., Pereira, O. R., and Cardoso, M. S. (2015). Antioxidant capacities of flavones and benefits in oxidative-stress related diseases. Curr. Top. Med. Chem. 15, 105–119. doi: 10.2174/1568026615666141209144506
Chaabane, W., User, S. D. M, El-Gazzah Jaksik, R., Sajjadi, E. J., et al. (2013). Autophagy, apoptosis, mitoptosis and necrosis: interdependence between those pathways and effects on cancer. Arch. Immunol. Ther. Exp. 61, 43–58. doi: 10.1007/s00005-012-0205-y
Channavajhala, P., and Seldin, D. C. (2002). Functional interaction of protein kinase CK2 and c-Myc in lymphomagenesis. Oncogene 21, 5280–5288. doi: 10.1038/sj.onc.1205640
Chatterjee, M., Andrulis, M., Stuhmer, T., Muller, E., Hofmann, C., Steinbrunn, T., et al. (2013). The PI3K/Akt signaling pathway regulates the expression of Hsp70, which critically contributes to Hsp90-chaperone function and tumor cell survival in multiple myeloma. Haematologica 98, 1132–1141. doi: 10.3324/haematol.2012.066175
Chen, C., Pore, N., Behrooz, A., Ismail-Beigi, F, and Maity, A. (2001). Regulation of glut1 mRNA by hypoxia-inducible factor-1 Interaction between H-ras and hypoxia. J. Biol. Chem. 276, 9519–9525. doi: 10.1074/jbc.M010144200
Chen, J., Lin, H., and Hu, M. (2003). Metabolism of flavonoids via enteric recycling: role of intestinal disposition. J. Pharmacol. Exp. Therapeut. 304, 1228–1235. doi: 10.1124/jpet.102.046409
Chen, M., Wang, X., Zha, D., Cai, F., Zhang, W., He, Y., et al. (2016). Apigenin potentiates TRAIL therapy of non-small cell lung cancer via upregulating DR4/DR5 expression in a p53-dependent manner. Sci. Rep. 6:35468. doi: 10.1038/srep35468
Chen, T., Li, L.-P., Lu, X.-Y., Jiang, H.-D., and Zeng, S. (2007). Absorption and excretion of luteolin and apigenin in rats after oral administration of Chrysanthemum morifolium extract. J. Agri. Food Chem. 55, 273–277. doi: 10.1021/jf062088r
Cheng, L., Yan, B., Chen, K., Jiang, Z., Zhou, C., Cao, J., et al. (2018). Resveratrol-induced downregulation of NAF-1 enhances the sensitivity of pancreatic cancer cells to gemcitabine via the ROS/Nrf2 signaling pathways. Oxid. Med. Cell Longev. 2018:9482018. doi: 10.1155/2018/9482018
Chien, M. H., Lin, Y. W., Wen, Y. C., Yang, Y. C., Hsiao, M., Chang, J. L., et al. (2019). Targeting the SPOCK1-snail/slug axis-mediated epithelial-to-mesenchymal transition by apigenin contributes to repression of prostate cancer metastasis. J. Exp. Clin. Cancer Res. 38:246. doi: 10.1186/s13046-019-1247-3
Choi, E. J., and Kim, G.-H. (2009). Apigenin induces apoptosis through a mitochondria/caspase-pathway in human breast cancer MDA-MB-453 cells. J. Clin. Biochem. Nutr. 44, 260–265. doi: 10.3164/jcbn.08-230
Chong, S. J. F., Marchi, S., Petroni, G., Kroemer, G., Galluzzi, L., and Pervaiz, S. (2020). Noncanonical cell fate regulation by Bcl-2 proteins. Trends Cell Biol. 30, 537–555. doi: 10.1016/j.tcb.2020.03.004
Chunhua, L., Donglan, L., Xiuqiong, F., Lihua, Z., Qin, F., Yawei, L., et al. (2013). Apigenin up-regulates transgelin and inhibits invasion and migration of colorectal cancer through decreased phosphorylation of AKT. J. Nutr. Biochem. 24, 1766–1775. doi: 10.1016/j.jnutbio.2013.03.006
Cirmi, S., Ferlazzo, N., Lombardo, G. E., Maugeri, A., Calapai, G., Gangemi, S., et al. (2016). Chemopreventive agents and inhibitors of cancer hallmarks: may citrus offer new perspectives?" Nutrients 8:698. doi: 10.3390/nu8110698
Coombs, M. R. P., Harrison, M. E., and Hoskin, D. W. (2016). Apigenin inhibits the inducible expression of programmed death ligand 1 by human and mouse mammary carcinoma cells. Cancer Lett. 380, 424–433. doi: 10.1016/j.canlet.2016.06.023
Cordani, M., Oppici, E., Dando, I., Butturini, E. E, Dalla Pozza, M., et al. (2016). Mutant p53 proteins counteract autophagic mechanism sensitizing cancer cells to mTOR inhibition. Mol. Oncol. 10, 1008–1029. doi: 10.1016/j.molonc.2016.04.001
Dai, J., Van Wie, P. G., Fai, L. Y., Kim, D., Wang, L., Poyil, P., et al. (2016). Downregulation of NEDD9 by apigenin suppresses migration, invasion, and metastasis of colorectal cancer cells. Toxicol. Appl. Pharmacol. 311, 106–112. doi: 10.1016/j.taap.2016.09.016
Das, S., Das, J., Samadder, A., Boujedaini, N., and Khuda-Bukhsh, A. R. (2012). Apigenin-induced apoptosis in A375 and A549 cells through selective action and dysfunction of mitochondria. Exp. Biol. Med. 237, 1433–1448. doi: 10.1258/ebm.2012.012148
Das, S., Das, J., Samadder, A., Paul, A., and Khuda-Bukhsh, A. R. (2013). Efficacy of PLGA-loaded apigenin nanoparticles in Benzo[a]pyrene and ultraviolet-B induced skin cancer of mice: mitochondria mediated apoptotic signalling cascades. Food Chem. Toxicol. 62, 670–680. doi: 10.1016/j.fct.2013.09.037
Dayalan Naidu, S., Kostov, R. V., and Dinkova-Kostova, A. T. (2015). Transcription factors Hsf1 and Nrf2 engage in crosstalk for cytoprotection. Trends Pharmacol. Sci. 36, 6–14. doi: 10.1016/j.tips.2014.10.011
Deng, Q., Fang, Q., Xie, B., Sun, H., Bao, Y., and Zhou, S. (2020). Exosomal long non-coding RNA MSTRG.292666.16 is associated with osimertinib (AZD9291) resistance in non-small cell lung cancer. Aging 12:103119. doi: 10.18632/aging.103119
Dosio, F., Arpicco, S., Stella, B., and Fattal, E. (2016). Hyaluronic acid for anticancer drug and nucleic acid delivery. Adv. Drug Deliv. Rev. 97, 204–236. doi: 10.1016/j.addr.2015.11.011
Elmore, S. (2007). Apoptosis: a review of programmed cell death. Toxicol. Pathol. 35, 495–516. doi: 10.1080/01926230701320337
Erdogan, S., Doganlar, O., Doganlar, Z. B., Serttas, R., Turkekul, K., Dibirdik, I., et al. (2016). The flavonoid apigenin reduces prostate cancer CD44+ stem cell survival and migration through PI3K/Akt/NF-κB signaling. Life Sci. 162, 77–86. doi: 10.1016/j.lfs.2016.08.019
Fang, J., Bao, Y. Y., Zhou, S. H., and Fan, J. (2015). Apigenin inhibits the proliferation of adenoid cystic carcinoma via suppression of glucose transporter-1. Mol. Med. Rep. 12, 6461–6466. doi: 10.3892/mmr.2015.4233
Farhood, B., Goradel, N. H., Mortezaee, K., Khanlarkhani, N., Najafi, M., and Sahebkar, A. (2019). Melatonin and cancer: From the promotion of genomic stability to use in cancer treatment. J. Cell. Physiol. 234, 5613–5627. doi: 10.1002/jcp.27391
Farhood, B., Khodamoradi, E., Hoseini-Ghahfarokhi, M., Motevaseli, E., Mirtavoos-Mahyari, H., Eleojo Musa, A., et al. (2020). TGF-β in radiotherapy: mechanisms of tumor resistance and normal tissues injury. Pharmacol. Res. 155:104745. doi: 10.1016/j.phrs.2020.104745
Farooqi, A. A., Qureshi, M. Z., Khalid, S., Attar, R., Martinelli, C., Sabitaliyevich, U. Y., et al. (2019). Regulation of cell signaling pathways by berberine in different cancers: searching for missing pieces of an incomplete jig-saw puzzle for an effective cancer therapy. Cancers 11:478. doi: 10.3390/cancers11040478
Freitas, S., Costa, S., Azevedo, C., Carvalho, G., Freire, S., Barbosa, P., et al. (2011). Flavonoids inhibit angiogenic cytokine production by human glioma cells. Phytother. Res. 25, 916–921. doi: 10.1002/ptr.3338
Gaballah, H. H., Gaber, R. A., and Mohamed, D. A. (2017). Apigenin potentiates the antitumor activity of 5-FU on solid Ehrlich carcinoma: crosstalk between apoptotic and JNK-mediated autophagic cell death platforms. Toxicol. Appl. Pharmacol. 316, 27–35. doi: 10.1016/j.taap.2016.12.012
Galluzzi, L., and Green, D. R. (2019). Autophagy-independent functions of the autophagy machinery. Cell 177, 1682–1699. doi: 10.1016/j.cell.2019.05.026
Galluzzi, L., and Kroemer, G. (2020). Transient autophagy inhibition precipitates oncogenesis: a red flag for pharmacological autophagy inhibitors? Trends Cell Biol. 30, 339–340. doi: 10.1016/j.tcb.2020.02.004
Garcea, G., Doucas, H., Steward, W. P., Dennison, A. R., and Berry, D. P. (2006). Hypoxia and angiogenesis in pancreatic cancer. ANZ J. Surg. 76, 830–842. doi: 10.1111/j.1445-2197.2006.03872.x
Garufi, A., D'Orazi, V., Crispini, A., and D'Orazi, G. (2015). Zn(II)-curc targets p53 in thyroid cancer cells. Int. J. Oncol. 47, 1241–1248. doi: 10.3892/ijo.2015.3125
Garufi, A., Pistritto, G., Cirone, M., and D'Orazi, G. (2016). Reactivation of mutant p53 by capsaicin, the major constituent of peppers. J. Exp. Clin. Cancer Res. 35:136. doi: 10.1186/s13046-016-0417-9
Garufi, A., Pucci, D., D'Orazi, V., Cirone, M., Bossi, G., Avantaggiati, M. L., et al. (2014). Degradation of mutant p53H175 protein by Zn(II) through autophagy. Cell Death Dis. 5:e1271. doi: 10.1038/cddis.2014.217
Gilardini Montani, M. S., Cecere, N., and Granato, M. (2019). Mutant p53, stabilized by its interplay with HSP90, activates a positive feed-back loop between NRF2 and p62 that induces chemo-resistance to apigenin in pancreatic cancer cells. Cancers 11:703. doi: 10.3390/cancers11050703
Gourley, C., Balmaña, J., Ledermann, J. A., Serra, V., Dent, R., Loibl, S., et al. (2019). Moving from poly (ADP-Ribose) polymerase inhibition to targeting DNA repair and DNA damage response in cancer therapy. J. Clin. Oncol. 37, 2257–2269. doi: 10.1200/JCO.18.02050
Gowda, R., Jones, N. R., Banerjee, S., and Robertson, G. P. (2013). Use of nanotechnology to develop multi-drug inhibitors for cancer therapy. J. Nanomed. Nanotechnol. 4:184. doi: 10.4172/2157-7439.1000184
Gradolatto, A., Basly, J.-P., Berges, R., Teyssier, C., Chagnon, M.-C., Siess, M.-H., et al. (2005). Pharmacokinetics and metabolism of apigenin in female and male rats after a single oral administration. Drug Metabol. Disposition 33, 49–54. doi: 10.1124/dmd.104.000893
Granato, M., Gilardini, M. S., Montani Santarelli, R., D'Orazi, G., Faggioni, A., and Cirone, M. (2017). Apigenin, by activating p53 and inhibiting STAT3, modulates the balance between pro-apoptotic and pro-survival pathways to induce PEL cell death. J. Exp. Clin. Cancer Res. 36:167. doi: 10.1186/s13046-017-0632-z
Gupta, S., Afaq, F., and Mukhtar, H. (2001). Selective growth-inhibitory, cell-cycle deregulatory and apoptotic response of apigenin in normal versus human prostate carcinoma cells. Biochem. Biophys. Res. Commun. 287, 914–920. doi: 10.1006/bbrc.2001.5672
Gupta, S., Afaq, F., and Mukhtar, H. (2002). Involvement of nuclear factor-kappa B, Bax and Bcl-2 in induction of cell cycle arrest and apoptosis by apigenin in human prostate carcinoma cells. Oncogene 21, 3727–3738. doi: 10.1038/sj.onc.1205474
Gurung, R. B., Kim, E.-H., Oh, T.-J., and Sohng, J. K. (2013). Enzymatic synthesis of apigenin glucosides by glucosyltransferase (YjiC) from Bacillus licheniformis DSM 13. Mol. Cells 36, 355–361. doi: 10.1007/s10059-013-0164-0
Hamada, S., Taguchi, K., Masamune, A., Yamamoto, M., and Shimosegawa, T. (2017). Nrf2 promotes mutant K-ras/p53-driven pancreatic carcinogenesis. Carcinogenesis 38, 661–670. doi: 10.1093/carcin/bgx043
Hashemi Goradel, N., Najafi, M., Salehi, E., Farhood, B., and Mortezaee, K. (2019). Cyclooxygenase-2 in cancer: a review. J. Cell. Physiol. 234, 5683–5699. doi: 10.1002/jcp.27411
Hasnat, M., Pervin, M., Lim, J., and Lim, B. (2015). Apigenin attenuates melanoma cell migration by inducing anoikis through integrin and focal adhesion kinase inhibition. Molecules 20, 21157–21166. doi: 10.3390/molecules201219752
Hazari, Y., Bravo-San Pedro, J. M., Hetz, C., Galluzzi, L., and Kroemer, G. (2020). Autophagy in hepatic adaptation to stress. J. Hepatol. 72, 183–196. doi: 10.1016/j.jhep.2019.08.026
Hoca, M., Becer, E., Kabadayi, H., Yucecan, S., and Vatansever, H. S. (2019). The effect of resveratrol and quercetin on epithelial-mesenchymal transition in pancreatic cancer stem cell. Nutr. Cancer 72, 1231–1242. doi: 10.1080/01635581.2019.1670853
Hu, C.-M. J., and Zhang, L. (2012). Nanoparticle-based combination therapy toward overcoming drug resistance in cancer. Biochem. Pharmacol. 83, 1104–1111. doi: 10.1016/j.bcp.2012.01.008
Hu, X. W., Meng, D., and Fang, J. (2008). Apigenin inhibited migration and invasion of human ovarian cancer A2780 cells through focal adhesion kinase. Carcinogenesis 29, 2369–2376. doi: 10.1093/carcin/bgn244
Huang, T., Chen, Q. F., Chang, B. Y., Shen, L. J., Li, W., Wu, P. H., et al. (2019a). TFAP4 Promotes hepatocellular carcinoma invasion and metastasis via activating the PI3K/AKT signaling pathway. Dis. Markers 2019:7129214. doi: 10.1155/2019/7129214
Huang, X., Gan, G., Wang, X., Xu, T., and Xie, W. (2019b). The HGF-MET axis coordinates liver cancer metabolism and autophagy for chemotherapeutic resistance. Autophagy 15, 1258–1279. doi: 10.1080/15548627.2019.1580105
Inoue, M., Hager, J. H., Ferrara, N., Gerber, H. P., and Hanahan, D. (2002). VEGF-A has a critical, nonredundant role in angiogenic switching and pancreatic beta cell carcinogenesis. Cancer Cell 1, 193–202. doi: 10.1016/S1535-6108(02)00031-4
Ito, H., Duxbury, M., Zinner, M. J., Ashley, S. W., and Whang, E. E. (2004). Glucose transporter-1 gene expression is associated with pancreatic cancer invasiveness and MMP-2 activity. Surgery 136, 548–556. doi: 10.1016/j.surg.2004.05.032
Ivan, M., Kondo, K., Yang, H., Kim, W., Valiando, J., Ohh, M., et al. (2001). HIFα targeted for VHL-mediated destruction by proline hydroxylation: implications for O2 sensing. Science 292, 464–468. doi: 10.1126/science.1059817
Jamaledin, R., Di Natale, C., Onesto, V., Taraghdari, Z. B., Zare, E. N., Makvandi, P., et al. (2020). Progress in microneedle-mediated protein delivery. J. Clin. Med. 9:542. doi: 10.3390/jcm9020542
Jangdey, M. S., Gupta, A., and Saraf, S. (2017). Fabrication, in-vitro characterization, and enhanced in-vivo evaluation of carbopol-based nanoemulsion gel of apigenin for UV-induced skin carcinoma. Drug Deliv. 24, 1026–1036. doi: 10.1080/10717544.2017.1344333
Jangdey, M. S., Kaur, C. D., and Saraf, S. (2019). Efficacy of Concanavalin-A conjugated nanotransfersomal gel of apigenin for enhanced targeted delivery of UV induced skin malignant melanoma. Artif. Cells Nanomed. Biotechnol. 47, 904–916. doi: 10.1080/21691401.2019.1578784
Jin, X., Yang, Q., and Zhang, Y. (2017). Synergistic apoptotic effects of apigenin TPGS liposomes and tyroservatide: implications for effective treatment of lung cancer. Int. J. Nanomed. 12, 5109–5118. doi: 10.2147/IJN.S140096
Johnson, J., and de Mejia, G. E. (2011). Dietary factors and pancreatic cancer: the role of food bioactive compounds. Mol. Nutr. Food Res. 55, 58–73. doi: 10.1002/mnfr.201000420
Johnson, J. L., and de Mejia, G. E. (2013). Flavonoid apigenin modified gene expression associated with inflammation and cancer and induced apoptosis in human pancreatic cancer cells through inhibition of GSK-3β/NF-κ B signaling cascade. Mol. Nutr. Food Res. 57, 2112–2127. doi: 10.1002/mnfr.201300307
Johnson, J. L., and Gonzalez de Mejia, E. (2013). Interactions between dietary flavonoids apigenin or luteolin and chemotherapeutic drugs to potentiate anti-proliferative effect on human pancreatic cancer cells, in vitro. Food Chem. Toxicol. 60, 83–91. doi: 10.1016/j.fct.2013.07.036
Johnson, J. L., Rupasinghe, S. G., Stefani, F., Schuler, M. A., and Gonzalez de Mejia, E. (2011). Citrus flavonoids luteolin, apigenin, and quercetin inhibit glycogen synthase kinase-3β enzymatic activity by lowering the interaction energy within the binding cavity. J. Med. Food 14, 325–333. doi: 10.1089/jmf.2010.0310
Karayiannakis, A. J., Bolanaki, H., Syrigos, K. N., Asimakopoulos, B., Polychronidis, A., Anagnostoulis, S., et al. (2003). Serum vascular endothelial growth factor levels in pancreatic cancer patients correlate with advanced and metastatic disease and poor prognosis. Cancer Lett. 194, 119–124. doi: 10.1016/S0304-3835(03)00047-8
Karim, R., Palazzo, C., Laloy, J., Delvigne, A. S., Vanslambrouck, S., Jerome, C., et al. (2017). Development and evaluation of injectable nanosized drug delivery systems for apigenin. Int. J. Pharm. 532, 757–768. doi: 10.1016/j.ijpharm.2017.04.064
Kaur, P., Shukla, S., and Gupta, S. (2008). Plant flavonoid apigenin inactivates Akt to trigger apoptosis in human prostate cancer: an in vitro and in vivo study. Carcinogenesis 29, 2210–2217. doi: 10.1093/carcin/bgn201
Ketkaew, Y., Osathanon, T., Pavasant, P., and Sooampon, S. (2017). Apigenin inhibited hypoxia induced stem cell marker expression in a head and neck squamous cell carcinoma cell line. Archiv. Oral Biol. 74, 69–74. doi: 10.1016/j.archoralbio.2016.11.010
Kim, B., Jung, N., Lee, S., Sohng, J. K., and Jung, H. J. (2016). Apigenin inhibits cancer stem cell-like phenotypes in human glioblastoma cells via suppression of c-met signaling. Phytother. Res. 30, 1833–1840. doi: 10.1002/ptr.5689
Kim, J. H., Moon, M. J., Kim, D. Y., Heo, S. H., and Jeong, Y. Y. (2018). Hyaluronic acid-based nanomaterials for cancer therapy. Polymers 10:1133. doi: 10.3390/polym10101133
King, J. C., Lu, Q-Y., Li, G., Moro, A., Takahashi, H., Chen, M., et al. (2012). Evidence for activation of mutated p53 by apigenin in human pancreatic cancer. Biochim. Biophys. Acta 1823, 593–604. doi: 10.1016/j.bbamcr.2011.12.008
Korolchuk, V. I., and Rubinsztein, D. C. (2011). Regulation of autophagy by lysosomal positioning. Autophagy 7, 927–928. doi: 10.4161/auto.7.8.15862
Koukourakis, M. I., Bentzen, S. M., Giatromanolaki, A., Wilson, G. D., Daley, F. M., Saunders, M. I., et al. (2006). Endogenous markers of two separate hypoxia response pathways (hypoxia inducible factor 2 alpha and carbonic anhydrase 9) are associated with radiotherapy failure in head and neck cancer patients recruited in the CHART randomized trial. J. Clin. Oncol. 24, 727–735. doi: 10.1200/JCO.2005.02.7474
Krajka-Kuzniak, V., Cykowiak, M., Szaefer, H., Kleszcz, R., and Baer-Dubowska, W. (2020). Combination of xanthohumol and phenethyl isothiocyanate inhibits NF-κB and activates Nrf2 in pancreatic cancer cells. Toxicol in vitro 65:104799. doi: 10.1016/j.tiv.2020.104799
Kumari, S., Badana, A. K., Murali Mohan, G., Shailender, G., and Malla, R. (2018). Reactive oxygen species: a key constituent in cancer survival. Biomark Insights 13:1177271918755391. doi: 10.1177/1177271918755391
Lakshmanan, A., Scarberry, D., Green, J. A., Zhang, X. S, and Selmi-Ruby Jhiang, S. M. (2015). Modulation of thyroidal radioiodide uptake by oncological pipeline inhibitors and Apigenin. Oncotarget 6:31792. doi: 10.18632/oncotarget.5172
Landesman-Bollag, E., Channavajhala, P. L., Cardiff, R. D., and Seldin, D. C. (1998). p53 deficiency and misexpression of protein kinase CK2α collaborate in the development of thymic lymphomas in mice. Oncogene 16, 2965–2974. doi: 10.1038/sj.onc.1201854
Larsson, S. C., Håkansson, N., Näslund, I., Bergkvist, L., and Wolk, A. (2006). Fruit and vegetable consumption in relation to pancreatic cancer risk: a prospective study. Cancer Epidemiol. Biomarkers Prev. 15, 301–305. doi: 10.1158/1055-9965.EPI-05-0696
Lee, H. H., Jung, J., Moon, A., Kang, H., and Cho, H. (2019a). Antitumor and anti-invasive effect of apigenin on human breast carcinoma through suppression of IL-6 expression. Int. J. Mol. Sci. 20:133143. doi: 10.3390/ijms20133143
Lee, J. H., Chinnathambi, A., Alharbi, S. A., Shair, O. H. M., Sethi, G., and Ahn, K. S. (2019b). Farnesol abrogates epithelial to mesenchymal transition process through regulating Akt/mTOR pathway. Pharmacol. Res. 150:104504. doi: 10.1016/j.phrs.2019.104504
Lee, J. H., Mohan, C. D., Shanmugam, M. K., Rangappa, S., Sethi, G., Siveen, K. S., et al. (2020). Vitexin abrogates invasion and survival of hepatocellular carcinoma cells through targeting STAT3 signaling pathway. Biochimie 175, 58–68. doi: 10.1016/j.biochi.2020.05.006
Lee, J. H., Rangappa, S., Mohan, C. D., Basappa, G., Sethi, Z.-X., Lin, K. S., et al. (2019c). Brusatol, a Nrf2 inhibitor targets STAT3 signaling cascade in head and neck squamous cell carcinoma. Biomolecules 9:550. doi: 10.3390/biom9100550
Lee, Y., Sung, B., Kang, Y. J., Kim, D. H., Jang, J. Y., Hwang, S. Y., et al. (2014). Apigenin-induced apoptosis is enhanced by inhibition of autophagy formation in HCT116 human colon cancer cells. Int. J. Oncol. 44, 1599–1606. doi: 10.3892/ijo.2014.2339
Lee, Y.-M., Lee, G., Oh, T-I., Kim, B. M., Shim, D. W., Lee, K-H., et al. (2016). Inhibition of glutamine utilization sensitizes lung cancer cells to apigenin-induced apoptosis resulting from metabolic and oxidative stress. Int. J. Oncol. 48, 399–408. doi: 10.3892/ijo.2015.3243
Li, B., Robinson, D. H., and Birt, D. F. (1997). Evaluation of properties of apigenin and [G-3H] apigenin and analytic method development. J. Pharmaceut. Sci. 86, 721–725. doi: 10.1021/js960383s
Li, D., Yallowitz, A., Ozog, L., and Marchenko, N. (2014). A gain-of-function mutant p53-HSF1 feed forward circuit governs adaptation of cancer cells to proteotoxic stress. Cell Death Dis. 5:e1194. doi: 10.1038/cddis.2014.158
Li, W., Wang, Z., Xiao, X., Han, L., Wu, Z., Ma, Q., et al. (2019). Curcumin attenuates hyperglycemia-driven EGF-induced invasive and migratory abilities of pancreatic cancer via suppression of the ERK and AKT pathways. Oncol. Rep. 41, 650–658. doi: 10.3892/or.2018.6833
Liang, N., Liu, X., Zhang, S., and Sun, H. (2019). The role of Beclin 1 in IR-induced crosstalk between autophagy and G2/M cell cycle arrest. Cell Signal 62:109353. doi: 10.1016/j.cellsig.2019.109353
Liao, X.-Z., Gao, Y., Sun, L-L., Liu, H., Chen, H-R., Yu, L., et al. (2020). Rosmarinic acid reverses non-small cell lung cancer cisplatin resistance by activating the MAPK signaling pathway. Phytother. Res. 34, 1142–1153. doi: 10.1002/ptr.6584
Lieben, L. (2017). Immunotherapy: keeping breast cancer in check. Nat. Rev. Cancer 17, 454–455. doi: 10.1038/nrc.2017.55
Lim, W., Park, S., Bazer, F. W., and Song, G. (2016). Apigenin reduces survival of choriocarcinoma cells by inducing apoptosis via the PI3K/AKT and ERK1/2 MAPK pathways. J. Cell Physiol. 231, 2690–2699. doi: 10.1002/jcp.25372
Lin, X., Khalid, S., Qureshi, M. Z., Attar, R., Yaylim, I., Ucak, I., et al. (2016). VEGF mediated signaling in oral cancer. Cell Mol. Biol. 62, 64–68. doi: 10.14715/cmb/2016.62.14.11
Lisek, K., Campaner, E., Ciani, Y., Walerych, D., and Del Sal, G. (2018). Mutant p53 tunes the NRF2-dependent antioxidant response to support survival of cancer cells. Oncotarget 9, 20508–20523. doi: 10.18632/oncotarget.24974
Liskova, A., Stefanicka, P., Samec, M., Smejkal, K., Zubor, P., Bielik, T., et al. (2020). Dietary phytochemicals as the potential protectors against carcinogenesis and their role in cancer chemoprevention. Clin. Exp. Med. 20, 173–190. doi: 10.1007/s10238-020-00611-w
Liu, J., Cao, X. C., Xiao, Q., and Quan, M. F. (2015a). Apigenin inhibits HeLa sphere-forming cells through inactivation of casein kinase 2α. Mol. Med. Rep. 11, 665–669. doi: 10.3892/mmr.2014.2720
Liu, L. Z., Fang, J., Zhou, Q., Hu, X., Shi, X., and Jiang, B. H. (2005). Apigenin inhibits expression of vascular endothelial growth factor and angiogenesis in human lung cancer cells: implication of chemoprevention of lung cancer. Mol. Pharmacol. 68, 635–643. doi: 10.1124/mol.105.011254
Liu, X., Li, L., Lv, L., Chen, D., Shen, L., and Xie, Z. (2015b). Apigenin inhibits the proliferation and invasion of osteosarcoma cells by suppressing the Wnt/β-catenin signaling pathway. Oncol. Rep. 34, 1035–1041. doi: 10.3892/or.2015.4022
Liu, Y., and Hu, M. (2002). Absorption and metabolism of flavonoids in the caco-2 cell culture model and a perused rat intestinal model. Drug Metabol. Disposition 30, 370–377. doi: 10.1124/dmd.30.4.370
Liu, Y., Li, Q., Zhou, L., Xie, N., Nice, E. C., Zhang, H., et al. (2016). Cancer drug resistance: redox resetting renders a way. Oncotarget 7, 42740–42761. doi: 10.18632/oncotarget.8600
Liu, Z. J., Xu, W., Han, J., Liu, Q. Y., Gao, L. F., Wang, X. H., et al. (2020). Quercetin induces apoptosis and enhances gemcitabine therapeutic efficacy against gemcitabine-resistant cancer cells. Anticancer Drugs. 31, 684–692. doi: 10.1097/CAD.0000000000000933
Long, J., Zhang, Y., Yu, X., Yang, J., LeBrun, D. G., Chen, C., et al. (2011). Overcoming drug resistance in pancreatic cancer. Expert Opin. Ther. Targets 15, 817–828. doi: 10.1517/14728222.2011.566216
Mabjeesh, N., and Amir, S. (2007). Hypoxia-inducible factor (HIF) in human tumorigenesis. Histol. Histopathol. 22, 559–572. doi: 10.14670/HH-22.559
Madunić, J., Madunić, I. V., Gajski, G., Popić, J., and Garaj-Vrhovac, V. (2018). Apigenin: a dietary flavonoid with diverse anticancer properties. Cancer Lett. 413, 11–22. doi: 10.1016/j.canlet.2017.10.041
Mafuvadze, B., Liang, Y. C., Besch-Williford, Zhang, X., and Hyder, S. M. (2012). Apigenin induces apoptosis and blocks growth of medroxyprogesterone acetate-dependent BT-474 xenograft tumors. Horm. Cancer 3, 160–171. doi: 10.1007/s12672-012-0114-x
Maggioni, D., Garavello, W., Rigolio, R., Pignataro, L., Gaini, R., and Nicolini, G. (2013). Apigenin impairs oral squamous cell carcinoma growth in vitro inducing cell cycle arrest and apoptosis. Int. J. Oncol. 43, 1675–1682. doi: 10.3892/ijo.2013.2072
Mahmoudi, S., Ghorbani, M., Sabzichi, M., Ramezani, F., Hamishehkar, H., and Samadi, N. (2019). Targeted hyaluronic acid-based lipid nanoparticle for apigenin delivery to induce Nrf2-dependent apoptosis in lung cancer cells. J. Drug Deliv. Sci. Technol. 49, 268–276. doi: 10.1016/j.jddst.2018.11.013
Makvandi, P., Ali, G. W., Della Sala, F., Abdel-Fattah, W. I., and Borzacchiello, A. (2019). Biosynthesis and characterization of antibacterial thermosensitive hydrogels based on corn silk extract, hyaluronic acid and nanosilver for potential wound healing. Carboyhdrate Polym. 223, 115023–115034. doi: 10.1016/j.carbpol.2019.115023
Makvandi, P., Ali, G. W., Della Sala, F., Abdel-Fattah, W. I., and Borzacchiello, A. (2020). Hyaluronic acid/corn silk extract based injectable nanocomposite: a biomimetic antibacterial scaffold for bone tissue regeneration. Mater. Sci. Eng. C Mater. Biol. Appl. 107:110195. doi: 10.1016/j.msec.2019.110195
Mantovani, F., Collavin, L., and Del Sal, G. (2019). Mutant p53 as a guardian of the cancer cell. Cell Death Differ. 26, 199–212. doi: 10.1038/s41418-018-0246-9
Maruszewska, A., and Tarasiuk, J. (2020). Quercetin triggers induction of apoptotic and lysosomal death of sensitive and multidrug resistant leukaemia HL60 cells. Nutr. Cancer. doi: 10.1080/01635581.2020.1752745. [Epub ahead of print].
Masuelli, L., Benvenuto, M., Mattera, R., E., Di Stefano, Zago, E., Taffera, G., et al. (2017). In vitro and in vivo anti-tumoral effects of the flavonoid apigenin in malignant mesothelioma. Front. Pharmacol. 8:373. doi: 10.3389/fphar.2017.00373
Melillo, G. (2006). Inhibiting hypoxia-inducible factor 1 for cancer therapy. Mol. Cancer Res. 4, 601–605. doi: 10.1158/1541-7786.MCR-06-0235
Melstrom, L. G., Salabat, M. R., Ding, X. Z., Milam, B. M., Strouch, M., Pelling, J. C., et al. (2008). Apigenin inhibits the GLUT-1 glucose transporter and the phosphoinositide 3-kinase/Akt pathway in human pancreatic cancer cells. Pancreas 37, 426–431. doi: 10.1097/MPA.0b013e3181735ccb
Melstrom, L. G., Salabat, M. R., Ding, X. Z., Strouch, M. J., Grippo, P. J., Mirzoeva, S., et al. (2011). Apigenin down-regulates the hypoxia response genes: HIF-1α, GLUT-1, and VEGF in human pancreatic cancer cells. J. Surg. Res. 167, 173–181. doi: 10.1016/j.jss.2010.10.041
Meng, S., Zhu, Y., Li, J. F., Wang, X., Liang, Z., Li, S. Q., et al. (2017). Apigenin inhibits renal cell carcinoma cell proliferation. Oncotarget 8, 19834–19842. doi: 10.18632/oncotarget.15771
Mickova, A., Buzgo, M., Benada, O., Rampichova, M., Fisar, Z., Filova, E., et al. (2012). Core/shell nanofibers with embedded liposomes as a drug delivery system. Biomacromolecules 13, 952–962. doi: 10.1021/bm2018118
Middleton, E., Kandaswami, C., and Theoharides, T. C. (2000). The effects of plant flavonoids on mammalian cells: implications for inflammation, heart disease, and cancer. Pharmacol. Rev. 52, 673–751.
Mirzoeva, S., Franzen, C. A., and Pelling, J. C. (2014). Apigenin inhibits TGF-β-induced VEGF expression in human prostate carcinoma cells via a Smad2/3-and Src-dependent mechanism. Mol. Carcinogenesis 53, 598–609. doi: 10.1002/mc.22005
Moore, P. S., Sipos, B., Orlandini, S., Sorio, C., Real, F. X., Lemoine, N. R., et al. (2001). Genetic profile of 22 pancreatic carcinoma cell lines. Analysis of K-ras, p53, p16 and DPC4/Smad4. Virchows Arch. 439, 798–802. doi: 10.1007/s004280100474
Mortezaee, K., Najafi, M., Farhood, B., Ahmadi, A., Potes, Y., Shabeeb, D., et al. (2019a). Modulation of apoptosis by melatonin for improving cancer treatment efficiency: an updated review. Life Sci. 228, 228–241. doi: 10.1016/j.lfs.2019.05.009
Mortezaee, K., Najafi, M., Farhood, B., Ahmadi, A., Shabeeb, D., and Musa, A. E. (2019b). NF-κB targeting for overcoming tumor resistance and normal tissues toxicity. J. Cell. Physiol. 234, 17187–17204. doi: 10.1002/jcp.28504
Mortezaee, K., Potes, Y., H., Mirtavoos-Mahyari Motevaseli, E., Shabeeb, D., Musa, A. E., et al. (2019c). Boosting immune system against cancer by melatonin: a mechanistic viewpoint. Life Sci. 238:116960. doi: 10.1016/j.lfs.2019.116960
Mortezaee, K., Salehi, E., Mirtavoos-mahyari, H., Motevaseli, E., Najafi, M., Farhood, B., et al. (2019d). Mechanisms of apoptosis modulation by curcumin: implications for cancer therapy. J. Cell. Physiol. 234, 12537–12550. doi: 10.1002/jcp.28122
Mrazek, A. A., Porro, L. J., Bhatia, V., Falzon, M., Spratt, H., Zhou, J., et al. (2015). Apigenin inhibits pancreatic stellate cell activity in pancreatitis. J. Surg. Res. 196, 8–16. doi: 10.1016/j.jss.2015.02.032
Mueckler, M. (1994). Facilitative glucose transporters. Eur. J. Biochem. 219, 713–725. doi: 10.1111/j.1432-1033.1994.tb18550.x
Muller, P. A., and Vousden, K. H. (2014). Mutant p53 in cancer: new functions and therapeutic opportunities. Cancer Cell 25, 304–317. doi: 10.1016/j.ccr.2014.01.021
Murugan, A. K. (2019). mTOR: role in cancer, metastasis and drug resistance. Semin. Cancer Biol. 59, 92–111. doi: 10.1016/j.semcancer.2019.07.003
Nagaraju, G. P., Benton, L., Bethi, S. R., Shoji, M., and El-Rayes, B. F. (2019). Curcumin analogs: their roles in pancreatic cancer growth and metastasis. Int. J. Cancer 145, 10–19. doi: 10.1002/ijc.31867
Najafi, M., Cheki, M., Rezapoor, S., Geraily, G., Motevaseli, E., Carnovale, C., et al. (2018). Metformin: prevention of genomic instability and cancer: a review. Mutation Res. 827, 1–8. doi: 10.1016/j.mrgentox.2018.01.007
Najafi, M., Goradel, N. H., Farhood, B., Salehi, E., Solhjoo, S., Toolee, H., et al. (2019a). Tumor microenvironment: interactions and therapy. J. Cell. Physiol. 234, 5700–5721. doi: 10.1002/jcp.27425
Najafi, M., Hashemi Goradel, N., Farhood, B., Salehi, E., Nashtaei, M. S., Khanlarkhani, N., et al. (2019b). Macrophage polarity in cancer: a review. J. Cell. Biochem. 120, 2756–2765. doi: 10.1002/jcb.27646
Nazim, U. M., Yin, H., and Park, S. Y. (2020). Downregulation of cFLIP and upregulation of DR5 by cantharidin sensitizes TRAILmediated apoptosis in prostate cancer cells via autophagy flux. Int. J. Mol. Med. 46, 280–288. doi: 10.3892/ijmm.2020.4566
Nelson, N., Szekeres, K., Iclozan, C., Rivera, I. O., McGill, A., Johnson, G., et al. (2017). Apigenin: selective CK2 inhibitor increases Ikaros expression and improves T cell homeostasis and function in murine pancreatic cancer. PLoS ONE 12:e0170197. doi: 10.1371/journal.pone.0170197
Nelson, N., Xiang, S., Zhang, X., Gilvary, D., Djeu, J., Husain, K., et al. (2015). Murine pancreatic adenocarcinoma reduces Ikaros expression and disrupts T cell homeostasis. PLoS ONE 10:e0115546. doi: 10.1371/journal.pone.0115546
Osada, M., Imaoka, S., and Funae, Y. (2004). Apigenin suppresses the expression of VEGF, an important factor for angiogenesis, in endothelial cells via degradation of HIF-1α protein. FEBS Lett. 575, 59–63. doi: 10.1016/j.febslet.2004.08.036
Ougolkov, A. V., Fernandez-Zapico, M. E., Savoy, D. N., Urrutia, R. A., and Billadeau, D. D. (2005). Glycogen synthase kinase-3β participates in nuclear factor κB-mediated gene transcription and cell survival in pancreatic cancer cells. Cancer Res. 65, 2076–2081. doi: 10.1158/0008-5472.CAN-04-3642
Pan, X., Yang, Z., Yang, Z., Zhou, S., Zhang, H., and Zang, L. (2013). Effect of apigenin on proliferation and apoptosis of human lung cancer NCI-H460 cells. J. Southern Med. University 33:1137.
Pápay, Z. E., Kállai-Szabó, N., Balogh, E., Ludányi, K., Klebovich, I., and Antal, I. (2017b). Controlled release oral delivery of apigenin containing pellets with antioxidant activity. Curr. Drug Deliv. 14, 145–154. doi: 10.2174/1567201813666160602193047
Pápay, Z. E., Kósa, A., Böddi, B., Merchant, Z., Saleem, I. Y., Zariwala, M. G., et al. (2017a). Study on the pulmonary delivery system of apigenin-loaded albumin nanocarriers with antioxidant activity. J. Aerosol. Med. Pulm. Drug Deliv. 30, 274–288. doi: 10.1089/jamp.2016.1316
Patel, D., Shukla, S., and Gupta, S. (2007). Apigenin and cancer chemoprevention: progress, potential and promise (review). Int. J. Oncol. 30, 233–245. doi: 10.3892/ijo.30.1.233
Patra, S., Mishra, S. R., Behera, B. P., Mahapatra, K. K., Panigrahi, D. P., Bhol, C. S., et al. (2020). Autophagy-modulating phytochemicals in cancer therapeutics: current evidences and future perspectives. Semin. Cancer Biol. doi: 10.1016/j.semcancer.2020.05.008. [Epub ahead of print].
Perrott, K. M., Wiley, C. D., Desprez, P-Y., and Campisi, J. (2017). Apigenin suppresses the senescence-associated secretory phenotype and paracrine effects on breast cancer cells. Geroscience 39, 161–173. doi: 10.1007/s11357-017-9970-1
Pham, H., Chen, M., Takahashi, H., King, J., Reber, H. A., Hines, O. J., et al. (2012). Apigenin inhibits NNK-induced focal adhesion kinase activation in pancreatic cancer cells. Pancreas 41:1306. doi: 10.1097/MPA.0b013e31824d64d9
Polesel, J., Talamini, R., Negri, E., Bosetti, C., Boz, G., Lucenteforte, E., et al. (2010). Dietary habits and risk of pancreatic cancer: an Italian case-control study. Cancer Causes Control 21, 493–500. doi: 10.1007/s10552-009-9480-2
Qin, Y., Zhao, D., Zhou, H.-g., Wang, X.-h., Zhong, W.-l., Chen, S., et al. (2016). Apigenin inhibits NF-κB and snail signaling, EMT and metastasis in human hepatocellular carcinoma. Oncotarget 7:41421. doi: 10.18632/oncotarget.9404
Rojo de la Vega, M., Chapman, E., and Zhang, D. D. (2018). NRF2 and the hallmarks of cancer. Cancer Cell 34, 21–43. doi: 10.1016/j.ccell.2018.03.022
Ruela-de-Sousa, R. R., Fuhler, G. M., Blom, N., Ferreira, C. V., Aoyama, H., and Peppelenbosch, M. P. (2010). Cytotoxicity of apigenin on leukemia cell lines: implications for prevention and therapy. Cell Death Dis. 1:e19. doi: 10.1038/cddis.2009.18
Salabat, M. R., Melstrom, L. G., Strouch, M. J., Ding, X. Z., Milam, B. M., Ujiki, M. B., et al. (2008). Geminin is overexpressed in human pancreatic cancer and downregulated by the bioflavanoid apigenin in pancreatic cancer cell lines. Mol. Carcinogenesis 47, 835–844. doi: 10.1002/mc.20441
Salehi, B., Venditti, A., M., Sharifi-Rad, D., Kregiel, J., Sharifi-Rad, Durazzo, A., et al. (2019). The therapeutic potential of apigenin. Int. J. Mol. Sci. 20:1305. doi: 10.3390/ijms20061305
Salmani, J. M. M., Zhang, X. P., Jacob, J. A., and Chen, B. A. (2017). Apigenin's anticancer properties and molecular mechanisms of action: recent advances and future prospectives. Chin. J. Nat. Med. 15, 321–329. doi: 10.1016/S1875-5364(17)30052-3
Sameiyan, E., Hayes, A. W., and Karimi, G. (2019). The effect of medicinal plants on multiple drug resistance through autophagy: a review of in vitro studies. Eur. J. Pharmacol. 852, 244–253. doi: 10.1016/j.ejphar.2019.04.001
Schatzkin, A., and Kelloff, G. (1995). Chemo- and dietary prevention of colorectal cancer. Eur. J. Cancer 31a, 1198–1204. doi: 10.1016/0959-8049(95)00138-9
Schulz-Heddergott, R., and Moll, U. M. (2018). Gain-of-Function (GOF) mutant p53 as actionable therapeutic target. Cancers 10:60188. doi: 10.3390/cancers10060188
Seldin, D. C., Lou, D. Y., Toselli, P., Landesman-Bollag, E., and Dominguez, I. (2008). Gene targeting of CK2 catalytic subunits. Mol. Cell Biochem. 316, 141–147. doi: 10.1007/s11010-008-9811-8
Sen, K., Banerjee, S., and Mandal, M. (2014). Reversal of Warburg effect by Apigenin and 5-Fluorouracil loaded dual drug liposomes result in enhanced colorectal chemotherapy. Cancer Res. 74(19 Suppl.):Abstract nr 4594. doi: 10.1158/1538-7445.AM2014-4594
Sen, K., Banerjee, S., and Mandal, M. (2019). Dual drug loaded liposome bearing apigenin and 5-Fluorouracil for synergistic therapeutic efficacy in colorectal cancer. Colloids Surf. B Biointerfaces 180, 9–22. doi: 10.1016/j.colsurfb.2019.04.035
Sen, K., and Mandal, M. (2013). Second generation liposomal cancer therapeutics: transition from laboratory to clinic. Int. J. Pharmaceut. 448, 28–43. doi: 10.1016/j.ijpharm.2013.03.006
Seo, H.-S., Choi, H-S., Kim, S-R., Choi, Y. K., Woo, S-M., Shin, I., et al. (2012). Apigenin induces apoptosis via extrinsic pathway, inducing p53 and inhibiting STAT3 and NFκB signaling in HER2-overexpressing breast cancer cells. Mol. Cell. Biochem. 366, 319–334. doi: 10.1007/s11010-012-1310-2
Seo, H.-S., Ku, J. M., Choi, H-S., Woo, J-K., Jang, B-H., Shin, Y. C., et al. (2014). Induction of caspase-dependent apoptosis by apigenin by inhibiting STAT3 signaling in HER2-overexpressing MDA-MB-453 breast cancer cells. Anticancer Res. 34, 2869–2882.
Seo, H. S., Jo, J. K., Ku, J. M., Choi, H. S., Choi, Y. K., Woo, J. K., et al. (2015a). Induction of caspase-dependent extrinsic apoptosis by apigenin through inhibition of signal transducer and activator of transcription 3 (STAT3) signalling in HER2-overexpressing BT-474 breast cancer cells. Biosci. Rep. 35:165. doi: 10.1042/BSR20150165
Seo, H. S., Ku, J. M., Choi, H. S., Woo, J. K., Jang, B. H., Go, H., et al. (2015b). Apigenin induces caspase-dependent apoptosis by inhibiting signal transducer and activator of transcription 3 signaling in HER2-overexpressing SKBR3 breast cancer cells. Mol. Med. Rep. 12, 2977–2984. doi: 10.3892/mmr.2015.3698
Shao, H., Jing, K., Mahmoud, E., Huang, H., Fang, X., and Yu, C. (2013). Apigenin sensitizes colon cancer cells to antitumor activity of ABT-263. Mol. Cancer Ther. 12, 2640–2650. doi: 10.1158/1535-7163.MCT-13-0066
Shuai, C., Zan, J., Qi, F., Wang, G., Liu, Z., Yang, Y., et al. (2019). nMgO-incorporated PLLA bone scaffolds: enhanced crystallinity and neutralized acidic products. Mater. Design 174, 107801–107810. doi: 10.1016/j.matdes.2019.107801
Shukla, S., Bhaskaran, N., Babcook, M. A., Fu, P., MacLennan, G. T., and Gupta, S. (2014a). Apigenin inhibits prostate cancer progression in TRAMP mice via targeting PI3K/Akt/FoxO pathway. Carcinogenesis 35, 452–460. doi: 10.1093/carcin/bgt316
Shukla, S., Fu, P., and Gupta, S. (2014b). Apigenin induces apoptosis by targeting inhibitor of apoptosis proteins and Ku70-Bax interaction in prostate cancer. Apoptosis 19, 883–894. doi: 10.1007/s10495-014-0971-6
Shukla, S., and Gupta, S. (2008). Apigenin-induced prostate cancer cell death is initiated by reactive oxygen species and p53 activation. Free Radic. Biol. Med. 44, 1833–1845. doi: 10.1016/j.freeradbiomed.2008.02.007
Shukla, S., and Gupta, S. (2010). Apigenin: a promising molecule for cancer prevention. Pharmaceut. Res. 27, 962–978. doi: 10.1007/s11095-010-0089-7
Shukla, S., Kanwal, R., Shankar, E., Datt, M., Chance, M. R., Fu, P., et al. (2015a). Apigenin blocks IKKα activation and suppresses prostate cancer progression. Oncotarget 6:31216. doi: 10.18632/oncotarget.5157
Shukla, S., Shankar, E., Fu, P., MacLennan, G. T., and Gupta, S. (2015b). Suppression of NF-κB and NF-κB-regulated gene expression by apigenin through IκBα and IKK pathway in TRAMP mice. PLoS ONE 10:e0138710. doi: 10.1371/journal.pone.0138710
Siegel, R. L., Miller, K. D., and Jemal, A. (2018). Cancer statistics, 2018. CA Cancer J. Clin. 68, 7–30. doi: 10.3322/caac.21442
Singh, P., Mishra, S. K., Noel, S., Sharma, S., and Rath, S. K. (2012). Acute exposure of apigenin induces hepatotoxicity in Swiss mice. PLoS ONE 7:e31964. doi: 10.1371/journal.pone.0031964
Singletary, K. (2000). Diet, natural products and cancer chemoprevention. J. Nutr. 130(2S Suppl.), 465s−466s. doi: 10.1093/jn/130.2.465S
Solarova, Z., Mojzis, J., and Solar, P. (2015). Hsp90 inhibitor as a sensitizer of cancer cells to different therapies. Int. J. Oncol. 46, 907–926. doi: 10.3892/ijo.2014.2791
Solmaz, S., Adan Gokbulut, A., Cincin, B., Ozdogu, H., Boga, C., Cakmakoglu, B., et al. (2014). Therapeutic potential of apigenin, a plant flavonoid, for imatinib-sensitive and resistant chronic myeloid leukemia cells. Nutr. Cancer 66, 599–612. doi: 10.1080/01635581.2014.894099
Srinivas, U. S., Tan, B. W. Q., Vellayappan, B. A., and Jeyasekharan, A. D. (2019). ROS and the DNA damage response in cancer. Redox Biol. 25:101084. doi: 10.1016/j.redox.2018.101084
Strouch, M. J., Milam, B. M., Melstrom, L. G., McGill, J. J., Salabat, M. R., Ujiki, M. B., et al. (2009). The flavonoid apigenin potentiates the growth inhibitory effects of gemcitabine and abrogates gemcitabine resistance in human pancreatic cancer cells. Pancreas 38, 409–415. doi: 10.1097/MPA.0b013e318193a074
Sun, J. W., Qiu, S., Yang, J. Y., Chen, X., and Li, H. X. (2020). Hsa_circ_0124055 and hsa_circ_0101622 regulate proliferation and apoptosis in thyroid cancer and serve as prognostic and diagnostic indicators. Eur. Rev. Med. Pharmacol. Sci. 24, 4348–4360. doi: 10.26355/eurrev_202004_21016
Sung, B., Chung, H. Y., and Kim, N. D. (2016). Role of apigenin in cancer prevention via the induction of apoptosis and autophagy. J. Cancer Prev. 21, 216–226. doi: 10.15430/JCP.2016.21.4.216
Tan, B. L., and Norhaizan, M. E. (2019). Curcumin combination chemotherapy: the implication and efficacy in cancer. Molecules 24:142527. doi: 10.3390/molecules24142527
Tan, S., Yamashita, A., Gao, S. J., and Kurisawa, M. (2019). Hyaluronic acid hydrogels with defined crosslink density for the efficient enrichment of breast cancer stem cells. Acta Biomater. 94, 320–329. doi: 10.1016/j.actbio.2019.05.040
Tang, A. Q., Cao, X. C., Tian, L., He, L., and Liu, F. (2015). Apigenin inhibits the self-renewal capacity of human ovarian cancer SKOV3-derived sphere-forming cells. Mol. Med. Rep. 11, 2221–2226. doi: 10.3892/mmr.2014.2974
Tang, D., Chen, K., Huang, L., and Li, J. (2017). Pharmacokinetic properties and drug interactions of apigenin, a natural flavone. Expert Opin. Drug Metabol. Toxicol. 13, 323–330. doi: 10.1080/17425255.2017.1251903
Telange, D. R., Patil, A. T., Pethe, A. M., Fegade, H., Anand, S., and Dave, V. S. (2017). Formulation and characterization of an apigenin-phospholipid phytosome (APLC) for improved solubility, in vivo bioavailability, and antioxidant potential. Eur. J. Pharm. Sci. 108, 36–49. doi: 10.1016/j.ejps.2016.12.009
Tempero, M. A., Malafa, M. P., Al-Hawary, M., Asbun, H., Bain, A., Behrman, S. W., et al. (2017). Pancreatic adenocarcinoma, version 2.2017, NCCN clinical practice guidelines in oncology. J. Natl. Compr. Canc. Netw. 15, 1028–1061. doi: 10.6004/jnccn.2017.0131
Tong, J., Shen, Y., Zhang, Z., Hu, Y., Zhang, X., and Han, L. (2019). Apigenin inhibits epithelial-mesenchymal transition of human colon cancer cells through NF-κB/Snail signaling pathway. Biosci Rep. 39:BSR20190452. doi: 10.1042/BSR20190452
Tong, X., Smith, K. A., and Pelling, J. C. (2012). Apigenin, a chemopreventive bioflavonoid, induces AMP-activated protein kinase activation in human keratinocytes. Mol. Carcinog. 51, 268–279. doi: 10.1002/mc.20793
Tsao, A. S., Kim, E. S., and Hong, W. K. (2004). Chemoprevention of cancer. CA Cancer J. Clin. 54, 150–180. doi: 10.3322/canjclin.54.3.150
Tseng, T. H., Chien, M. H., Lin, W. L., Wen, Y. C., Chow, J. M., Chen, C. K., et al. (2017). Inhibition of MDA-MB-231 breast cancer cell proliferation and tumor growth by apigenin through induction of G2/M arrest and histone H3 acetylation-mediated p21(WAF1/CIP1) expression. Environ. Toxicol. 32, 434–444. doi: 10.1002/tox.22247
Ujiki, M. B., Ding, X-Z., Salabat, M. R., Bentrem, D. J., Golkar, L., Milam, B., et al. (2006). Apigenin inhibits pancreatic cancer cell proliferation through G2/M cell cycle arrest. Mol. Cancer 5:76. doi: 10.1186/1476-4598-5-76
Varghese, E., Liskova, A., Kubatka, P., Samuel, S. M., and Büsselberg, D. (2020). Anti-angiogenic effects of phytochemicals on miRNA regulating breast cancer progression. Biomolecules 10:191. doi: 10.3390/biom10020191
Vega-Rubín-de-Celis, S. (2019). The role of beclin 1-dependent autophagy in cancer. Biology 9:10004. doi: 10.3390/biology9010004
Vela, L., and Marzo, I. (2015). Bcl-2 family of proteins as drug targets for cancer chemotherapy: the long way of BH3 mimetics from bench to bedside. Curr. Opin. Pharmacol. 23, 74–81. doi: 10.1016/j.coph.2015.05.014
Wan, L., Guo, C., Yu, Q., Li, Y., Wang, X., Wang, X., et al. (2007). Quantitative determination of apigenin and its metabolism in rat plasma after intravenous bolus administration by HPLC coupled with tandem mass spectrometry. J. Chromatogr. B 855, 286–289. doi: 10.1016/j.jchromb.2007.05.007
Wang, B., and Zhao, X. H. (2017). Apigenin induces both intrinsic and extrinsic pathways of apoptosis in human colon carcinoma HCT-116 cells. Oncol. Rep. 37, 1132–1140. doi: 10.3892/or.2016.5303
Wang, I. K., Lin-Shiau, S. Y., and Lin, J. K. (1999). Induction of apoptosis by apigenin and related flavonoids through cytochrome c release and activation of caspase-9 and caspase-3 in leukaemia HL-60 cells. Eur. J. Cancer 35, 1517–1525. doi: 10.1016/S0959-8049(99)00168-9
Wang, M., Firrman, J., Liu, L., and Yam, K. (2019a). A review on flavonoid apigenin: Dietary intake, ADME, antimicrobial effects, and interactions with human gut microbiota. BioMed Res. Int. 2019:7010467. doi: 10.1155/2019/7010467
Wang, M., Firrman, J., Zhang, L., G., Arango-Argoty, Tomasula, P., Liu, L., et al. (2017). Apigenin impacts the growth of the gut microbiota and alters the gene expression of Enterococcus. Molecules 22:1292. doi: 10.3390/molecules22081292
Wang, Q., Zeng, P., Liu, Y., Wen, G., Fu, X., and Sun, X. (2015). Inhibition of autophagy ameliorates atherogenic inflammation by augmenting apigenin-induced macrophage apoptosis. Int. Immunopharmacol. 27, 24–31. doi: 10.1016/j.intimp.2015.04.018
Wang, S., Liu, X., Khan, A. A., Li, H., Tahir, M., Yan, X., et al. (2020). miR-216a-mediated upregulation of TSPAN1 contributes to pancreatic cancer progression via transcriptional regulation of ITGA2. Am. J. Cancer Res. 10, 1115–1129.
Wang, Z., Tian, Y., Zhang, H., Qin, Y., Li, D., Gan, L., et al. (2016). Using hyaluronic acid-functionalized pH stimuli-responsive mesoporous silica nanoparticles for targeted delivery to CD44-overexpressing cancer cells. Int. J. Nanomed. 11:6485. doi: 10.2147/IJN.S117184
Wang, Z. C., Huang, F. Z., Xu, H. B., Sun, J. C., and Wang, C. F. (2019b). MicroRNA-137 inhibits autophagy and chemosensitizes pancreatic cancer cells by targeting ATG5. Int. J. Biochem. Cell Biol. 111, 63–71. doi: 10.1016/j.biocel.2019.01.020
Way, T.-D., Kao, M-C., and Lin, J.-K. (2004). Apigenin induces apoptosis through proteasomal degradation of HER2/neu in HER2/neu-overexpressing breast cancer cells via the phosphatidylinositol 3-kinase/Akt-dependent pathway. J. Biol. Chem. 279, 4479–4489. doi: 10.1074/jbc.M305529200
Wen, X., and Klionsky, D. J. (2019). At a glance: a history of autophagy and cancer. Semin. Cancer Biol. 66, 3–11. doi: 10.1016/j.semcancer.2019.11.005
Woo, S. M., Seo, S. U., Kubatka, P., Min, K-j., and Kwon, T. K. (2019). Honokiol enhances TRAIL-mediated apoptosis through STAMBPL1-induced survivin and c-FLIP degradation. Biomolecules 9:838. doi: 10.3390/biom9120838
Wu, D.-G., Yu, P., Li, J-W., Jiang, P., Sun, J., Wang, H-Z., et al. (2014). Apigenin potentiates the growth inhibitory effects by IKK-β-mediated NF-κB activation in pancreatic cancer cells. Toxicol. Lett. 224, 157–164. doi: 10.1016/j.toxlet.2013.10.007
Xu, D., Yuan, H., Meng, Z., Yang, C., Li, Z., Li, M., et al. (2020). Cadherin 13 inhibits pancreatic cancer progression and epithelial-mesenchymal transition by Wnt/β-catenin signaling. J. Cancer 11, 2101–2112. doi: 10.7150/jca.37762
Xu, M., Wang, S., Song, Y., Yao, J., Huang, K., and Zhu, X. (2016). Apigenin suppresses colorectal cancer cell proliferation, migration and invasion via inhibition of the Wnt/β-catenin signaling pathway. Oncol. Lett. 11, 3075–3080. doi: 10.3892/ol.2016.4331
Xu, W., Qian, J., Hou, G., Suo, A., Wang, Y., Wang, J., et al. (2017). Hyaluronic acid-functionalized gold nanorods with pH/NIR dual-responsive drug release for synergetic targeted photothermal chemotherapy of breast cancer. ACS Appl. Mater. Interfaces 9, 36533–36547. doi: 10.1021/acsami.7b08700
Yan, B., Cheng, L., Jiang, Z., Chen, K., Zhou, C., Sun, L., et al. (2018). Resveratrol inhibits ROS-promoted activation and glycolysis of pancreatic stellate cells via suppression of miR-21. Oxid. Med. Cell Longev. 2018:1346958. doi: 10.1155/2018/1346958
Yan, X., Qi, M., Li, P., Zhan, Y., and Shao, H. (2017). Apigenin in cancer therapy: anti-cancer effects and mechanisms of action. Cell Biosci. 7:50. doi: 10.1186/s13578-017-0179-x
Yang, H., Villani, R. M., Wang, H., Simpson, M. J., Roberts, M. S., Tang, M., et al. (2018). The role of cellular reactive oxygen species in cancer chemotherapy. J. Exp. Clin. Cancer Res. 37:266. doi: 10.1186/s13046-018-0909-x
Yang, M. H., Kim, J., Khan, I. A., Walker, L. A., and Khan, S. I. (2014). Nonsteroidal anti-inflammatory drug activated gene-1 (NAG-1) modulators from natural products as anti-cancer agents. Life Sci. 100, 75–84. doi: 10.1016/j.lfs.2014.01.075
Yang, Y., and Klionsky, D. J. (2020). Autophagy and disease: unanswered questions. Cell Death Differentiation 27, 858–871. doi: 10.1038/s41418-019-0480-9
Yin, Z., Zhou, Y., Ma, T., Chen, S., Shi, N., Zou, Y., et al. (2020). Down-regulated lncRNA SBF2-AS1 in M2 macrophage-derived exosomes elevates miR-122-5p to restrict XIAP, thereby limiting pancreatic cancer development. J. Cell Mol. Med. 24, 5028–5038. doi: 10.1111/jcmm.15125
Zambrano, A., Molt, M., Uribe, E., and Salas, M. (2019). Glut 1 in cancer cells and the inhibitory action of resveratrol as a potential therapeutic strategy. Int. J. Mol. Sci. 20:3374. doi: 10.3390/ijms20133374
Zhang, J., Liu, D., Huang, Y., Gao, Y., and Qian, S. (2012). Biopharmaceutics classification and intestinal absorption study of apigenin. Int. J. Pharm. 436, 311–317. doi: 10.1016/j.ijpharm.2012.07.002
Zhang, L., Cheng, X., Gao, Y., Zheng, J., Xu, Q., Sun, Y., et al. (2015). Apigenin induces autophagic cell death in human papillary thyroid carcinoma BCPAP cells. Food Funct. 6, 3464–3472. doi: 10.1039/C5FO00671F
Zhang, L., Tao, X., Fu, Q., Ge, C., Li, R., Li, Z., et al. (2019). Curcumin inhibits cell proliferation and migration in NSCLC through a synergistic effect on the TLR4/MyD88 and EGFR pathways. Oncol. Rep. 42, 1843–1855. doi: 10.3892/or.2019.7278
Zhang, X. (2019). Interactions between cancer cells and bone microenvironment promote bone metastasis in prostate cancer. Cancer Commun. 39:76. doi: 10.1186/s40880-019-0425-1
Zhao, G., Han, X., Cheng, W., Ni, J., Zhang, Y., Lin, J., et al. (2017a). Apigenin inhibits proliferation and invasion, and induces apoptosis and cell cycle arrest in human melanoma cells. Oncol. Rep. 37, 2277–2285. doi: 10.3892/or.2017.5450
Zhao, T., He, Y., Chen, H., Bai, Y., Hu, W., and Zhang, L. (2017b). Novel apigenin-loaded sodium hyaluronate nano-assemblies for targeting tumor cells. Carbohydr. Polym. 177, 415–423. doi: 10.1016/j.carbpol.2017.09.007
Zheng, H., Yang, Y., Hong, Y. G., Wang, M. C., Yuan, S. X., Wang, Z. G., et al. (2019). Tropomodulin 3 modulates EGFR-PI3K-AKT signaling to drive hepatocellular carcinoma metastasis. Mol. Carcinog. 58, 1897–1907. doi: 10.1002/mc.23083
Zheng, J. H., Viacava Follis, A., Kriwacki, R. W., and Moldoveanu, T. (2016). Discoveries and controversies in BCL-2 protein-mediated apoptosis. FEBS J. 283, 2690–2700. doi: 10.1111/febs.13527
Zhou, C., Qian, W., Ma, J., Cheng, L., Jiang, Z., Yan, B., et al. (2019). Resveratrol enhances the chemotherapeutic response and reverses the stemness induced by gemcitabine in pancreatic cancer cells via targeting SREBP1. Cell Prolif. 52:e12514. doi: 10.1111/cpr.12514
Zhou, Z., Tang, M., Liu, Y., Zhang, Z., Lu, R., and Lu, J. (2017). Apigenin inhibits cell proliferation, migration, and invasion by targeting Akt in the A549 human lung cancer cell line. Anticancer Drugs 28, 446–456. doi: 10.1097/CAD.0000000000000479
Zhu, L.-P., He, Y.-J., Hou, J.-C., Chen, X., Zhou, S.-Y., Yang, S.-J., et al. (2017). The role of circRNAs in cancers. Biosci. Rep. 37:750. doi: 10.1042/BSR20170750
Zhu, Y., Wu, J., Li, S., Wang, X., Liang, Z., Xu, X., et al. (2015). Apigenin inhibits migration and invasion via modulation of epithelial mesenchymal transition in prostate cancer. Mol. Med. Rep. 11, 1004–1008. doi: 10.3892/mmr.2014.2801
Keywords: apigenin, pancreatic cancer, oxidative stress, apoptosis, therapy
Citation: Ashrafizadeh M, Bakhoda MR, Bahmanpour Z, Ilkhani K, Zarrabi A, Makvandi P, Khan H, Mazaheri S, Darvish M and Mirzaei H (2020) Apigenin as Tumor Suppressor in Cancers: Biotherapeutic Activity, Nanodelivery, and Mechanisms With Emphasis on Pancreatic Cancer. Front. Chem. 8:829. doi: 10.3389/fchem.2020.00829
Received: 24 February 2020; Accepted: 05 August 2020;
Published: 15 October 2020.
Edited by:
Luis Álvarez de Cienfuegos, University of Granada, SpainReviewed by:
Clemens Zwergel, Sapienza University of Rome, ItalyEdson Roberto Silva, University of São Paulo, Brazil
Copyright © 2020 Ashrafizadeh, Bakhoda, Bahmanpour, Ilkhani, Zarrabi, Makvandi, Khan, Mazaheri, Darvish and Mirzaei. This is an open-access article distributed under the terms of the Creative Commons Attribution License (CC BY). The use, distribution or reproduction in other forums is permitted, provided the original author(s) and the copyright owner(s) are credited and that the original publication in this journal is cited, in accordance with accepted academic practice. No use, distribution or reproduction is permitted which does not comply with these terms.
*Correspondence: Maryam Darvish, maryam_darvish@yahoo.com; Hamed Mirzaei, mirzaei-h@kaums.ac.ir; h.mirzaei2002@gmail.com
†These authors have contributed equally to this work