- Department of Product Design, Mechatronics and Environment, Transilvania University of Brasov, Brasov, Romania
Applications of TiO2 nanomaterials in photocatalysis, batteries, supercapacitors and solar cells, have seen widespread development in recent decades. Nowadays, black TiO2 have won attention due to enhancing the solar light absorption by the formation of oxygen vacancies and Ti3+ defects, to promote the separation of photo-generated charge carriers leading to the improvement of the photocatalytic performance in H2 production and pollutants degradation. The enhanced photocatalytic activity of black TiO2 is also due to a lattice disorder on the surface and the presence of oxygen vacancies, Ti3+ ions, Ti-OH and Ti-H groups. Enhancing the optical absorption characteristics of TiO2 and change of energy level and band-gap of materials have been successfully demonstrated to improve their photocatalytic activities, especially for black TiO2 nanoparticles, which promote visible light absorption. The current review focuses on the investigation of the chemical reduction synthetic route for black TiO2 nanomaterials, and their proposed association with green applications such as photodegradation of organic pollutants and photocatalytic water splitting. The synthesis methods of black TiO2 involves the changes from Ti4+ to Ti3+ state, into different strategies: (1) The use of highly active hydrogen species such as H2, H2/Ar or H2/N2 gases, and metal hydrides (NaBH4, CaH2), (2) the reduction by active metals such as aluminum, magnesium and zinc, and (3) organic molecules such as imidazole and ascorbic acid.
Theoretical Consideration of Black TiO2
Chen et al. first reported black TiO2 with a narrowed band-gap of 1.5 eV to expand the full spectrum sunlight absorption and promote an increase in the photocatalytic activity, by introducing surface disorders in the TiO2 (Chen et al., 2011). Hu et al. observed in 2012 a remarkable enhancement in the visible-light absorption and the photocatalysis of TiO2 after hydrogen treatment, attributed to surface disorder and the formation of oxygen vacancies (Hu, 2012; Wang and Chou, 2016; Zhu et al., 2016).
In the past decade, a considerable effort has been committed to preparing black TiO2 by introducing Ti3+ defects and oxygen vacancies into the titanium oxide lattice (Di Valentin et al., 2009; Su et al., 2014; Li et al., 2015; Tian et al., 2015; Xin et al., 2015). Oxygen vacancy and Ti3+ defects are more detectable in black TiO2 compared with white TiO2. Oxygen vacancies have been detected by a few techniques (Zhang and Park, 2017): electron paramagnetic resonance (EPR), electron spin resonance (ESR), and Raman spectroscopy. Ti3+ defects are not proved in white TiO2, but they are detected in black TiO2 based on X-ray photoelectron spectroscopy (XPS), by EPR (Jedsukontorn et al., 2017) or ESR spectroscopy (Tian et al., 2015). The yellow TiO2 synthesis at a low temperature had more oxygen vacancies and Ti3+ defects compared with white TiO2, which decreases the band-gap from 3.1 to 2.9 eV (Bi et al., 2020).
The formation defects in titanium oxide are given below (Jayashree and Ashokkumar, 2018):
Oxygen vacancy (Kröger-Vink notation ) formation at a low oxygen pressure
Titanium interstitials Ti3+ (Kröger-Vink notation ):
Cui et al. (2014) described the generation of the Ti3+ and oxygen vacancies, by the equation of the defect (equation 3). They observed that the number of oxygen vacancies is half of the Ti3+ sites (equation 3). The absorption increases with the density of Ti3+ or O vacancies, as the density of Ti3+ increases with the Al reduction in temperature.
The colorful TiO2 with better absorption properties and improved photocatalytic activities compared with white TiO2 have been designed through (i) metal doping in which metal replaces Ti4+ ions in the TiO2 lattice (Chen et al., 2015), (ii) non-metal doping to replace O2− ions in the TiO2 lattice (Hamilton et al., 2014), (iii) to replace partial Ti4+ and O2 ions in the TiO2 lattice. To replace Ti4+ in TiO2 with any cation is more accessible than to substitute O2− with anions (such as nitrogen, carbon, sulfur) due to the difference in the charge state and ionic radii (Lee et al., 2014).
The structural modifications in black titanium oxide, involving Ti3+ centers and oxygen vacancies, conduct significant changes in crystallinity, and optoelectronic as well as the surface properties, and the most marked effect is the color changing. Increasing the optical absorption properties and diminishing electron-hole recombination of TiO2 are expected to be meaningful for excellent photoactivity.
The optical band gaps of white anatase and rutile TiO2 are reported as 3.2 and 3.0 eV, respectively (Haider et al., 2019), that means TiO2 can adsorb only the UV part of the solar spectrum. A significant reduction in the TiO2 band-gap around 1.23 eV and optical absorption near 1,000 nm in the near-infrared region (Ullattil et al., 2018), has been reported by hydrogenation of anatase nanocrystals under pressure resulting in the black TiO2 materials (Chen et al., 2011; Liu et al., 2013).
The color change of titanium oxide from gray, blue, brown or black color, reflects in turn the optical properties and structural changes (Yan et al., 2017). By exposure to visible light, a heterojunction type I (Isac et al., 2019) is formed between white TiO2 and colored TiO2, the band energy levels of colored TiO2 are included in that of the white TiO2, and both heterojunction semiconductors could be excited to produce electrons and holes (Figure 1).
The colored TiO2 shows a light absorption around 2eV, by introducing oxygen vacancies () and Ti3+ formation into TiO2 lattice (Naldoni et al., 2019) or introducing disordered layers in the surface of crystalline TiO2 (Song et al., 2017) enhanced solar light adsorption and served to prove their photocatalytic performance. The oxygen vacancy can significantly affect the electric and optical properties of the materials, by forming a donor level below the conduction band, located at 1.8eV below conduction band of titania as shown in Figure 1. The Ti3+ defect is responsible for changes in the electronic conductivity and optical properties. The Ti3+ and defects can be created by the reduction of TiO2, either electrochemically, or through gas annealing and exposure in a vacuum (Lee et al., 2014).
Hu emphasize the importance of the crystalline phase of titanium oxide in the synthesis of black TiO2; the rutile phase is theoretically easier to obtain than anatase crystalline phase because the formation energy of an oxygen vacancy in the rutile surface (110) is lower (5.5eV) than in the anatase surface (001) (7.54 eV) (Hu, 2012). The most common phase of the black TiO2 is rutile or anatase; the rutile phase is formed at a temperature below 500°C. The oxygen deficiency and amorphous surface of TiO2−x were also reported by Tan et al. (2014). The photocatalytic behavior of TiO2 was found to depend on the crystalline phase; the white TiO2 anatase phase has been shown to have higher photocatalytic efficiency than rutile TiO2. Contrary to the previous statement, black rutile TiO2 has been reported to have the best photocatalytic performance.
The synthesis methods of black TiO2 are significantly affected by the structural, morphological and optical properties; involving the changes from Ti4+ to Ti3+ state, into different strategies, (i) the use of highly active hydrogen species such as H2, H2/Ar or H2/N2 gases, and metal hydrides (NaBH4, CaH2), (ii) the reduction by active metals such aluminum, magnesium and zinc, (iii) organic molecules such as imidazole and ascorbic acid, have been confirmed to be capable of reducing white TiO2 to black titania.
Synthesis Approach of Black TiO2 by Chemical Reduction
The synthesis methods explored through hydrogenation, plasma, chemical reduction, electrochemical reduction, laser ablation in liquid, and oxidation approaches were available in the literature over the last decade for black TiO2 photocatalytic materials (Rajaraman et al., 2020).
The synthesis route influences the physicochemical properties and photocatalytic performance of black TiO2. A significant number of studies highlight the formation of black TiO2 by hydrogen thermal treatment when the samples had surface and bulk defects comparing with plasma treatment under Ar (95%)/H2 (5%) atmosphere where the bulk defects were revealed (Wang and Chou, 2016). The color of the samples turned brown at 400°C, while the samples turned black at 500°C. The white TiO2 Degussa powder was unchanged under hydrogenation, emphasized the role of precursors and synthesis route (Leshuk et al., 2013).
The reduction strategy can be generally explained in Equation 4, where Red represents the reductant:
The noble gas atmosphere has been considered as reductant due to defective TiO2−x formation in argon, nitrogen atmosphere, and the disordered layer forms only if crystallization is performed in an oxygen-free environment (Tian et al., 2015).
The first synthesis of black titanium oxide consists of anatase nanoparticles through treating white TiO2 nanoparticles (precursors, titanium tetra-isopropoxide, ethanol, hydrochloric acid, deionised water, and Pluronic F127 as an organic template) under a 20.0-bar H2 atmosphere at about 200°C for 5 days (Chen et al., 2011).
NaBH4 Reduction
By reducing hydrides, black titanium oxide can be obtained through three approaches: (i) physical mixing of white TiO2 and hydride, followed by annealing in an inert atmosphere (Ar, N2) at temperature of 300…400°C, (ii) hydrothermal synthesis (Ren et al., 2015) and (iii) sol-gel process (Fang et al., 2014).
The sodium borohydride (NaBH4) is a commonly used reducing reagent (Table 1), due to its ability to reduce Ti4+ to Ti3+, as in Equations (6) and (7), and to produce in situ active H2 at room temperature, that reduces the white TiO2 into black TiO2 (Equation 8). During the NaBH4 reduction process, boron oxide species are produced due to their insolubility in the ethanol, and it can be easily washed out by HCl solution to remove the surface impurities and expose the color centers on the surface of the catalyst, significantly increasing the visible light absorption. The degradation efficiency increases 9 times after washing with HCl solution (Fang et al., 2014).
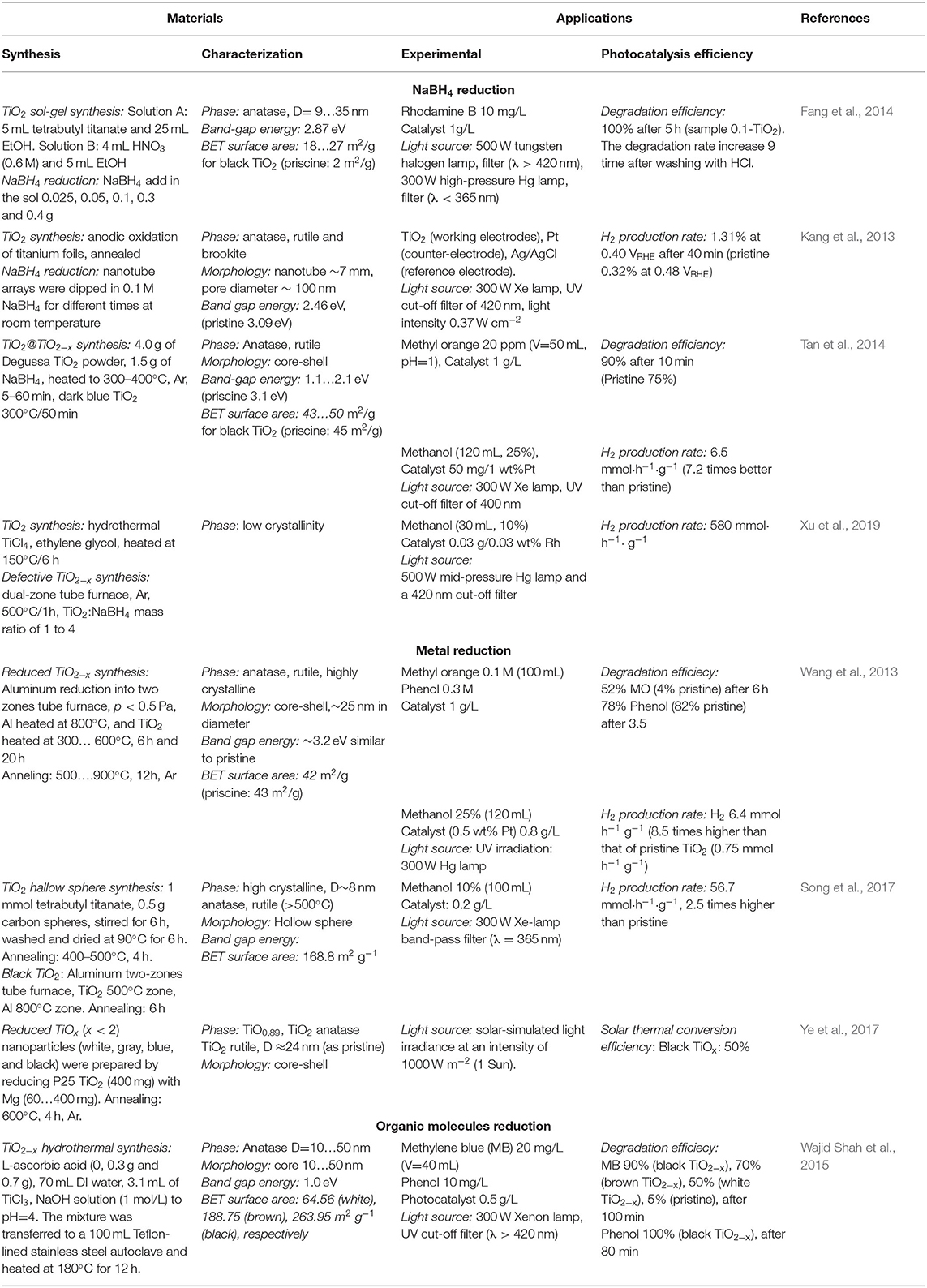
Table 1. The chemical reduction synthesis methods, properties and photocatalytic applications of representative black TiO2 materials.
The rate of the generation of H2 is higher in acidic conditions at experiments performed at 25°C with 20 g water per gram of sodium borohydride, and a wt. ratio accelerators/NaBH4, around 1. The representative acidic materials that act as accelerators are tartaric acid, citric acid, succinic acid, oxalic acid (85…98% hydrogen liberated after 3 min), ammonium carbonate, maleic acid, aluminum sulfate, sodium diacid phosphate (90….80% of H2 after 10 min), maleic anhydride, ammonium chloride, benzoic acid (80….65% of H2 after 10 min). The catalytic effect of metals salt (cobalt, aluminum) was also demonstrated (Schlesinger et al., 1953).
The treatment of the TiO2 nanotube in NaBH4 for a short time (20–40 min) reduced the surface of TiO2 into Ti3+, and introduced an oxygen vacancy that creates localized states, producing a narrower band-gap of 2.46 eV, which extends its optical absorption to the visible region comparing with 3.09 eV for pristine TiO2 nanotubes (Table 1) (Kang et al., 2013). The films show good stability and excellent reproducibility of the samples.
Tan et al. report a solid-state chemical reduction of TiO2 at mild temperatures (300–350°C), for different times up to 1 h, an approach for large-scale production for visible light photocatalysis and solar-driven H2 production (Table 1). The preparation of black TiO2 followed the procedure: 4 g of TiO2 Degussa P25 powder was mixed at room temperature with 1.5 g of NaBH4 (98%) and heated in a tubular furnace under Ar atmosphere, up to 300°C and held for 5–120 min. When the temperature increase to 350°C, the black titanium oxide was obtained in 60 min. The colored powders from light blue to black were washed with deionised water and ethanol several times to remove unreacted NaBH4 and dried at 70°C (Tan et al., 2014).
Xu et al. obtained black TiO2 powders in a dual-zone quart tube furnace using titanium oxide synthesized of TiCl4 and ethylene glycol at 150°C for 6 h in a Teflon-lined stainless-steel autoclave with NaBH4 as reductant agent (Table 1). The reduction was carried out in an argon atmosphere, between 200 and 500°C for 1 h. The presence of Ti3+ and oxygen vacancy defects significantly increased the intensity of the band absorption in the visible spectrum range (Xu et al., 2019).
Metal Reduction
In recent studies, active metals such as magnesium, lithium, aluminum and zinc were used for the synthesis of black TiO2 with oxygen-deficient metal oxides (Zu et al., 2019).
Ou et al. developed a room-temperature lithium reduction strategy removing oxygen, and generating oxygen vacancies into the titanium dioxide nanoparticles lattice. Lithium metal with a high reductive capacity can reduce a significant number of metal oxides at room temperature (TiO2, ZnO, SnO2, CeO2). TiO2 Degussa P25 and lithium powders (0.5% wt%) were mixed with a dispersant (dimethyl carbonate), then washed with diluted hydrochloric acid to remove lithium oxide, centrifugate and washing. The dried powders appear in different colors ranging from blue to black, and shift with the increase in lithium content (Ou et al., 2018).
The aluminum reduction of titanium oxide produces black TiO2 in two ways: (i) reduction approach in an evacuated two-zone vacuum furnace, low temperature (300–600°C) for TiO2 and high temperature (800°C) for aluminum and (ii) thermal treatment of a mixture of TiO2 and aluminum powder (Table 1) (Wang et al., 2013). The black TiO2 nanotube arrays have been used as a photoanode of photoelectrochemical cells for water-splitting, which was about 5 times higher than that of pristine (Cui et al., 2014).
Song et al. Herein prepared the black TiO2 nanoparticles through subsequent Al reduction, with hollow nanosphere morphology, high crystallinity, small grain size (~8 nm), and high surface area (168.8 m2·g−1) for photocatalytic hydrogen generation (56.7 mmol h−1·g−1) 2.5 times higher than pristine TiO2 nanostructures. The aluminum reaction was performed for 6 h in an evacuated two-zone furnace, pristine TiO2 hollow nanospheres were placed in the low-temperature zone (400….600°C), and the aluminum powder was placed at 800°C (Table 1) (Song et al., 2017).
Sinhamahapatra et al. report in 2015 a magnesiothermic reduction under a 5% H2/Ar atmosphere followed by acid treatment to synthesize reduced black TiO2 nanoparticles with improved optical absorption in the visible and infrared region for enhanced photocatalytic hydrogen production in the methanol-water system in the presence of Pt as a co-catalyst (Sinhamahapatra et al., 2015).
Nanoparticles with different colors were synthesized by Ye et al. (2017) using Mg as a reductant (Table 1). Commercial P25 TiO2 nanoparticles were mixed with Mg powder into wt. ratio 20:3, 10:3, 5:3, and 1:1, before being purged with argon for 15 min and calcined at 600°C under an Ar atmosphere for 4 h. The TiOx (x <2) nanoparticles with different Ti/O ratios increased with the increasing addition of Mg in the reaction (Equation 9) and colors (turned gray, blue-gray, light black, and dark black, respectively). The nanoparticles are material for converting solar energy to the thermal energy for evaporation of water.
Organic Molecules Reductant
Seok et al. synthesis Ti3+ self-doped TiO2 using the sol-gel route: 5 g of TiOSO4, 250 ml distilled water, 1.5 g urea as a dispersant, NaOH was added (pH=7), and precursors annealed under an oxidative atmosphere at 350°C for 6 h in the presence of 2-methylimidazole and HCl when the Ti4+ was reduced to Ti3+ which resulted in lower internal resistance and improved electronic conductivity with application in Li-ion batteries as anode materials with a capacity retention of 88% at 50°C (Seok et al., 2016).
A facile hydrothermal approach, described in Table 1, has been developed by Wajid et al. to prepare defective TiO2−x high surface nanocore using ascorbic acid as a reductant, established theoxygen vacancy concentration and tunable band-gap by setting the amount of ascorbic acid (Wajid Shah et al., 2015).
The synthesis methods of black TiO2 changed the phase and crystallinity, morphology, band-gap and BET surface area, essential elements in photocatalysis as described in Table 1. The experimental conditions (pollutants and catalysts concentration, light irradiation and intensity) influence the pollutant degradation efficiency and H2 production rate by photocatalysis (Table 1).
The synthesis techniques to obtain black TiO2 and defective TiO2−x follow four strategies: introducing surface disorders, Ti3+ defects, oxygen vacancies, Ti-OH and Ti-H groups to narrowing the band-gap for photo-related applications (Liu et al., 2017; Yan et al., 2017).
Chemical reduction is associated with a change in the oxidation state of Ti4+ with the formation of Ti3+ species responsible for the electronic conductivity, essential for many applications of TiO2, especially photocatalysis (Di Valentin et al., 2009). The surface Ti3+ species are unstable and can be quickly oxidized by oxygen in air or water, developing a method to synthesize black TiO2 materials that with improved visible-light photocatalytic activity is a challenge (Zheng et al., 2013).
A Fundamental Process in Photocatalytic Activity of Black TiO2
In the past years, black titanium oxide has attracted attention in different fields, such as photocatalytic pollutants degradation (Chen et al., 2011; Li et al., 2019; Plodinec et al., 2019), photocatalytic hydrogen production through water splitting (Wang et al., 2017; Pan et al., 2019), photocatalytic CO2 reduction (Qingli et al., 2015; Zhao et al., 2016; Gao et al., 2020), solar–thermal material (Ye et al., 2017), supercapacitor (Zhi et al., 2016; Huang et al., 2018), photoanode in Dye-Sensitized Solar Cells (Ullattil et al., 2017), Lithium-ion batteries (Kim et al., 2017) (Yang et al., 2018), and medicine (Ni et al., 2017; Mazare et al., 2019).
The principle of the semiconductors photocatalysis consists of the following components: photon absorption, carriers separation, carrier diffusion simultaneously with carrier transport, catalytic efficiency and mass transfer of reactants and products (Takanabe, 2017).
Photon absorption: if the semiconductor has energy equal to or greater than Eg and consequent excitation of electrons (e−) to the CB leaving positively charged vacancies, holes (h+), in the VB (Figure 1)
Carriers separation: the heterojunction between nanoparticles can better band gap arrangement, to improve the separation of photo-generated charge carriers (Figure 1), which is advantageous of improving the photocatalytic performance. The oxygen vacancy defects and Ti3+ centers on the surface of TiO2 favor the separation of charge carriers (electrons and holes) and can trap the hole.
Carrier diffusion simultaneously with carrier transport. The photo-generated electrons can initiate the reduction processes, including O2 reduction to superoxides, H2 generation, and CO2 reduction to methane, methanol, or formaldehyde (Wen et al., 2015). The electron transfer is significant for the knowledge of the fundamental concepts of photocatalytic processes and to have an opinion about design and industrialization of the photocatalytic process (Mohamed and Bahnemann, 2012).
The transfer of electron/hole pairs to the interface initiates the redox reaction. The lifetime of the photo-generated charge carrier determines the efficiency of photocatalytic processes (Takanabe, 2017). Hence, increasing the efficiency of charge separation/transport in semiconductor nanoparticles is one of the major problems in photocatalysis to be addressed by the black TiO2.
The presence of oxygen vacancies in TiO2 can efficiently extend the visible light absorption range of titania because the localized oxygen vacancy states are located at 0.75 to 1.18 eV below the conduction band of TiO2 (Asahi et al., 2001) (Figure 1). The hydroxyl radicals (HO∙) can be formed when hydroxyl anions (HO−), and adsorbed water trap the holes, which are capable of degrading the organic pollutants in wastewater.
Summary and Outlook
The current review focuses on the investigation of the chemical reduction synthetic route for black TiO2 nanomaterials, and their applications related to the environmental application such as photodegradation of organic pollutants and photocatalytic water splitting.
Since 1972, when Fujishima and Honda (Fujishima and Honda, 1972) reported about the water-splitting process using a TiO2 electrode under UV irradiation, photocatalysis has attracted attention. The solar-driven applications of TiO2 have been limited due to its band-gap (around 3.2 eV). A remarkable step in solar-driven photocatalysis was presented in 2011 by Chen and co-authors when black TiO2 enhanced the photocatalytic activity of TiO2. In the last years, many studies have focused on the synthesis and explanation of different properties of black-TiO2 to improve the activity of the photocatalyst under visible irradiation. An important drawback is the synthesis requirements such as long annealing treatments (a few days), and the high pressure of hydrogen atmosphere, up to 20 bar.
The colored TiO2 can turn from white to yellow, blue, brown or black, due to the change in optical properties (modification of its band-gap), and defects in the surface layers that enhanced solar light adsorption and photocatalytic reactions. Among the colored forms, black TiO2 has been one of the most investigated because it can get excellent optical, chemical and electronic properties due to at least one of these characteristics: the presence of Ti3+ ions, oxygen vacancies undetectable in white TiO2 and usually present in black TiO2, structural disorder/defects in the surface, Ti-OH groups, Ti-H groups, and modifications of the valence band edge. The colored TiO2 has rich oxygen vacancies and Ti3+ defects, which conduct to better conductivity for electron transfer, increased visible absorption and higher conduction band potential. The oxygen vacancies and Ti3+ defects can act as traps for reducing the recombination of e−/h+ pairs and enhancing the photocatalytic activity.
The chemical reduction methods include the reduction of TiO2 with active hydrogen species such as H2, H2/Ar or H2/N2 gases, using high temperatures with active metals such aluminum, magnesium and zinc powders, or the reduction of TiO2 in solution with NaBH4 and organic molecules such as imidazole and ascorbic acid can effectively lead to the color change of TiO2 into black color. The color change of TiO2 depends on the synthesis conditions, such as pressure, temperature, time, and the reducing agent, featuring different structural (lattice changes or disordering), chemical (formation of Ti3+, oxygen vacancies, Ti-H, Ti-OH), physical properties (such as optical properties), and photocatalytic activities in both hydrogen generation and organic pollutant removal. The thermal treatment changes the color of the samples between yellow at 300–350°C, brown at 400°C and black above 450°C, the crystal structure has no major changes due to hydrogenation.
Black titanium oxide is a versatile photocatalyst with an extended absorption spectrum into the vis light range of the solar spectrum. From both a material and a chemical reaction perspective, this may provide new opportunities in efficiently utilizing the visible-light region of the spectrum to finally improve the efficiency of black TiO2 nanomaterials for practical photocatalytic applications.
Author Contributions
LA planned the content and wrote the manuscript. AE contributed to the photocatalysis chapter. All authors contributed to the article and approved the submitted version.
Funding
This work was supported by a grant of the Romanian National Authority for Scientific Research and Innovation, CCCDI-UEFISCDI, Project number 114/2019 ERANET-M.-TESTIMONIES, within PNCDI III.
Conflict of Interest
The authors declare that the research was conducted in the absence of any commercial or financial relationships that could be construed as a potential conflict of interest.
References
Asahi, R., Morikawa, T., Ohwaki, T., Aoki, K., and Taga, Y. (2001). Visible-light photocatalysis in nitrogen-doped titanium oxides. Science 293, 269–271. doi: 10.1126/science.1061051
Bi, X., Du, G., Sun, D., Zhang, M., Yu, Y., Su, Q., et al. (2020). Room-temperature synthesis of yellow TiO2 nanoparticles with enhanced photocatalytic properties. Appl. Surf. Sci. 511:145617. doi: 10.1016/j.apsusc.2020.145617
Chen, X., Liu, L., and Huang, F. (2015). Black titanium dioxide (TiO2) nanomaterials. Chem. Soc. Rev. 44, 1861–1885 doi: 10.1039/C4CS00330F
Chen, X., Liu, L., Yu, P. Y., and Mao, S. S. (2011). Increasing solar absorption for photocatalysis with black hydrogenated titanium dioxide nanocrystals. Science 331, 746–750. doi: 10.1126/science.1200448
Cui, H., Zhao, W., Yang, C., Yin, H., Lin, T., Shan, Y., et al. (2014). Black TiO2 nanotube arrays for high-efficiency photoelectrochemical water-splitting. J. Mater. Chem. A 2, 8612–8616. doi: 10.1039/C4TA00176A
Di Valentin, C., Pacchioni, G., and Selloni, A. (2009). Reduced and n-type doped TiO2: nature of Ti3+ species. J. Phy. Chem. C 113, 20543–20552. doi: 10.1021/jp9061797
Fang, W., Xing, M., and Zhang, J. (2014). A new approach to prepare Ti3+ self-doped TiO2 via NaBH4 reduction and hydrochloric acid treatment. Appl. Catal. B Environ. 160–161, 240–246. doi: 10.1016/j.apcatb.2014.05.031
Fujishima, A., and Honda, K. (1972). Electrochemical photolysis of water at a semiconductor electrode. Nature 238, 37–38. doi: 10.1038/238037a0
Gao, J., Shen, Q., Guan, R., Xue, J., Liu, X., Jia, H., et al. (2020). Oxygen vacancy self-doped black TiO2 nanotube arrays by aluminothermic reduction for photocatalytic CO2 reduction under visible light illumination. J. CO2 Util. 35, 205–215. doi: 10.1016/j.jcou.2019.09.016
Haider, A. J., Jameel, Z. N., and Al-Hussaini, I. H. M. (2019). Review on: titanium dioxide applications. Enrgy. Proced. 157, 17–29. doi: 10.1016/j.egypro.2018.11.159
Hamilton, J. W. J., Byrne, J. A., Dunlop, P. S. M., Dionysiou, D. D., Pelaez, M., O'Shea, K., et al. (2014). Evaluating the mechanism of visible light activity for N,F-TiO2 using photoelectrochemistry. J. Phys. Chem. C 118, 12206–12215. doi: 10.1021/jp4120964
Hu, Y. H. (2012). A highly efficient photocatalyst-hydrogenated black TiO2 for the photocatalytic splitting of water. Angew. Chem. Int. Ed. 51, 12410–12412. doi: 10.1002/anie.201206375
Huang, C. H., Meen, T. H., Ji, L. W., Chao, S. M., Wu, T. L., Tsai, J. K., et al. (2018). Effects of TiO2 nanoparticle doping in coconut-shell carbon on the properties of supercapacitor. Sens. Mater. 30, 645–653. doi: 10.18494/SAM.2018.1769
Isac, L., Cazan, C., Enesca, A., and Andronic, L. (2019). Copper sulfide based heterojunctions as photocatalysts for dyes photodegradation. Front. Chem. 7:694. doi: 10.3389/fchem.2019.00694
Jayashree, S., and Ashokkumar, M. (2018). Switchable intrinsic defect chemistry of titania for catalytic applications. Catalysts 8, 1–26. doi: 10.3390/catal8120601
Jedsukontorn, T., Ueno, T., Saito, N., and Hunsom, M. (2017). Facile preparation of defective black TiO2 through the solution plasma process: effect of parametric changes for plasma discharge on its structural and optical properties. J. Alloys Compd. 726, 567–577. doi: 10.1016/j.jallcom.2017.08.028
Kang, Q., Cao, J., Zhang, Y., Liu, L., Xu, H., and Ye, J. (2013). Reduced TiO2 nanotube arrays for photoelectrochemical water splitting. J. Mater. Chem. A 1, 5766–5774. doi: 10.1039/c3ta10689f
Kim, D. S., Chung, D. J., Bae, J., Jeong, G., and Kim, H. (2017). Surface engineering of graphite anode material with black TiO2−x for fast chargeable lithium ion battery. Electrochim. Acta 258, 336–342. doi: 10.1016/j.electacta.2017.11.056
Lee, K., Mazare, A., and Schmuki, P. (2014). One-dimensional titanium dioxide nanomaterials: nanotubes. Chem. Rev. 114, 9385–9454. doi: 10.1021/cr500061m
Leshuk, T., Linley, S., and Gu, F. (2013). Hydrogenation processing of TiO2 nanoparticles. Can. J. Chem. Eng. 91, 799–807. doi: 10.1002/cjce.21745
Li, G., Lian, Z., Li, X., Xu, Y., Wang, W., Zhang, D., et al. (2015). Ionothermal synthesis of black Ti3+-doped single-crystal TiO2 as an active photocatalyst for pollutant degradation and H2 generation. J. Mater. Chem. A 3, 3748–3756 doi: 10.1039/C4TA02873B
Li, Z., Bian, H., Xiao, X., Shen, J., Zhao, C., Lu, J., et al. (2019). Defective black TiO2 nanotube arrays for enhanced photocatalytic and photoelectrochemical applications. ACS Appl. Nano Mater. 2, 7372–7378. doi: 10.1021/acsanm.9b01878
Liu, L., Yu, P. Y., Chen, X., Mao, S. S., and Shen, D. Z. (2013). Hydrogenation and disorder in engineered black TiO2. Phys. Rev. Lett. 111:065505. doi: 10.1103/PhysRevLett.111.065505
Liu, Y., Tian, L., Tan, X., Li, X., and Chen, X. (2017). Synthesis, properties, and applications of black titanium dioxide nanomaterials. Sci. Bull. 62, 431–441. doi: 10.1016/j.scib.2017.01.034
Mazare, A., Park, J., Simons, S., Mohajernia, S., Hwang, I., Yoo, J. E., et al. (2019). Black TiO2 nanotubes: efficient electrodes for triggering electric field-induced stimulation of stem cell growth. Acta Biomater. 97, 681–688. doi: 10.1016/j.actbio.2019.08.021
Mohamed, H. H., and Bahnemann, D. W. (2012). The role of electron transfer in photocatalysis: fact and fictions. Appl. Catal. B 128, 91–104. doi: 10.1016/j.apcatb.2012.05.045
Naldoni, A., Altomare, M., Zoppellaro, G., Liu, N., Kment, Š., Zboril, R., et al. (2019). Photocatalysis with reduced TiO2: from black TiO2 to cocatalyst-free hydrogen production. ACS Catal. 9, 345–364. doi: 10.1021/acscatal.8b04068
Ni, W., Li, M., Cui, J., Xing, Z., Li, Z., Wu, X., et al. (2017). 808 nm light triggered black TiO2 nanoparticles for killing of bladder cancer cells. Mater. Sci. Eng. C 81, 252–260. doi: 10.1016/j.msec.2017.08.020
Ou, G., Xu, Y., Wen, B., Lin, R., Ge, B., Tang, Y., et al. (2018). Tuning defects in oxides at room temperature by lithium reduction. Nat. Commun. 9:1302. doi: 10.1038/s41467-018-03765-0
Pan, J., Dong, Z., Wang, B., Jiang, Z., Zhao, C., Wang, J., et al. (2019). The enhancement of photocatalytic hydrogen production via Ti3+ self-doping black TiO2/g-C3N4 hollow core-shell nano-heterojunction. Appl. Catal. B 242, 92–99. doi: 10.1016/j.apcatb.2018.09.079
Plodinec, M., Grčić, I., Willinger, M. G., Hammud, A., Huang, X., PanŽić, I., et al. (2019). Black TiO2 nanotube arrays decorated with Ag nanoparticles for enhanced visible-light photocatalytic oxidation of salicylic acid. J. Alloys Compd. 776, 883–896. doi: 10.1016/j.jallcom.2018.10.248
Qingli, W., Zhaoguo, Z., Xudong, C., Zhengfeng, H., Peimei, D., Yi, C., et al. (2015). Photoreduction of CO2 using black TiO2 films under solar light. J. CO2 Util. 12, 7–11. doi: 10.1016/j.jcou.2015.09.001
Rajaraman, T. S., Parikh, S. P., and Gandhi, V. G. (2020). Black TiO2: a review of its properties and conflicting trends. Chem. Eng. J. 389:123918. doi: 10.1016/j.cej.2019.123918
Ren, R., Wen, Z., Cui, S., Hou, Y., Guo, X., and Chen, J. (2015). Controllable synthesis and tunable photocatalytic properties of Ti3+-doped TiO2. Sci. Rep. 5:10714. doi: 10.1038/srep10714
Schlesinger, H. I., Brown, H. C., Finholt, A. E., Gilbreath, J. R., Hoekstra, H. R., and Hyde, E. K. (1953). Sodium borohydride, its hydrolysis and its use as a reducing agent and in the generation of hydrogen. J. Am. Chem. Soc. 75, 215–219. doi: 10.1021/ja01097a057
Seok, D., Il, W.u M., Shim, K. B., Kang, Y., and Jung, H. K. (2016). High-rate performance of Ti3+ self-doped TiO2 prepared by imidazole reduction for Li-ion batteries. Nanotechnology 27, 1–5. doi: 10.1088/0957-4484/27/43/435401
Sinhamahapatra, A., Jeon, J. P., and Yu, J. S. (2015). A new approach to prepare highly active and stable black titania for visible light-assisted hydrogen production. Energy Environ. Sci. 8, 3539–3544. doi: 10.1039/C5EE02443A
Song, H., Li, C., Lou, Z., Ye, Z., and Zhu, L. (2017). Effective formation of oxygen vacancies in black TiO2 nanostructures with efficient solar-driven water splitting. ACS Sustain. Chem. Eng. 5, 8982–8987. doi: 10.1021/acssuschemeng.7b01774
Su, J., Zou, X., and Chen, J. S. (2014). Self-modification of titanium dioxide materials by Ti3+ and/or oxygen vacancies: new insights into defect chemistry of metal oxides. RSC Adv. 4, 13979–13988. doi: 10.1039/C3RA47757F
Takanabe, K. (2017). Photocatalytic water splitting: quantitative approaches toward photocatalyst by design. ACS Catal. 7, 8006–8022. doi: 10.1021/acscatal.7b02662
Tan, H., Zhao, Z., Niu, M., Mao, C., Cao, D., Cheng, D., et al. (2014). A facile and versatile method for preparation of colored TiO2 with enhanced solar-driven photocatalytic activity. Nanoscale 6, 10216–10223. doi: 10.1039/C4NR02677B
Tian, M., Mahjouri-Samani, M., Eres, G., Sachan, R., Yoon, M., Chisholm, M. F., et al. (2015). Structure and formation mechanism of black TiO2 nanoparticles. ACS Nano 9, 10482–10488. doi: 10.1021/acsnano.5b04712
Ullattil, S. G., Narendranath, S. B., Pillai, S. C., and Periyat, P. (2018). Black TiO2 nanomaterials: a review of recent advances. Chem. Eng. J. 343, 708–736. doi: 10.1016/j.cej.2018.01.069
Ullattil, S. G., Thelappurath, A. V., Tadka, S. N., Kavil, J., Vijayan, B. K., and Periyat, P. (2017). A sol-solvothermal processed ‘Black TiO2' as photoanode material in dye sensitized solar cells. Sol. Energy 155, 490–495. doi: 10.1016/j.solener.2017.06.059
Wajid Shah, M., Zhu, Y., Fan, X., Zhao, J., Li, Y., Asim, S., et al. (2015). Facile synthesis of defective TiO2−x nanocrystals with high surface area and tailoring bandgap for visible-light photocatalysis. Sci. Rep. 5:15804. doi: 10.1038/srep15804
Wang, B., Shen, S., and Mao, S. S. (2017). Black TiO2 for solar hydrogen conversion. J. Materiomics 3, 96–111. doi: 10.1016/j.jmat.2017.02.001
Wang, C. C., and Chou, P. H. (2016). Effects of various hydrogenated treatments on formation and photocatalytic activity of black TiO2 nanowire arrays. Nanotechnology 27, 1–9. doi: 10.1088/0957-4484/27/32/325401
Wang, Z., Yang, C., Lin, T., Yin, H., Chen, P., Wan, D., et al. (2013). Visible-light photocatalytic, solar thermal and photoelectrochemical properties of aluminium-reduced black titania. Energy Environ. Sci. 6, 3007–3014. doi: 10.1039/c3ee41817k
Wen, J., Li, X., Liu, W., Fang, Y., Xie, J., and Xu, Y. (2015). Photocatalysis fundamentals and surface modification of TiO2 nanomaterials. Chinese J. Catal. 36, 2049–2070. doi: 10.1016/S1872-2067(15)60999-8
Xin, X., Xu, T., Yin, J., Wang, L., and Wang, C. (2015). Management on the location and concentration of Ti3+ in anatase TiO2 for defects-induced visible-light photocatalysis. Appl. Catal. B 176–177, 354–362. doi: 10.1016/j.apcatb.2015.04.016
Xu, J., Zhang, J., Cai, Z., Huang, H., Huang, T., Wang, P., et al. (2019). Facile and large-scale synthesis of defective black TiO2−x (B) nanosheets for efficient visible-light-driven photocatalytic hydrogen evolution. Catalysts 9, 2–9. doi: 10.3390/catal9121048
Yan, X., Li, Y., and Xia, T. (2017). Black titanium dioxide nanomaterials in photocatalysis. Int. J. Photoenergy 2017:8529851. doi: 10.1155/2017/8529851
Yang, Y., Shi, W., Liao, S., Zhang, R., and Leng, S. (2018). Black defect-engineered TiO2 nanocrystals fabricated through square-wave alternating voltage as high-performance anode materials for lithium-ion batteries. J. Alloys Compd. 746, 619–625. doi: 10.1016/j.jallcom.2018.02.309
Ye, M., Jia, J., Wu, Z., Qian, C., Chen, R., O'Brien, P. G., et al. (2017). Synthesis of black TiOx nanoparticles by Mg reduction of TiO2 nanocrystals and their application for solar water evaporation. Adv. Energy Mater. 7:1601811 doi: 10.1002/aenm.201601811
Zhang, K., and Park, J. H. (2017). Surface localisation of defects in black TiO2: enhancing photoactivity or reactivity. J. Phys. Chem. Lett. 8 199–207 doi: 10.1021/acs.jpclett.6b02289
Zhao, J., Li, Y., Zhu, Y., Wang, Y., and Wang, C. (2016). Enhanced CO2 photoreduction activity of black TiO2-coated Cu nanoparticles under visible light irradiation: role of metallic Cu. Appl. Catal. A Gen. 510, 34–41. doi: 10.1016/j.apcata.2015.11.001
Zheng, Z., Huang, B., Meng, X., Wang, J., Wang, S., Lou, Z., et al. (2013). Metallic zinc- assisted synthesis of Ti3+ self-doped TiO2 with tunable phase composition and visible-light photocatalytic activity. Chem. Commun. 49, 868–870. doi: 10.1039/C2CC37976G
Zhi, J., Yang, C., Lin, T., Cui, H., Wang, Z., Zhang, H., et al. (2016). Flexible all solid state supercapacitor with high energy density employing black titania nanoparticles as a conductive agent. Nanoscale 8, 4054–4062. doi: 10.1039/C5NR08136J
Zhu, G., Shan, Y., Lin, T., Zhao, W., Xu, J., Tian, Z., et al. (2016). Hydrogenated blue titania with high solar absorption and greatly improved photocatalysis. Nanoscale 8, 4705–4712. doi: 10.1039/C5NR07953E
Keywords: black TiO2, chemical reduction, defect chemistry, photocatalysis, visible light irradiation
Citation: Andronic L and Enesca A (2020) Black TiO2 Synthesis by Chemical Reduction Methods for Photocatalysis Applications. Front. Chem. 8:565489. doi: 10.3389/fchem.2020.565489
Received: 25 May 2020; Accepted: 24 September 2020;
Published: 17 November 2020.
Edited by:
Khalid Z. Elwakeel, Jeddah University, Saudi ArabiaReviewed by:
Mohd Hafiz Dzarfan Othman, University of Technology Malaysia, MalaysiaMoamen S. Refat, Taif University, Saudi Arabia
Copyright © 2020 Andronic and Enesca. This is an open-access article distributed under the terms of the Creative Commons Attribution License (CC BY). The use, distribution or reproduction in other forums is permitted, provided the original author(s) and the copyright owner(s) are credited and that the original publication in this journal is cited, in accordance with accepted academic practice. No use, distribution or reproduction is permitted which does not comply with these terms.
*Correspondence: Luminita Andronic, YW5kcm9uaWMtbHVtaW5pdGFAdW5pdGJ2LnJv