- 1Department of Materials Science, Lomonosov Moscow State University, Moscow, Russia
- 2Department of Chemistry, Lomonosov Moscow State University, Moscow, Russia
Widely spread crystal lattices of perovskites represent a natural flexible platform for chemical design of various advanced functional materials with unique features. An interplay between chemical bonding, defects and crystallochemical peculiarities makes the perovskite structure a “LEGO designer” utilizing natural features of chemical elements of the renowned Mendeleev's Periodic Table (PTE) celebrating its 150-year anniversary. In this mini-review, crystal chemistry and bonding features, physical and functional properties, preparation methods and tuning functional properties with periodicity “tools” of the PTE will be exemplified for legendary families of high-temperature superconductive cuprates, colossal magnetoresistive manganites and hybrid lead halides for a new generation of solar cells.
Introduction
The perovskite lattice (Figure 1) represents one of the most common motifs of solid phases and, also, is quite typical for the famous families of advanced functional materials including photocatalysis, electrocatalysts and fuel cell components, insertion cathodes of chemical power sources, high—temperature superconductors, multyferroics, magnetic and magnetoresistive materials, materials for solar cell energy and photoluminescence (Ahn et al., 2004; Haugan et al., 2004; Gao et al., 2010; Du et al., 2011, 2013; Jiang et al., 2012; Osterloh, 2013; Frost et al., 2014; Song et al., 2015; De Roo et al., 2016; Weidman et al., 2016; Hwang et al., 2017, 2019; Wang et al., 2019; Hao et al., 2020). At the same time only three families of perovskite-based functional materials have attracted major interest in view of prospects in developing mass-production technologies and practical applications.
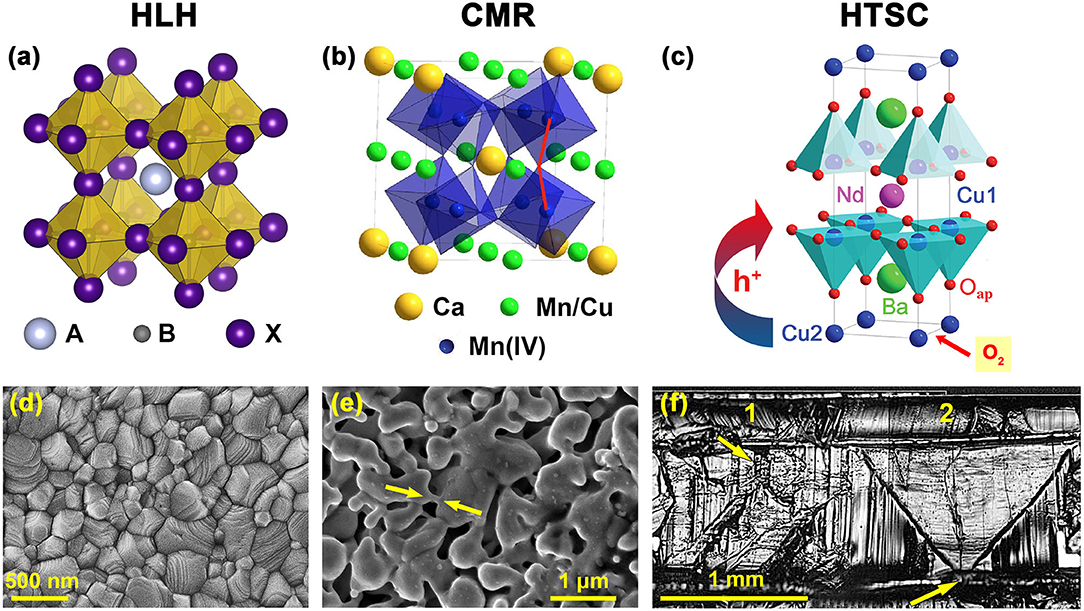
Figure 1. Crystal structures (a,b,c) and, respectively, typical morphologies from a top view of films or coatings of solution–derived HLH perovskites (d), ASP—deposited CMR manganites (e) and melt—processed HTSC cuprates (f). The A, B, X for the structure of HLH perovskite (a) represent a large inorganic or organic cation (A), lead (B) and halogen (X), respectively. The structure of CMR manganites (b) is shown for a special family of the CaCu3Mn4O12 solid solution. The HTSC is represented by the most famous family of REE-barium cuprates (NdBa2Cu3O6+x), the arrow symbolizes the hole (h+) transfer from Cu(2) ions in the charge reservoir to Cu(1) in superconducting plains. The white arrows show intergrain necks (e) or nucleation sites of crystallization for two adjacent millimeter–sized HTSC grains on a graphotexturing substrate (f). Note, the morphology shown for CMR manganites is not acceptable for HLH or HTSC perovskites while the HLH microstructure would not be suitable for HTSCs.
Colossal MagnetoResistive (CMR) manganites (Figure 1b) were discovered in the 1950's (Van Santen and Jonker, 1950; Volger, 1954) and rediscovered in the 1990's (von Helmolt et al., 1993). In 2007, a Nobel Prize in Physics was awarded for the same effect observed in layered heterostructures demonstrating the Giant MagnetoResistance based on the fundamentally important effect of control of electron scattering by spin polarization (Fert et al., 1995). High–Temperature Super Conducting (HTSC) cuprates (Figure 1c) have been found in 1986 (Bednorz and Müller, 1986) and have been awarded next year with the Nobel Prize in Physics which is one of a total of six Nobel Prizes for superconductivity. The most recent high-impact perovskites (Figure 1a) have been suggested for Perovskite Photovoltaics presented solely by Hybrid Lead Halides applied for solar cells in 2009 by Tsutomi Miyasaka (Kojima et al., 2009), developed further in 2012 by Michael Grätzel with essential works known from Henry Snaith, Nam-Gyu Park, and others (Grätzel, 2014; Park et al., 2016; Eperon et al., 2017; Li et al., 2017, 2018; Gao et al., 2018; Leijtens et al., 2018; Snaith and Hacke, 2018; Fu et al., 2019; Nayak et al., 2019). They represent a new generation of solar cell materials overcoming easily the records of DSSC devices with chemically stable and quite effective tetrapyrolic sensitizers and approaching closely the best values of effectiveness of silicon (Bottari et al., 2010; Li and Diau, 2013; Mathew et al., 2014; Martynov et al., 2019; Abdulaeva et al., 2020).
These families of perovskite-like materials represent three conceptually different areas of materials research for advanced electronics and alternative energy. The unique behavior of these materials originates of chemical element features composing the phases, different metal—non-metal bonds resulting in different target physical properties and demanding, in turn, quite special optimal morphologies of the materials under the question (Figure 1). The latter, obviously, can be achieved by material-specific production techniques being the limiting factors of the materials implementation. It is risky to compare all these almost dissimilar materials (Table 1) but there is the only valuable aspect of such a story that is closely related to the key chemical approaches utilized for enhancing their optimal morphologies. This material—related analysis seems to be useful for a rational design and future progress in preparation techniques toward the development of the perovskite—based devices with record characteristics. This purpose is highlighted in the current mini-review to shape the research directions of prospective chemical preparation routes based on the PTE peculiarities of the respective elements.
Structural Features of Perovskites vs. Composing Element Peculiarities
None of the discussing materials demonstrate ideal ABX3 perovskite structures where A—a larger central cation, B—a smaller cation octahedrally surrounded by X anions (Figure 1). Moreover, different HTSC, CMR, HLH families and homologs, intergrown or none-perovskite structures are well-known (Zhang et al., 1996; Tretyakov and Goodilin, 2000; Tretyakov et al., 2004; Attfield, 2011; Lee et al., 2014; Ovcharov et al., 2019). However, their “classical” representatives are stack to the simplest perovskite lattice (Table 1, Figure 1). HTSC cuprates and CMR manganites, according to the Pearson's formalism1, are the compounds of hard acids (cations) from the d-block (Cu and Mn) and a hard base O2− from the p-block. On the contrary, the lead halide perovskites are composed from a soft acid Pb2+ and a soft base I− from the p-block of PTE. These combinations result in phases which are more stable but different. The hard acid–hard base compounds with stronger interactions of smaller non-polarizable ions in the lattice exist in air up to the temperatures of ca. 1000 (HTSC)−1,300°C (CMR) while the HLH soft acid–soft base perovskites with large polarizable ions do not survive, expectedly, above 120–150°C but easily form solutions and adducts with various donor solvents (Fateev et al., 2018) thus presenting the most attractive solvent-based deposition technologies of solar cell production (Park, 2016). HLH is a unique compound family made of the heaviest and the largest non-radioactive elements of PTE.
HTSC cuprates (Figure 1c) and CMR manganites (Figure 1b) are mixed—valent phases with a large non-stoichiometry of X—anion for HTSC or A—cation in the case of CMR phases (Tretyakov and Goodilin, 2000; Pomerantseva et al., 2004; Tretyakov et al., 2004). The HLH perovskites demonstrate a moderate or small X-site non-stoichiometry and, formally, fixed oxidation states of lead and halogens. The mixed-valent states of HTSC cuprates and CMR manganites are achieved by means of two chemically different approaches. Oxygen non-stoichiometry is the major factor used for oxidation of the HTSC phases with molecular oxygen (Figure 1) and that leads to an increase of the copper oxidation state, for example, from +1/+2 for REEBa2Cu3O6 (REE—Rare Earth Elements) to +2 and exotic Cu(III) for REEBa2Cu3O7 (Shiohara and Goodilin, 2000; Tretyakov and Goodilin, 2000). CMR manganites use heterovalent doping of the A—cation rather than varying oxygen content to provide the needed balance of Mn(III) / Mn(IV) in the structure. Normally, the B—site substitution, especially heterovalent, provides no drastic improvement of functional properties and, often, deteriorates them. For example, <1–5 at % of Zn, Mg, and other elements substituting copper in REEBa2Cu3O7 lead to a half reduction of the superconductivity transition temperature, similar substitutions of manganese in CMR manganites are also risky, have no linear dependence on their concentration and normally are not effective for properties improvements. For HLH (Figure 1a), lead substitution with tin, bismuth etc. usually results in decreasing functional parameters while the X-site substitution with mixed halide ions is often useful for fine tuning of the physical and thermodynamic properties. The X-site substitution, even heterovalent (like fluorine), is applied rarely to tune the properties of HTSCs and CMR materials but it could not be considered as primary method of their target chemical modifications.
The A-site cation plays an important but a secondary role. The A-cation usually affects no physical properties but it is primarily needed to stabilize the structure electrostatically and geometrically since the ionic radii of this cation is counted in the famous Goldshmidt tolerance factor predicting the overall structure stability. Noticeably, a 12–13% decrease of the REE size due to the lanthanide contraction effect results in the REEBa2Cu3O7 melting temperature falling by about 120°C (Shiohara and Goodilin, 2000), from 1,085°C (Nd) down to 965°C (Yb); this effect is much weaker for mixed REE–AEE CMR manganites (AEE—Alkali Earth Elements). In the case of HLH, the largest purely inorganic cation in the PTE, Cs+, seems to be still too small to solely stabilize the HLH perovskite structure near room temperature thus demanding a larger cation, such as methylammonium and formamidinium (Travis et al., 2016). The latter makes the HLH perovskites belong to the hybrid, organic–inorganic, phases and therefore this feature entirely changes their chemical properties and preparation techniques. A further increase of the A-cation size or changing its geometry produces low-dimensional HLH phases with complex structures (Smith et al., 2018); thus a set of available cations to form the HLH perovskites is quite limited. The consequences of the asymmetry of such a “hybrid” cation include local structural distortions and, for some solid solutions, a possibility of spinodal decomposition which is useless for the HLH perovskites. Oppositely, the spinodal decomposition is a remarkable phenomenon for the A-site substituted solid solutions based on the HTSC cuprates (Petrykin et al., 2000; Shiohara and Goodilin, 2000) since the demixing generates compositional nanofluctuations acting as effective pinning centers and thus resulting in record critical currents under magnetic fields. A more complex structure of HTSCs and CMR manganites could also yield antisites in the structure of perovskites resulting in the preparation “prehistory” effects (Petrykin et al., 2000). In the case of HLH perovskites, a mixture of various A-cations are routinely applied for the entropy-driven stabilization (Yi et al., 2016).
The framework of corner-sharing octahedra BX6 of the perovskites generates the main application-related properties of these materials (Tretyakov et al., 2004). In this contest, The Jahn–Teller effect, being quite typical for Mn3+ and Cu2+, causes frustrated structures, spin waves for manganites (Pomerantseva et al., 2004) or result in drastic structural distortions for HTSCs. In the case of REEBa2Cu3O7, the structure (Figure 1c) is composed of three perovskite-like oxygen deficient intergrown blocks (Shiohara and Goodilin, 2000; Tretyakov and Goodilin, 2000). The two of them containing barium and empty oxygen vacancies near copper (Cu(1)O2—BaO(ap)—Cu(2)Ox) operate as charge reservoirs accumulating holes upon copper oxygenation and oxygen content growth, VO** + 1/2 O2 = OOX + 2 h*. It is a direct representation of the Jahn–Teller effect that there is no octahedra with copper and oxygen in this structure but, instead, Cu(1) is included into flat superconducting (SC) planes CuO2 and have a five-fold pyramidal coordination counting also the “apical” oxygen O(ap) in the BaO “layer.” The second type of copper, Cu(2), possesses a two-fold linear coordination for the tetragonal oxygen-disordered REEBa2Cu3O6 compound or rhombs for the superconducting REEBa2Cu3O7 orthorhombic phase with ordered residual oxygen vacancies. The central CuO2-REE–CuO2 block contains two flat superconducting planes and REE3+ cations. Upon oxygenation, holes are concentrated in the charge reservoir blocks and are transferred then to the superconducting plains CuO2 by shifting the apical oxygen O(ap) from Cu(2) toward Cu(1). A critical concentration of holes in the SC plains gives bosons, the BCS pairs, if cooled below a SC critical temperature Tc. Actually, such a crystal architecture makes HTSCs cuprates highly anisotropic layered compounds originated of perovskites.
The flatter the SC plains, the higher Tc of HTSCs, otherwise overlapping the d-orbitals of Cu(1) and p-orbitals of oxygen is deteriorated. In the case of CMR manganites and HLH perovskites, no deep modifications of the perovskite motif are observed (Tretyakov et al., 2004). Moreover, the Mn–O–Mn chains in the structure of CMR phases (Figure 1b) have to be linear with the angle between Mn3+, O2− and Mn4+ close to 180° for effective overlapping of the respective dMn and pO orbitals corresponding each other by symmetry (Babushkina et al., 1998; Pomerantseva et al., 2004). Due to the antiferromagnetic, double exchange in these linear fragments, electrons may transfer from Mn3+ to Mn4+ via the linking oxygen. To reduce the electrical resistance of the phase, the electron carriers should be correlated or spin–polarized by an external magnetic field in different parts of CMR manganite and grains which results in the negative magnetoresistance effect. The overlapping of the s, p-orbitals of iodine and lead in HLH semiconducting phases also provides effective pathways for charge transfer however both hole and electron carriers are generated by the photovoltaic effect utilizing electron density redistribution between the s- and p–orbitals of Pb2+ and p–orbitals of I− within the Pb-I-Pb framework (Table 1). The HLH phases seem to be mostly “tolerant” to various defects (Meggiolaro et al., 2018) as not typical for classical semiconductors. Among others, the HLH perovskites seem to be the chemically and physically simplest phases (Figure 1a) with no peculiarities caused by defect ordering, heterovalent substitution or spin-correlated phenomena. The noted peculiarities of the discussed frameworks pre-determine, to a large extent, both the morphology and production schemes of the final materials and devices.
Morphologies, Microstructures and Relevant Processing Techniques
Nowadays, the perovskite-like phases under discussion are mostly used as 2D polycrystalline materials (thin films and heterostuctures) with already rare inventions of their 3D ceramic or single crystalline forms. As a polycrystalline matter for advanced practical applications, each of the materials requires its own optimal combination of general morphological parameters like crystalline grain size, orientation, thickness, uniformity and the organization of intergrain boundaries since the required different morphologies of the materials ensure the achievement of record functional properties. All the HTSCs, CMR and HLH materials are prominent in low-current applications, sensing devices and smart circuits for information technologies, communication, and microelectronics. HTSCs and CMR materials are involved in applications as magnetic field sensors, in both the cases, the artificial or natural organization of grain boundaries play an extraordinary role. The most known and the most magnetic field sensitive devices are SQUID magnetometers and tomographs utilizing the quantum Josephson's effect for a special gap / boundary architecture within a superconductor (Colclough et al., 1987). CMR materials for spintronics utilize artificial junctions to operate with spin-polarized carriers created by a magnetic field in spin valves and other elements of spintronics (Yang et al., 2019); in the simple case of magnetic sensors, grain boundaries within CMR manganites play a major role for target variation of electrical conductivity. It should be noted that, oppositely, multiple grain boundaries play a negative role for HTSCs even in those low-current applications. HLH semiconducting materials demonstrate a photovoltaic effect leading to generation of carriers for conversion of solar energy into electricity (Grätzel, 2014; Chen et al., 2017; Eperon et al., 2017). In such a case, grain boundaries are not a positive factor since they could quench and trap the carriers reducing the operational effectiveness although they are not really used for the control of a transport current. Vice versa, HLH materials can effectively generate light by converting electrical energy in light-emitting devices and quantum dots under low voltages and low currents (De Roo et al., 2016; Fu et al., 2019). HTSCs are the only type of materials in this group requiring high current applications demanded for industrial transport of electricity, industrial current limiters or generation of record magnetic fields in energy generation or transport systems (Bednorz, 2019; Dong et al., 2019).
Thus, HTSCs, CMR manganites, HLH perovskites demand three different approaches to achieve a proper morphology (Figure 1). For important devices utilizing these three perovskites, thin films, heterostructures or sandwiched structures have to be deposited (Zhang et al., 1996; Shiohara and Goodilin, 2000; Tretyakov and Goodilin, 2000; Tretyakov et al., 2004; Snaith and Hacke, 2018). CMR manganites (Pomerantseva et al., 2004) operate with a relative change of resistance under applied magnetic fields and this demands lateral transport of weak electrical currents while intergrain boundaries become highly important due to the effect of tunneling magnetoresistance (TMS). This means that CMR manganites would require a uniform thickness of the films but not necessarily their single crystallinity, polycrystalline CMR films seem to have some advantages thus this type of perovskites post the weakest requirements to their microstructure (Figure 1e). HTSC cuprate films below 1 μm in thickness, preserving the epitaxial control over the biaxial texturing, are necessary for spreading a lateral critical current for long distances (RABiTS tapes, rolling-assisted-biaxially-textured-substrate, and other “second generation” HTSCs) but its values exceed a fantastic level of 107 A/cm2 which is achievable by no other known materials. Under such a large current, non-uniformity of any kind or “weak links” between the grains result immediately in “hot spots” with huge local overheating leading finally to thermal destruction of the films. Therefore, HTSCs are extraordinarily sensitive to their microstructure demanding, at least, large grain and biaxially textured (epitaxial) films with clean grain boundaries, fully transparent for large values of electric current below Tc (Figure 1f). The HLH perovskites remain comparatively tolerant to microstructural requirements and occupy a position in between CMR and HTSC materials since they demand, due to an extraordinary large extinction coefficient, only 100–300 nm thin and uniform light absorbing layers with no pin holes to prevent shunting the circuit. This is demanded by the typical operational geometry of the new generation of solar cells with movement of negative and positive charge carriers across the sandwiched layers owing to the photovoltaic effect. The mean grain size of HTSC films would exceed millimeters (Figure 1f), the same typical parameter for CMR films falls into the submillimeter range (Pomerantseva et al., 2004). In contrast, HLH films with the best optoelectronic properties (Figure 1d) possess the grains of micrometer sizes and demand no perfect in-plain orientation although such films should contain no carrier traps at the boundaries (Zhang et al., 2016; Shlenskaya et al., 2018).
No real applications are known so far for single crystals of the noted perovskites although their effective grown techniques are developed successfully (Goodilin et al., 1997, 1998; Shiohara and Goodilin, 2000; Zhumekenov et al., 2018). Probably, some of them seem to be prospective for light-emitting devices, photodetectors and X-ray detectors (Wei and Huang, 2019; Murali et al., 2020). As for whiskers, they are not usually single crystalline, probably pseudomorphic (Petrov et al., 2017b), and are not yet involved in real applications.
HTSCs stand along among this group because of the second application domain related to large grain textured ceramics for industry like magnetic transport levitation, motors and generators (Tretyakov et al., 2004). In such ceramics, the same principle of biaxial texturing remains as in the case of HTSC thin films. Additionally, pinning centers of SC Abrikosov's vortexes are required for the ceramics however they are self-generated due to the developed processing schemes discussed below. At the same time, thin film solutions related to the so-called second generation of HTSCs are being developed to replace almost all high-current bulk materials.
Special requirements of achieving optimal morphologies lead to the wide elaboration of preparation techniques of these families of perovskite-related materials. In particular, thin film deposition and soft chemistry approaches are not unique and well-developed for all the discussing perovskite systems including, generally, different thermal, electron beam evaporation, laser ablation, CVD or MOCVD approaches, sol-gel and spin-coating techniques, aerosol spray pyrolysis, even graphoepitaxy; freeze drying is effective for preparation of some type of precursor particles (Tretyakov and Goodilin, 2000; Goodilin et al., 2002; Tretyakov et al., 2004; Gao et al., 2018; Snaith and Hacke, 2018; Nayak et al., 2019). Thin film deposition is quite complicated, but is already well-established, for the second generation of flexible tapes of HTSCs since this procedure includes unavoidably the metallic substrate texturing, coating with several buffer layers with precisely controlled microstructures followed by epitaxial growth of HTSC film, shunting and protective layers. The complexity of this procedure for finely tuned morphologies gave birth to special modifications of film deposition techniques like IBAD (ion beam assisted deposition) or ISD (inclined substrate deposition). Compared to that, HLH film deposition is much simpler and includes routine stages of deposition without controlling epitaxy relations. The specificity of those scale—up procedures for HLH is the ability to use nearly room temperatures and common solvents within the “wet” techniques like slot—die, blade-, spin-coating, ink–jet printing, screen printing etc (Li et al., 2018). Those methods have been surely tested for HTSC but they showed lower effectiveness compared to CVD or PVD because of much stricter requirement to the microstructure and higher phase conversion temperatures.
Solvent-deposition techniques of functional films seem to be oversimplified by common thinking however they are rather complicated by the phenomena of new phase formation, phase transformation, mass and heat transfer in terms of solvent evaporation, decomposition of intermediate hydrates and hydrolysis products (HTSC, CMR) or complex solvent adducts (HLH) resulting often in loosing morphological, chemical uniformity, shrinkage, crack formation etc (Petrov et al., 2017c; Shlenskaya et al., 2018). This all leads to the successful search for novel solvent-free, for example, melt-based, preparation approaches. Only two families of the perovskites utilize effectively melt preparation techniques—HTSCs and HLHs (Figure 1). In the first case, melt preparation is one of the basic and well-developed approaches (Table 1) while HLH phases have demonstrated such a potential only recently (Petrov et al., 2017a; Turkevych et al., 2019).
HTSCs undergo peritectic decomposition which can be exemplified for REEBa2Cu3O7 as “melting”: REEBa2Cu3O7 = REE2BaCuO5 + L + O2 where L–melt containing Cu(I) and Cu(2), REE2BaCuO5–a properitectic phase (Shiohara and Goodilin, 2000; Tretyakov and Goodilin, 2000). The reversal transformation depends on heat transfer, oxygen partial pressure and REE concentration in the melt. The latter is quite important because the melt is barium- and Cu(I)- rich and REE2BaCuO5 particles is the only source of REE. It actually gives no heterogeneous nucleation sites, as might be expected, it is proven that REE2BaCuO5, instead, provides a higher REE3+ content in the vicinity of the properitectic particles leading to homogeneous nucleation of REEBa2Cu3O7. Such a mechanism has direct consequences in terms of appearing several groups of melt processing (Table 1). All of these methods are already finally developed and therefore this system gives a full set of possible examples of effective melt preparation routes. In particular, most of the methods utilize, expectedly, melting and cooling regimes. Another group applies an isothermal controllable variation of pO2, as a volatile component, to crystallize the melt. Finally, some methods apply a spatial gradient of REE to provide the needed biaxial texture of HTSCs. The size distribution of the properitectic phase seems to be quite important since it determines the key morphological features of melt—processed large grain HTSC ceramics as well as the generation of effective pinning centers for type II superconductors. Therefore a special degree of freedom is used to vary this parameter closely connected with shifting the precursors toward more non-equilibrium states, in particular, by replacing the final product REEBa2Cu3O7 undergoing melting by imitators of the quenched peritectic melt, like REE2BaCuO5 and Cu(II) cuprate mixtures, or REE2O3 and Cu(I) cuprite mixtures, as observed upon decomposition of the REEBa2Cu3O7 phase at 1,300–1,400°C and quenching (Tretyakov et al., 2004). The best methods already known for HTSCs melt processing (Table 1) utilize both, the shift to non-equilibrium mixtures and the controllable change of pO2 (Tretyakov and Goodilin, 2000).
It is hard to expect that the HLH perovskites will undergo the same complex evolution of melt processing techniques since this family requires much simpler microstructures with no biaxial texturing, inclusions of secondary phases or large grains. At the same time, at least two analogous approaches are already suggested thus manifesting a start of development of HLH melt processing as a trend. Unfortunately, HLHs undergo irreversible decomposition with loosing highly volatile components like iodine and methylamine upon melting (Boyd et al., 2019). In this contest, traditional melt processing, even despite of comfortable melting points around 140–170°C is not applicable. At the same time, an excess of iodine or methylamine forms a self-flux - the room-temperature melts allowing crystallization of HLH perovskites from those liquids (Chen et al., 2017; Petrov et al., 2019). The recently developed and quite promising RP—MAGIC approach (Table 1) utilizes reactionary polyiodide melts (RPM) to convert thin layers of metallic lead to form a uniform film of light absorbing HLH: Pb + MAI3 = MAPbI3 (Turkevych et al., 2019). The driving force of this “chemical” crystallization process is that this is not an equilibrium system and has a huge difference in chemical potentials of components between the contacting phases. The chemical transformation of lead into a chemically compatible phase PbI2 results in its dissolution in RPM followed by crystallization of the HLH perovskite from supersaturated RPM since it is dictated by the driving force of the first stage of the lead interaction with RPM. Actually, this much resembles the most effective protocol of HTSC melt processing (OCMG, Tretyakov and Goodilin, 2000), starting from the REE2O3 phase incompatible with an admixture of Cu(I) barium cuprite which is transformed upon heating in liquid converting REE2O3 into the REE2BaCuO5 compatible phase followed by its dissolution in the cuprate melt under cooling to crystallize finally the REEBa2Cu3O7 HTSC (Tretyakov et al., 2004). Thus, the philosophy of preparation of new functional materials is common enough for different perovskites under the question with deviations naturally connected with chemical features predicted by the element's position in PTE.
Conclusions and Perspectives
The families of promising perovskite materials discussed in this review have achieved quite different stages of implementation and practical applications. Despite the earliest discovery of CMR manganites, their applications are postponed for years because of their too narrow possible use in spintronics which is still not competitive with current trends in modern electronics. Expectations to use the CMR materials as wide-spread and fast magnetic sensors are moderate since SQUID devices outperform the manganites to a large extent. The remaining area of manganite applications is still connected with multiferroic systems and possible devices based on these multifunctional materials. HTSCs have achieved the heights of first industrial applications in superconducting electronics like SQUID and magnetic medical tomographs or industrial fault current limiters based on the second generation HTSC tapes, all after about 30 years beyond the HTSC discovery. There are a few already successful pilot projects of HTSC applications in transport systems like Maglev trains, propulsion ships and even small air craft jet systems. At the same time, a huge area of future applications of HTSCs is thinkable in megascience magnetic systems like synchrotrons, adroid colliders and thermonuclear plasma traps. However, the cost and operation stability issues still do not allow for the replacement of low temperature intermetallic superconductors. The frontier HLH solar cells and efficient light emitting devices, tandem power generating systems are the current challenges for science and engineering of hybrid perovskites with important remaining problems of stability and scaling up approaches. At the same time, their simpler architecture, chemistry and morphological requirements allow us to believe that these perovskites will come into commercial use much sooner compared to the other two families of perovskites, at least it is a prediction to optimistically believe.
Author Contributions
EG prepared and wrote the manuscript draft. All authors added textual and reference information and approved the manuscript submission after mutual discussion.
Funding
This work was supported by Russian Science Foundation (project No. 19-73-30022).
Conflict of Interest
The authors declare that the research was conducted in the absence of any commercial or financial relationships that could be construed as a potential conflict of interest.
Acknowledgments
The authors thank S. Lee, E. Pomerantseva, V. Ivanov, and D. Itkis for fruitful discussions.
Footnote
1. ^Pearson, 1963; species with larger radii, corresponding smaller charge states and higher polarizability of chemical elements reduce the lattice energy of solid phases and their dependent “thermal stability.”
References
Abdulaeva, I. A., Birin, K. P., Bessmertnykh-Lemeune, A., Tsivadze, A., and Gorbunova, Y. G. (2020). Heterocycle-appended porphyrins: synthesis and challenges. Coord. Chem. Rev. 407:213108. doi: 10.1016/j.ccr.2019.213108
Ahn, K. H., Lookman, T., and Bishop, A. R. (2004). Strain-induced metal-insulator phase coexistence in perovskite manganites. Nature 428, 401–404. doi: 10.1038/nature02364
Attfield, J. P. (2011). Chemistry and high temperature superconductivity. J. Mater. Chem. 21, 4756–4764. doi: 10.1039/c0jm03274c
Babushkina, N. A., Belova, L. M., Gorbrnko, O. Y., Kaul, A. R., Bosak, A. A., Ozhogin, V. I., et al. (1998). Metal–insulator transition induced by oxygen isotope exchange in the magnetoresistive perovskite manganites. Nature 391, 159–161. doi: 10.1038/34380
Bednorz, J. G., and Müller, K. A. (1986). Possible highT c superconductivity in the Ba–La–Cu–O system. Zeitschrift fur Phys. B Condens. Matter. 64, 189–193. doi: 10.1007/BF01303701
Bottari, G., de la Torre, G., Guldi, D. M., and Torres, T. (2010). Covalent and noncovalent phthalocyanine–carbon nanostructure systems: synthesis, photoinduced electron transfer, and application to molecular photovoltaics. Chem. Rev. 110, 6768–6816. doi: 10.1021/cr900254z
Boyd, C. C., Cheacharoen, R., Leijtens, T., and McGehee, M. D. (2019). Understanding degradation mechanisms and improving stability of perovskite photovoltaics. Chem. Rev. 119, 3418–3451. doi: 10.1021/acs.chemrev.8b00336
Chen, H., Ye, F., Tang, W., He, J., Yin, M., Wang, Y., et al. (2017). A solvent- and vacuum-free route to large-area perovskite films for efficient solar modules. Nature 550, 92–95. doi: 10.1038/nature23877
Colclough, M. S., Gough, C. E., Keene, M., Muirhead, C. M., Thomas, N., Abell, J. S., et al. (1987). Radio–frequency SQUID operation using a ceramic high–temperature superconductor. Nature 328, 47–48. doi: 10.1038/328047a0
De Roo, J., Ibanez, M., Geiregat, P., Nedelcu, G., Walravens, W., Maes, J., et al. (2016). Highly dynamic ligand binding and light absorption coefficient of cesium lead bromide perovskite nanocrystals. ACS Nano 10, 2071–2081. doi: 10.1021/acsnano.5b06295
Dong, F., Huang, Z., Hao, L., Xu, X., Jin, Z., and Shao, N. (2019). An on–board 2G HTSC magnets system with cooling–power–free and persistent–current operation for ultrahigh speed superconducting maglevs. Sci. Rep. 9:11844. doi: 10.1038/s41598-019-48136-x
Du, Y., Wang, X., Chen, D., Yu, Y., Hao, W., Cheng, Z., et al. (2013). Manipulation of domain wall mobility by oxygen vacancy ordering in multiferroic YMnO3. Phys. Chem. Chem. Phys. 15, 20010–20015. doi: 10.1039/c3cp52892h
Du, Y., Wang, X. L., Chen, D. P., Dou, S. X., Cheng, Z. X., Higgins, M., et al. (2011). Domain wall conductivity in oxygen deficient multiferroic YMNO3 single crystals. Appl. Phys. Lett. 99:252107. doi: 10.1063/1.3671393
Eperon, G., Hörantner, M., and Snaith, H. (2017). Metal halide perovskite tandem and multiple-junction photovoltaics. Nat. Rev. Chem. 1:0095. doi: 10.1038/s41570-017-0095
Fateev, S. A., Petrov, A. A., Khrustalev, V. N., Dorovatovskii, P. V., Zubavichus, Y. V., Goodilin, E. A., et al. (2018). Solution processing of methylammonium lead iodide perovskite from γ-butyrolactone: crystallization mediated by solvation equilibrium. Chem. Mater. 30, 5237–5244. doi: 10.1021/acs.chemmater.8b01906
Fert, A., Grünberg, P., Barthélémy, A., Petroff, F., and Zinn, W. (1995). Layered magnetic structures: interlayer exchange coupling and giant magnetoresistance. J. Magn. Magn. Mater. 140–144, 1–8. doi: 10.1016/0304-8853(94)00880-9
Frost, J. M., Butler, K. T., Brivio, F., Hendon, C. H., van Schilfgaarde, M., and Walsh, A. (2014). Atomistic origins of high-performance in hybrid halide perovskite solar cells. Nano Lett. 14, 2584–2590. doi: 10.1021/nl500390f
Fu, Y., Zhu, H., Chen, J., et al. (2019). Metal halide perovskite nanostructures for optoelectronic applications and the study of physical properties. Nat. Rev. Mater. 4, 169–188. doi: 10.1038/s41578-019-0080-9
Gao, P., Bin Mohd Yusoff, A. R., and Nazeeruddin, M. K. (2018). Dimensionality engineering of hybrid halide perovskite light absorbers. Nat. Commun. 9:5028. doi: 10.1038/s41467-018-07382-9
Gao, X. S., Rodriguez, B. J., Liu, L. F., Birajdar, B., Pantel, D., Ziese, M., et al. (2010). Microstructure and properties of well-ordered multiferroic Pb(Zr, Ti)O3/CoFe2O4 nanocomposites. ACS Nano 4, 1099–1107. doi: 10.1021/nn9012934
Goodilin, E., Kambara, M., Umeda, T., and Shiohara, Y. (1997). Solubility of neodymium in copper-rich oxide melts in air and growth of Nd1−xBa2−xCu3Oz solid solution single crystals. Phys. C 289, 37–50. doi: 10.1016/S0921-4534(97)01596-7
Goodilin, E., Oka, A., Wen, J. G., Shiohara, Y., Kambara, M., and Umeda, T. (1998). Twins and related morphology of as-grown neodymium-rich Nd1+xBa2−xCu3Oz crystals. Phys. C 299, 279–300. doi: 10.1016/S0921-4534(97)01808-X
Goodilin, E. A., Reddy, E. A., Noudem, J. G., Tarka, M., and Schmitz, G. J. (2002). Texture formation in melt-solidified yba2cu3oz thick films by artificial surface reliefs. J. Cryst. Growth 241, 512–534. doi: 10.1016/S0022-0248(02)01320-9
Grätzel, M. (2014). The light and shade of perovskite solar cells. Nat. Mater. 13, 838–842. doi: 10.1038/nmat4065
Hao, M., Bai, Y., Zeiske, S., Ren, L., Liu, J., Yuan, Y., et al. (2020). Ligand-assisted cation–exchange engineering for high–efficiency colloidal Cs1−xFAxPbI3 quantum dot solar cells with reduced phase segregation. Nat. Energy 5, 79–88. doi: 10.1038/s41560-019-0535-7
Haugan, T., Barnes, P. N., Wheeler, R., Meisenkothen, F., and Sumption, M. (2004). Addition of nanoparticle dispersions to enhance flux pinning of the YBa2Cu3O7−x superconductor. Nature 430, 867–870. doi: 10.1038/nature02792
Hwang, J., Feng, Z., Charles, N., Wang, X. R., Lee, D., Stoerzinger, K. A., et al. (2019). Tuning perovskite oxides by strain: electronic structure, properties, and functions in (electro)catalysis and ferroelectricit. Mater. Today 31, 100–118. doi: 10.1016/j.mattod.2019.03.014
Hwang, J., Rao, R. R., Giordano, L., Katayama, Y., Yu, Y., and Shao-Horn, Y. (2017). Perovskites in catalysis and electrocatalysis. Science 358, 751–756. doi: 10.1126/science.aam7092
Jiang, J., Li, Y. Y., Liu, J. P., Huang, X. T., Yuan, C. Z., and Lou, X. W. (2012). Recent advances in metal oxide-based electrode architecture design for electrochemical energy storage. Adv. Mat. 24, 5166–5180. doi: 10.1002/adma.201202146
Kojima, A., Teshima, K., Shirai, Y., and Miyasaka, T. (2009). Organometal halide perovskites as visible-light sensitizers for photovoltaic cells. J. Am. Chem. Soc. 131, 6050–6051. doi: 10.1021/ja809598r
Lee, S., Petrykin, V., Molodyk, A., Samoilenkov, S., Kaul, A., Vavilov, A., et al. (2014). Development and production of second generation high Tc superconducting tapes at superOx and first tests of model cables. Supercond. Sci. Technol. 27:044022. doi: 10.1088/0953-2048/27/4/044022
Leijtens, T., Bush, K. A., Prasanna, R., and McGehee, M. D. (2018). Opportunities and challenges for tandem solar cells using metal halide perovskite semiconductors. Nat. Energy 3, 828–838. doi: 10.1038/s41560-018-0190-4
Li, L.-L., and Diau, E. W.-G. (2013). Porphyrin-sensitized solar cells. Chem. Soc. Rev. 42, 291–304. doi: 10.1039/C2CS35257E
Li, W., Wang, Z., Deschler, F., Gao, S., Friend, R. H., and Cheetham, A. K. (2017). Chemically diverse and multifunctional hybrid organic–inorganic perovskites. Nat. Rev. Mater. 2:16099. doi: 10.1038/natrevmats.2016.99
Li, Z., Klein, T. R., Kim, D. H., Yang, M., Berry, J. J., van Hest, M. F. A. M., et al. (2018). Scalable fabrication of perovskite solar cells. Nat. Rev. Mater. 3:18017. doi: 10.1038/natrevmats.2018.17
Martynov, A. G., Safonova, E. A., Tsivadze, A. Y., and Gorbunova, Y. G. (2019). Functional molecular switches involving tetrapyrrolic macrocycles. Coord. Chem. Rev. 387, 325–347. doi: 10.1016/j.ccr.2019.02.004
Mathew, S., Yella, A., Gao, P., Humphry-Baker, R., Curchod, B. F. E., Ashari-Astani, N., et al. (2014). Dye-sensitized solar cells with 13% efficiency achieved through the molecular engineering of porphyrin sensitizers. Nat. Chem. 6, 242–247. doi: 10.1038/nchem.1861
Meggiolaro, D., Motti, S. G., Mosconi, E., Barker, A. J., Ball, J., Andrea Riccardo Perini, C., et al. (2018). Iodine chemistry determines the defect tolerance of lead-halide perovskites. Energy Environ. Sci. 11, 702–713. doi: 10.1039/C8EE00124C
Murali, B., Kolli, H. K., Yin, J., Ketavath, R., Bakr, O. M., and Mohammed, O. F. (2020). Single crystals: the next big wave of perovskite optoelectronics. ACS Mater. Lett. 2, 184–214. doi: 10.1021/acsmaterialslett.9b00290
Nayak, P. K., Mahesh, S., Snaith, H. J., and Cahen, D. (2019). Photovoltaic solar cell technologies: analysing the state of the art. Nat. Rev. Mater. 4, 269–285. doi: 10.1038/s41578-019-0097-0
Osterloh, F. E. (2013). Inorganic nanostructures for photoelectrochemical and photocatalytic water splitting. Chem. Soc. Rev. 42, 2294–2320. doi: 10.1039/C2CS35266D
Ovcharov, A. V., Degtyarenko, P. N., Chepikov, V. N., Vasiliev, A. L., Gavrilkin, S. Y., Karateev, I. A., et al. (2019). Microstructure and superconducting properties of high-rate PLD–derived GdBa2Cu3O7−δ coated conductors with BaSnO3 and BaZrO3 pinning centers. Sci. Rep. 9:15235. doi: 10.1038/s41598-019-51348-w
Park, N., Grätzel, M., Miyasaka, T., Zhu, K., and Emery, K. (2016). Towards stable and commercially available perovskite solar cells. Nat. Energy 1:16152. doi: 10.1038/nenergy.2016.152
Park, N.-G. (2016). Methodologies for high efficiency perovskite solar cells. Nano Converg. 3:15. doi: 10.1186/s40580-016-0074-x
Pearson, R. G. (1963). Hard and soft acids and bases. J. Am. Chem. Soc. 85, 3533–3539. doi: 10.1021/ja00905a001
Petrov, A. A., Belich, N. A., Grishko, A. Y., Stepanov, N. M., Dorofeev, S. G., Maksimov, E. G., et al. (2017a). A new formation strategy of hybrid perovskites via room temperature reactive polyiodide melts. Mater. Horiz. 4, 625–632. doi: 10.1039/C7MH00201G
Petrov, A. A., Fateev, S. A., Zubavichus, Y. V., Dorovatovskii, P. V., Victor, N., Zvereva, I. A., et al. (2019). Methylammonium polyiodides: remarkable phase diversity of the simplest and low-melting alkylammonium polyiodide system. J. Phys. Chem. Lett. 10, 5776–5780. doi: 10.1021/acs.jpclett.9b02360
Petrov, A. A., Pellet, N., Seo, J.-Y., Belich, N. A., Kovalev, D. Y., Shevelkov, A. V., et al. (2017b). New insight into the formation of hybrid perovskite nanowires via structure directing adducts. Chem. Mater. 29, 587–594. doi: 10.1021/acs.chemmater.6b03965
Petrov, A. A., Sokolova, I. P., Belich, N. A., Peters, G. S., Dorovatovskii, P. V., Zubavichus, Y. V., et al. (2017c). Crystal structure of DMF-intermediate phases uncovers the link between CH3NH3PbI3 morphology and precursor stoichiometry. J. Phys. Chem. C 121, 20739–20743. doi: 10.1021/acs.jpcc.7b08468
Petrykin, V. V., Goodilin, E. A., Hester, J., Trofimenko, E. A., Kakihana, M., Oleynikov, N. N., et al. (2000). Structural disorder and superconductivity suppression in NdBa2Cu3Oz (z similar to 7). Phys. C 340, 16–32. doi: 10.1016/S0921-4534(00)00368-3
Pomerantseva, E. A., Itkis, D. M., Goodilin, E. A., Noudem, J. G., Lobanov, M. V., Greenblatt, M., et al. (2004). Homogeneity field and magnetoresistance of the Ca(Mn, Cu)7O12 solid solution prepared in oxygen. J. Mater. Chem. 14, 1150–1156. doi: 10.1039/b313570e
Shiohara, Y., and Goodilin, E. A. (2000). “Single crystal growth for science and technology,” in Handbook on the Physics and Chemistry of Rare-Earths (Special Volumes on High Temperature Rare Earth Superconducrors), Vol. 30, eds K. A. Gschneidner, Jr., L. Etring, and M. B. Maple (Amsterdam: Elsevier Science Publishers), 154. doi: 10.1016/S0168-1273(00)30006-X
Shlenskaya, N. N., Belich, N. A., Gratzel, M., Goodilin, E. A., and Tarasov, A. B. (2018). Light-induced reactivity of gold and hybrid perovskite as a new possible degradation mechanism in perovskite solar cells. J. Mater. Chem. A 6, 1780–1786. doi: 10.1039/C7TA10217H
Smith, M. D., Crace, E. J., Jaffe, A., and Karunadasa, H. I. (2018). The diversity of layered halide perovskites. Annu. Rev. Mater. Res. 48, 111–136. doi: 10.1146/annurev-matsci-070317-124406
Snaith, H. J., and Hacke, P. (2018). Enabling reliability assessments of pre-commercial perovskite photovoltaics with lessons learned from industrial standards. Nat. Energy 3, 459–465. doi: 10.1038/s41560-018-0174-4
Song, J., Li, J., Li, X., Xu, L., Dong, Y., and Zeng, H. (2015). Quantum dot light-emitting diodes based on inorganic perovskite cesium lead halides (CsPbX3). Adv. Mater. 27, 7162–7167. doi: 10.1002/adma.201502567
Travis, W., Glover, E. N. K., Bronstein, H., Scanlon, D. O., and Palgrave, R. G. (2016). On the application of the tolerance factor to inorganic and hybrid halide perovskites: a revised system. Chem. Sci. 7, 4548–4556. doi: 10.1039/C5SC04845A
Tretyakov, Y. D., and Goodilin, E. A. (2000). Chemical principles of the metall-oxide superconductors preparation. Russian Chem. Rev. 69, 3–40. doi: 10.1070/RC2000v069n01ABEH000526
Tretyakov, Y. D., Goodilin, E. A., Peryshkov, D. V., and Itkis, D. M. (2004). Structural and microstructural features of functional materials based on cuprates and manganites. Russian Chem. Rev. 73, 954–973. doi: 10.1070/RC2004v073n09ABEH000920
Turkevych, I., Kazaoui, S., Belich, N. A., Grishko, A. Y., Fateev, S. A., Petrov, A. A., et al. (2019). Strategic advantages of reactive polyiodide melts for scalable perovskite photovoltaics. Nat. Nanotechnol. 14, 57–63. doi: 10.1038/s41565-018-0304-y
Van Santen, J. H., and Jonker, G. H. (1950). Electrical conductivity of ferromagnetic compounds of manganese with perovskite structure. Physica 16, 599–600. doi: 10.1016/0031-8914(50)90104-2
Volger, J. (1954). Further experimental investigations on some ferromagnetic oxidic compounds of manganese with perovskite structure. Physica 20, 49–66. doi: 10.1016/S0031-8914(54)80015-2
von Helmolt, R., Wecker, J., Holzapfel, B., Schultz, L., and Samwer, K. (1993). Giant negative magnetoresistance in perovskitelike La2/3Ba1/3MnOx ferromagnetic films. Phys. Rev. Lett. 71, 2331–2333. doi: 10.1103/PhysRevLett.71.2331
Wang, L., Xu, K., Cui, W., Lv, D., Wang, L., Ren, L., et al. (2019). Monolayer epitaxial heterostructures for selective visible-light-driven photocatalytic NO oxidatiion. Adv. Funct. Mater. 29:1808084. doi: 10.1002/adfm.201808084
Wei, H., and Huang, J. (2019). Halide lead perovskites for ionizing radiation detection. Nat. Commun. 10:1066. doi: 10.1038/s41467-019-08981-w
Weidman, M. C., Seitz, M., Stranks, S. D., and Tisdale, W. A. (2016). Highly tunable colloidal perovskite nanoplatelets through variable cation, metal, and halide composition. ACS Nano 10, 7830–7839. doi: 10.1021/acsnano.6b03496
Yang, W., Shi, Q., Miao, T., Li, Q., Cai, P., Liu, H., et al. (2019). Achieving large and nonvolatile tunable magnetoresistance in organic spin valves using electronic phase separated manganites. Nat. Commun. 10:3877. doi: 10.1038/s41467-019-11827-0
Yi, C., Luo, J., Meloni, S., Boziki, A., Ashari-Astani, N., Grätzel, C., et al. (2016). Entropic stabilization of mixed A-cation ABX 3 metal halide perovskites for high performance perovskite solar cells. Energy Environ. Sci. 9, 656–662. doi: 10.1039/C5EE03255E
Zhang, W., Eperon, G. E., and Snaith, H. J. (2016). Metal halide perovskites for energy applications. Nat. Energy 1:16048. doi: 10.1038/nenergy.2016.48
Zhang, W., Goodilin, E. A., and Hellstrom, E. E. (1996). Composition studies for Ag-sheathed Bi2Sr2CaCu2O8 conductors processed in 100% O2. Supercond. Sci. Technol. 9, 211–217. doi: 10.1088/0953-2048/9/3/014
Zhumekenov, A. A., Saidaminov, M. I., and Bakr, O. M. (2018). “Perovskite single crystals: synthesis, properties and devices,” in World Scientific Handbook of Organic Optoelectronic Devices, eds J. Huang and Y. Yuan (Singapore: World Scientific Publishing Co Pte Ltd.), 241–283. doi: 10.1142/9789813239494_0008
Keywords: perovskites, superconductivity, magnetoresistance, photovoltaics, copper, manganese, perovskite solar cells, lead halides
Citation: Belich N, Udalova N, Semenova A, Petrov A, Fateev S, Tarasov A and Goodilin E (2020) Perovskite Puzzle for Revolutionary Functional Materials. Front. Chem. 8:550625. doi: 10.3389/fchem.2020.550625
Received: 09 April 2020; Accepted: 28 August 2020;
Published: 02 November 2020.
Edited by:
Zhong Jin, Nanjing University, ChinaReviewed by:
Yanlin Song, Institute of Chemistry (CAS), ChinaYulia Gorbunova, NS Kurnakova Institute of General and Inorganic Chemistry (RAS), Russia
Copyright © 2020 Belich, Udalova, Semenova, Petrov, Fateev, Tarasov and Goodilin. This is an open-access article distributed under the terms of the Creative Commons Attribution License (CC BY). The use, distribution or reproduction in other forums is permitted, provided the original author(s) and the copyright owner(s) are credited and that the original publication in this journal is cited, in accordance with accepted academic practice. No use, distribution or reproduction is permitted which does not comply with these terms.
*Correspondence: Eugene Goodilin, Z29vZGlsaW5AaW5vcmcuY2hlbS5tc3UucnU=