- 1Department of Biosciences and Bioengineering, Indian Institute of Technology Bombay, Mumbai, India
- 2Centre for Research in Nanotechnology and Science, Indian Institute of Technology Bombay, Mumbai, India
- 3Department of Chemical Engineering, Thadomal Shahani Engineering College, Mumbai, India
Cancer has been widely investigated yet limited in its manifestation. Cancer treatment holds innovative and futuristic strategies considering high disease heterogeneity. Chemotherapy, radiotherapy and surgery are the most explored pillars; however optimal therapeutic window and patient compliance recruit constraints. Recently evolved immunotherapy demonstrates a vital role of the host immune system to prevent metastasis recurrence, still undesirable clinical response and autoimmune adverse effects remain unresolved. Overcoming these challenges, tunable biomaterials could effectively control the co-delivery of anticancer drugs and immunomodulators. Current status demands a potentially new approach for minimally invasive, synergistic, and combinatorial nano-biomaterial assisted targeted immune-based treatment including therapeutics, diagnosis and imaging. This review discusses the latest findings of engineering biomaterial with immunomodulating properties and implementing novel developments in designing versatile nanosystems for cancer theranostics. We explore the functionalization of nanoparticle for delivering antitumor therapeutic and diagnostic agents promoting immune response. Through understanding the efficacy of delivery system, we have enlightened the applicability of nanomaterials as immunomodulatory nanomedicine further advancing to preclinical and clinical trials. Future and present ongoing improvements in engineering biomaterial could result in generating better insight to deal with cancer through easily accessible immunological interventions.
Introduction
Cancer is a notable disease indexing high mortality rate worldwide as statistics had estimated approximately 18 million emerging cases and 9.6 million deaths during 2018 (Bray et al., 2018; Siegel et al., 2020). Amongst which Asian countries, lung cancer in male and breast cancer in female leads the chart with several folds. Surveillance data has reported to be limited in the countries that state epidemic at its early stage. Poor prognoses, diagnosis, and treatment in the view of variation amongst population could be relatable reason for such restrictions (Bray et al., 2018). Trend forecasting intrinsic and extrinsic factors are causative of fluctuation in cancer demography. Likewise, age-based cancer analysis has stipulated to reveal 89,500 new cases and 9,270 deaths in United States alone during the year 2020 with individuals between 15 and 39 years (Miller et al., 2020). Program such as Cancer Moonshot 2020 further proposes to awaken a diverse research community to keep working towards more potentially driven therapeutic strategies (Wang, 2016). Ahead of this, marked as inexplicable due to difficulty in diagnosis and recurrence, developing effective strategies to deal with cancer is in enormous demand. Individual conventional therapies such as chemo, radiation, and surgical methods are not sufficient to fully cure cancer. Another concept as immunotherapy evolved through assessing defensive behavioral responses resulting from the natural process of immune system displayed when they are on the verge to encounter malicious tumors by activation, modulation, suppression, etc. activities (Song et al., 2017). Coordination of different immune cells including Dendritic cells (DCs), NK cells, T-cells and macrophages results to induce variable reposes. Wherein the NK cells release porphyrin and granzyme toxin collectively into the target cells enhancing tumor apoptosis. T-cells are primary contributors of adaptive immune system. They are grouped into subsets according to the cluster of differentiation molecules expressed and role in immune system. Like T-cells, macrophages are also classified based on the functions they perform. M1 macrophages activate to secrete some pro-inflammatory cytokines participating in tumor cell destruction. In contrast, M2 macrophages work to produce anti-inflammatory cytokine combating the inflammatory response. DCs function mainly in processing and presenting antigen to T-cells facilitating antitumor immunity (Chulpanova et al., 2020). Collectively, immunotherapy for cancer is upsurging as the golden key by regulating the immune system and unraveling difficulties of personalized medicine. With the enhancement of immune strength being considered as rational of immunotherapy, immunomodulatory cancer theranostics aims to achieve the unmet clinical needs for providing effective antitumor therapy protruding minimum off-target effects in a reliable way covering a wide range of heterogeneous cells (Nam et al., 2019). One way to classify immunomodulation is based on the response they tend to produce. Specific modulation uses molecules like adjuvants directed towards activating target of interest, especially B-cells and T-cells but might result in ineffective mobilization. Whereas, the non-specific molecules like cytokines act as a depot for sustained release to boost the immune system and address symptomatic issues when the underlying cause is unclear. At times, such type of immunomodulation could be more potent often generating a better subtherapeutic effect than specific modulations but provoke severe immunotoxicity (Fang and Zhang, 2016). Apart from primary cells, discrete components of the immune system like mediators, checkpoints, tumor-associated macrophages (TAMs), Tumor-associated fibroblasts (TAFs), toll-like receptors (TLRs), myeloid-derived suppressor cells (MDSCs), nucleotide-binding oligomerization domain (NOD)-like receptors (NLRs), retinoic acid-inducible gene I (RIG-I)-like receptors (RLRs), and C-type lectins, etc. could also be utilized for immunomodulation (Song et al., 2017; Le et al., 2019). On the other side, immune response and related activities could also be supported by well-constructed nanobiomaterial having good carrier capacity. Scheme of tumor destruction by different nanomaterials and their modifications synergized with immune cell recruitment has been illustrated in Figure 1. A more comprehensive correlation of theranostic nanomaterial and immunomodulation will be discussed in the following sections.
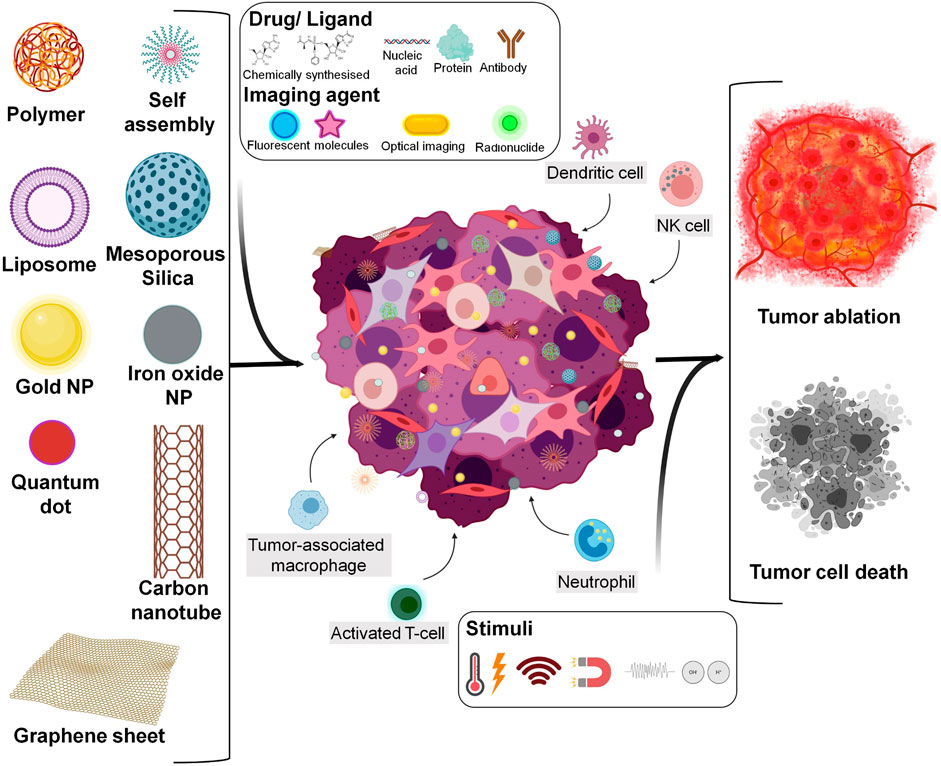
FIGURE 1. Overview stating types of engineered nanomaterial synchronised with immune cell activation for tumor destruction (Created with BioRender.com).
Nanomaterial is a widely researched domain to transform various aspects of technology. Theranostics, an integration of therapeutically active treatment and diagnosis of disease are an important application of nanomaterials. During the last two decades, many nanomedicines got clinically approved and many more are under investigation in which theranostic acts as carrier systems (Peer et al., 2007; Davis et al., 2008). Specifically, in biomedical domain, this combination of nanomaterial-based theranostics with immunotherapy has opened avenues in disease management (Kievit and Zhang, 2011; Kaushik and Nair, 2018; Nam et al., 2019). In nanotheranostic preparation, nanoparticles (NPs) are often loaded with therapeutic drugs, proteins, photothermal agents, imaging moieties, or immunoactive molecules. To achieve multitasking activities, the particle should display unique characteristic properties and tenability. Different steps to functionalize and tag molecules with NPs have gained prominence introducing new advantages of nanoformulation (Liu et al., 2018; Overchuk and Zheng, 2018). Depending on the route of administration, the NPs with attached recognizable ligands are readily taken up by the targeted cells following receptor-ligand interaction (Song et al., 2017). The NPs capability to flow along with bloodstream or obstruction during circulation depends on enhanced permeation and retention (EPR) effects. However, in most cases complex biological barriers encountered by NP limits the bioavailability of active agents as they get distributed to even undesired sites. Opsonization, blood platelets, protein plasma, coagulation factors, phagocytic system and cellular internalization all contribute to the formation of protein corona (layer of proteins adsorbed) on NP surface while passing through vascular compartment (Longmire et al., 2008; Blanco et al., 2015). Advertently, creating a high hindrance to activity and selectivity of the targeting ligands attached to the surface of NP by further reducing therapeutic outcome (Dutta et al., 2007; Salvati et al., 2013; Obst et al., 2017; Mendes et al., 2018). All of this unsolicited phenomenon can be dealt with by finely tuning the NPs to envelop drug from the reactive surrounding. Even the physiological conditions in which the tumor cells grow and proliferate has also been an important aspect of research. The tumor microenvironment (TME) is quite complex and different among tumor kinds thus greater challenges are faced by theranostic NPs during cellular penetration to deliver the cargo (Roma-Rodrigues et al., 2019). With continuous advancements of clinical oncology from the perspective of high throughput research, many biomarkers have been identified that are exclusively expressed on the tumor cells (Mendes et al., 2018). Few are found in typical TME, thus can be distinctively targeted by the NPs upon reaching the tumor site and significantly improving the treatment efficacy (Hamid et al., 2011; Yuan et al., 2016; Roma-Rodrigues et al., 2019). Biomarkers presented by tumor cell and their persisting microenvironment enforce the requirement to schematize non-invasive techniques through utilizing their property of acting as a receptor to various ligands (Yuan et al., 2016; George et al., 2019). This has been the foundation of nano-based objectives to achieve maximum tumor eradication.
For facilitating stimulation or suppression of immune responses, the NPs are combined with various type of immune system derived or based materials/immunogenic, like antigens, adjuvants, DNA/RNA sequence, peptides, interleukins, cytokines, the stimulator of interferon genes (STING), TLR antagonists, and indoleamine 2,3-diooxygenase (IDO) inhibitors, growth factors, etc. These immunomodulators subjected to design immunomodulatory nanoformulations either use standalone or combinational approaches. (Le et al., 2018; Gorbet and Ranjan, 2020). Some materials have intrinsic immunomodulation properties exhibiting basic characteristics and thus lead as an efficient material in synthesizing immunomodulatory cancer theranostics (Song et al., 2017). To further enhance the efficacy of immunomodulatory agents, few co-delivery agents have also been investigated. They intend to accompany and strengthen the activity of low response generating immunomodulators (Le et al., 2019). Substantially in immunological treatments for dealing with receptor expression level, non-invasive imaging techniques of ultrasound, Computer Tomography (CT), Magnetic Resonance Imaging (MRI), Positron Emission Tomography (PET), optical imaging and, fluorescence using probes and visualization features have turned out to be potentially viable platforms (Ehlerding et al., 2016; Zavaleta et al., 2018; Kasten et al., 2019). Moreover, multifunctional theranostics can help in observing the actual route of precision nanomedicines which when administered to screened tumors collectively segregated themselves as per enhanced EPR effect (Jiang et al., 2017; Nam et al., 2019). To gain information about real-time response and disease progression, molecular imaging has accounted to facilitate the elimination of ineffective treatment regimes (Ehlerding et al., 2016). Diagnosis involving therapeutic guidance with imaging tool, aids in monitoring engineered NP loaded with cargo. Further, revealing the steps covered by carrier system both inside out of the targeted site like uptake of nanomedicine and intratumoral distribution helps in systematically developing a standard protocol (Chen H. et al., 2017). If incorporated externally, the diagnostic compound should remain stable and protected until the acting constituent gets unloaded at the targeted site (Hossen et al., 2019). Hence, controlled optimization of NPs circumscribes the future of immunomodulating nanomaterial supporting both anticancer drug and bioimaging tracer with the ability to withstand the body’s internal pressure and other interactions.
The integration of cancer with immunotherapy has introduced investment as a burden for meeting cost-effective maximum therapeutic response and lower toxicity. Looking at the proficiency required in attaining the desired efficacy of immunologically active small molecules, various alterations in nanotechnology have been opted to achieve enhanced biodistribution, localization, and kinetics (Goldberg, 2015). A comparatively simple solution to modify nanomaterial as expected, nanotechnology has offered reliable perspectives of working on theranostic. Dealing with immunotherapy and diagnostics concurrently to meet the challenging demands of cancer could commence acceptable clinical results. Successfully planned and designed treatment may also deter the imperfections in clinics that critically lack analytical response uprooting variations in immunomodulatory nanomedicine (Chen H. et al., 2017). Additionally, attaining uniform NPs characteristics throughout the nanomedicine formulation when considering personalized treatment could be advantageous. Before radiating to approaches used in engineering cancer theranostic, lets dive into understanding the properties of different nanomaterial associated carrier systems closely.
Classification and Characteristics of Nanomaterials for Cancer Immunotheranostic
Multiple novel platforms are gaining interest in elucidating sophisticated solutions to treat cancer with emerging nanotechnology connecting immune response (Waldman et al., 2020). Nanotechnology interventions can also augment flexible biomaterials with designs related to additive manufacturing (Ghomi et al., 2020). However, with technical advancements, control over physicochemical parameters such as size, surface functionalization, shape and, charge paramount stimuli response as well as loading efficiency of nanomaterials. Variation in properties influences the underlying fate and mechanism of NPs at the target site (Ferrari, 2010; Fang and Zhang, 2016). Surface modifications leading to structural changes can be attained through different constructions of layers at the surface, shell, or in the core of NPs. Such alterations are executed by processing through metal ions, electrolytes, and surfactants (Shin et al., 2016). Tumor penetration and distribution enhances at NP size of 100 nm or less. Representing good antitumor activity, NPs at a much smaller dimension of 30 nm have been reported to enter even the poorly permeable tumor (Cabral et al., 2011). Another property of shape and charge states a reliable predictor for designing improved nanocarriers with reduced toxicity. When studied with different nanoscale geometries, under typical conditions the nanodiscs with hydrophilic anionic charge of high aspect ratio were found to be more internalized in epithelial and immune cells as compared to nanorods. Also, larger nanodisc and nanorods showed better uptake than smaller counterparts (Agarwal et al., 2013). The ratio of positive and negative surface charges for monodispersed and stable particles has also been evaluated as a deciding factor depending on the nature of the cargo to be delivered in vivo (Kranz et al., 2016). Functionalization of the material surface has been another important aspect to achieve effective targeting enabling binding of many ligands confronting optimum blood clearance and therefore sustainable half-life (Longmire et al., 2008; Fang and Zhang, 2016).
Like traditional material classification, immunomodulatory nanomaterials for cancer theranostics can also be broadly divided into three categories based on the core materials used: inorganic, organic (Mendes et al., 2018), and inorganic/organic hybrid NPs (Vivero-Escoto and Huang, 2011; Liang et al., 2013). Accompanied by considerable delivery criteria, drug delivery attempts to expedite synthesized multimodality nanosystems by controlled selection of NPs attributes. Despite such achievable goals, there is a need to optimize material choice depending on the type and nature of the drug delivery system for cancer immunotherapy.
Inorganic Nanoparticles
Inorganic nanoparticles have exhibited an excellent potentials in the field of cancer theranostics with versatile functionalization properties. Gold, silver, iron-based NPs, quantum dots, etc. are widely studied as some important classes of inorganic NPs (Mendes et al., 2018). They have largely been explored for their imaging, magnetic, radiation-controlled, and hyperthermic properties (Mendes et al., 2018). Apart from being bioinert, gold demonstrates the ease of processing by surface modification and can be crafted into different shapes and sizes. Gold NPs with their intrinsic optical properties are being used in therapeutic and diagnostic applications (Huang et al., 2007; Shi et al., 2014). Due to this, gold NPs have mostly been investigated for both hyperthermia based therapeutic treatments and contrast based imaging using MRI and PET/CT scans (Medina-Reyes et al., 2017). Moreover, gold have turned out to be the most explored metallic NPs that can stimulate the immune system and deliver drugs, antigen, nucleotides, aptamers, etc. The second type of inorganic NPs, quantum dots (QDs) are conventional yet effective in its applciations. They represent nanosystems in the range of 2–10 nm built by different materials including carbon, zinc sulfide, titanium oxide, etc. They generally pack into layers giving the appearance of small dots that can be easily coated on other nanosystems forming good imaging agents (Medina-Reyes et al., 2017). With the currently available methods including green synthesis it has become possible to control size, functionalize, and modify QDs. Iron Oxide NPs hold an important position in the field of nanotechnology for generating radiofrequency and magnetic effect-based hyperthermia which is sublethal at temperature <43°C. Surface modification and high contrast properties of iron oxide NPs give easy access to imaging and diagnostics purposes with MRI and CT scans. Application has been observed in initiating immunotherapy simultaneous to activation of heat shock proteins enhancing immune responses (Medina-Reyes et al., 2017; Singh et al., 2019). Radionuclide in medicine is known as radio nanomedicine abd use radiolabeled nuclei constituting a special group of inorganic NPs. They are used as molecular imaging modalities in PET, X-Rays and Single Photon Emission Computed Tomography (SPECT) for bioimaging the target sites and photodynamic therapy (PDT). Radionuclides like Gadolinium, Hafnium, Yttrium, Lutetium have entered clinical trials as theranostic systems for radiotherapy providing treatment in combination with immunologic agents (Ferreira et al., 2019). Biocompatible silica NPs are another class of inorganic materials having size ranging from 1 to 200 nm. They have been explored in many nanosystems in the form of rod-like, non-spherical shaped, and mesoporous silica NPs. High loading capabilities, easy surface modification, and targeting methodologies add to their characteristics. Further, they are widely being used for bioadhesives, drug delivery, tissue imaging, and diagnosis purposes (Medina-Reyes et al., 2017; Xu et al., 2017). In cancer immunotherapy, porous silica and gold for immunomodulatory activity have been immensely studied. (Nguyen et al., 2012; Almeida et al., 2014; Shahbazi et al., 2015). Besides all the benefits of theranostic, inorganic NPs possess major challenges of toxicity and accumulation in the body, rendering the preference to organic over inorganic materials (Mendes et al., 2018). Facts have been supported by the reports suggesting superparamagnetic iron oxide and quantum dots for causing cancer as they induce immunogenic toxicity in few animal models (Kodali et al., 2013; Mashinchian et al., 2014).
Organic Nanoparticles
With distinctively achievable morphology, excellent biocompatibility and lower toxicity organic NPs have been considered in drug delivery, imaging, and phototherapy for ages. Construction and tailoring of organic NPs can be done through hydrogen bonding, van der Waals, and electrostatic interactions (Fang et al., 2020). They form multiple types of material systems. Conventionally, lipid-based NPs are self-assembled vesicles, consisting of phospholipid amphiphilic molecules which serve as good carriers to deliver huge payloads. Improvement of lipid NPs can be easily done by controlling release rate, functionalizing with PEG for prolonged-release, targeting ligands, and fluorophores in response to some environmental stimulus (Hossen et al., 2019). They can be modified into different carriers like liposomes, micelles, niosomes, exosomes, etc (Medina-Reyes et al., 2017). Highly biocompatible liposomes have been encapsulated with various drugs, imaging, photothermal, photodynamic agents, along with other immunological agents to increase the internalization, retention, and delivery properties of the nanoformulations (Mendes et al., 2018). Apart from lipids, various types of NPs include polymers assemblies. Nanospheres, nanocapsules, dendrimers, micelles, co-polymers conjugates, polymer-lipid conjugates, etc. are few polymeric delivery systems. Polymers have some significant properties like a smooth escape from retinoendothelial system, high loading capacity, stimuli-based release of cargo from the depot and safety. Flexibility in design, diversity and large-scale synthesis makes polymers stand ahead of other organic NPs (Twibanire and Grindley, 2014). Polyethylene glycol (PEG), Polyethylene-L-glycolic acid (PLGA), and Polylactic acid (PLA) are some Food Drug Administration (FDA) approved polymers widely being used in nanoformulations. Nanosystems explicitly imposing properties like good mechanical strength use polymer mixtures to attain desired synergistic physiochemistry and sustainable dependency. A variety of copolymers, metals, and nucleotide-based conjugates are being introduced into multiple approaches (Mendes et al., 2018). Recently, multinuclear polymer has been developed for increasing protein loading capacity and release in a sustained manner without compromising bioactivity (Wu et al., 2014). As another type of organic NPs, carbon-based materials have been classified based on structures, like nanotubes, graphene sheets/quantum dots, fullerenes, etc. They depict unique structural rigidity and electrical properties (Georgakilas et al., 2015). However, studies have shown potential toxicity even at lower carbon concentrations restricting nanotheranostic prerequisites where it fails safety concerns (Medina-Reyes et al., 2017). The naive yet important group represents biomolecule based materials that use biological building blocks like carbohydrates, DNA and peptide as NPs aiming to induce minimum systemic toxicity with optimum desired immune response. Recently evolved and extensively exploring biological derived cell membranes or camouflages have been harnessed as vehicle system. Due to natural extraction, such bounded systems survive for longer in the biological fluids without losing stability and has been reported to easily navigate inside the targeted site (Mendes et al., 2018). However, during the material selection of organic nature, most commonly used and widely preferred are biodegradable lipids and polymer-based NPs (Shargh et al., 2016).
Inorganic/Organic Hybrid Nanoparticles
Overcoming the major drawback of toxic inorganic biomaterial by cheap and simple green chemistry approach has increased their utilization in hybrid systems along with organic materials (Madamsetty et al., 2019). Sufficing the advantages and disadvantages of each other, incorporation of inorganic and organic NPs in single system has added to the cancer immunotherapy development schemes. They use many stimuli-responsive nanocarriers demonstrating controlled drug release profile assisted by external triggers like ultrasound, heat, temperature, pH, magnetic field, and electric field (Mi, 2020). For instance, apoferritin, a protein nanocage having iron at its core shows pH-dependent structural assembly. This fundamental behavior is being highly appreciated in cancer theranostics (Madamsetty et al., 2019). This mixed type NPs can also be used to address covalent and non-covalent linkages with the drug. Superparamagnetic iron oxide NPs in association with polymer or lipid vesicle carriers can gauge the release of drugs under external magnetic field (Alonso et al., 2016; Grillo et al., 2016; Li et al., 2019). Using the ablation property of inorganic NPs like Gold and advantages of Iron oxide with biocompatible characteristics of polymer, a combinational systems have been synthesized and studied for near infrared (NIR)-triggered chemo-photothermal therapy (PTT) (Chen C. W. et al., 2017).
Porphyrin NPs of perfluorocarbon gas-filled lipid shells can be stimulated by low-frequency ultrasound which also acts as photoacoustic and fluorescent contrast imaging agent (Huynh et al., 2015). Magnetic nanogrenades with pH-sensitive ligands have been developed from iron oxide self-assembled NPs which disassemble into highly active subcellular compartments enabling good MR contrast, photodynamic/thermal, and fluorescence activity to detect early stage cancer. This has ventured as effective treatment for drug-resistant neoplastic cells (Ling et al., 2014). A liposomal carrier with ZnS/ZAISe QDs embedded in a phospholipid bilayer, loaded with DOX and later fused with macrophage membranes has shown superior optical imaging while accumulating in lung lesion tissues of mice (Liang et al., 2020). The modern hybrid systems would integrate physicochemical and bio-functionalities for supporting co-therapies by conjugating varieties of therapeutic cargos (Fan et al., 2017). Altogether, looking ahead of these entire advents, hybrid-based smart nanomaterial system could produce safer and superior clinical results with remotely supervised therapeutic, diagnostic and monitoring (Thorat et al., 2019).
To summarise, NPs demonstrate the ability to penetrate inside the tissue via the leaky vasculature and increase the EPR effect. Smaller molecules tend to accumulate faster in tumors and can stay for a longer time. Functionalized NPs could achieve active targeting in the tumor site with the help of proteins, aptamers, peptides, carbohydrates, nucleic acids, etc (Shargh et al., 2016). A sustained and controlled release can be attained effortlessly by using polymeric NPs because the drug release is either via diffusion, swelling, or via bulk erosion in a time-dependent manner. Properly engineered biodegradable nanomaterials have benefits such as maintaining the drug concentration in the desired range for long periods (Singh et al., 2019). The most prominent property of NPs is the capability to resemble multifunctionality and could be beneficial for immunological cancer research. The intention has been to incorporate imaging agent for monitoring the effect of treatment so that précised amount of drug is delivered at the correct location. Table 1 enlists examples of theranostic nanomaterial for delivering or augmenting immunomodulatory effects studied on cancer models.
Approaches for Cancer Immunomodulated Biomaterial System
Malignant cells in the body have ability to proliferate uncontrollably and eventually evade detection by immune system as they genetically modify themselves and continuously recruit immune cells for their growth and development (Gonzalez et al., 2018). However, certain strategies can be adopted to enhance the potential of immune system in fighting back robustly with well-controlled therapeutic approaches based on advanced delivery systems as summarized in Figure 2 (Ridge et al., 1998; Jain et al., 2015; Chen Q. et al., 2016; Deng and Zhang, 2018; Kutova et al., 2019; Chulpanova et al., 2020; Hauge and Rofstad, 2020). Specifically, functionalized biomaterials by physical or chemical bio-conjugation can help to suffice the need of treatment requirements. Enhancing or suppressing the immune response through biomedical involvement can diversify choices in regenerative medicine and cancer immunotherapies (Kim et al., 2020). Selections based on the type of immunomodulation assisted by biomaterial carrier such as a NP with desired properties can be achieved by various methods.
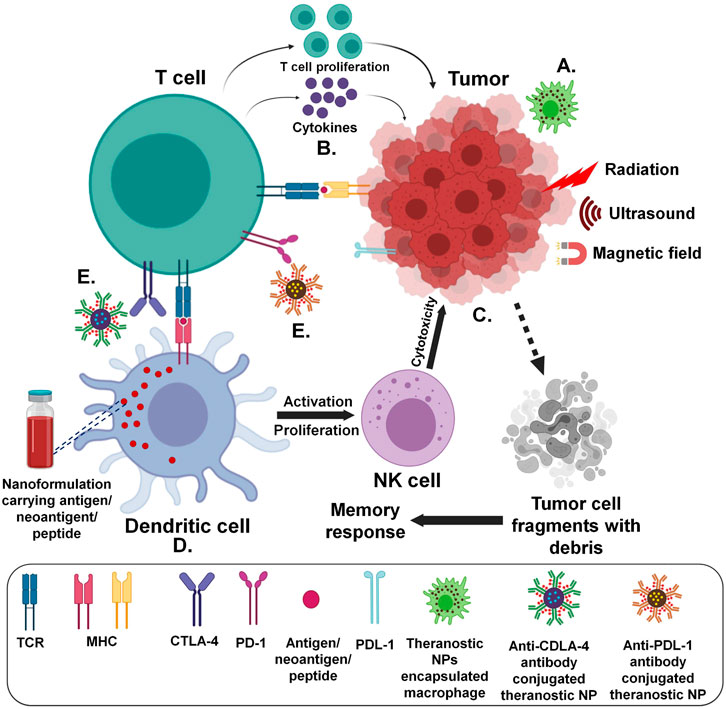
FIGURE 2. Strategies for antitumor immune system targeted delivery of theranostic NPs (Created with BioRender.com) (A) Immune cell/membrane entrapped (B) Direct/indirect cytokine release assisted (C) Tumor microenvironment and components targeted (D) Utilizing antigen presenting cells (E) Antibody mediated.
Immune Cell Utilization
Direct entrapment inside the cell, cell membrane-derived biomaterial coating or delivery by surface attachment provides new and interesting areas of investigation in dealing with cancers effectively (Tan et al., 2015; Fang et al., 2018; Li R. et al., 2018; Li Y. et al., 2019). Immune cells have been incorporated widely as delivery agents for treating many cancers (Wayne et al., 2019). Inhibiting the progression of cancer through the neutralization of circulating tumor cells has also been studied. Apart from engineering nanomaterials for conjugation and directional chemical modifications; advantages of cellular components involved in defense mechanism of the body have been investigated. Kang et al., used drug-loaded poly (lactic-co-glycolic acid) NPs enveloped by neutrophil membranes for managing metastasis by preventing niche genesis (Kang et al., 2017). In another study conducted by Coa et al., a targeted delivery system with NPs of poly (ethylene glycol) methyl ether-block-poly (lactic-co-glycolic acid); PEG-PLGA conjugated celastrol drug further coated by neutrophil membranes were employed to treat pancreatic carcinoma. Neutrophil membrane coated NPs reported to assist in site-specific distribution improving bioavailability. The NPs accumulated as first-line recruitment in the site of inflammation assisting cytokine release (Cao et al., 2019). Recently, one more study was conducted to inhibit cancer by the process of natural destruction mechanism. A nanosized delivery system with Cisplatin loaded pathogen secreted vesicles was recognized and engulfed by neutrophils. The chemical drift generated by the inflamed tumor caused neutrophil migration and subsequent infiltration at the tumor site releasing NPs. Enhanced targeting efficiency and its combination with PTT was shown to effectively irradiate tumor in EMT6 tumor-bearing mice (Li et al., 2020). Macrophages have a better storage capacity and can easily penetrate the solid mass as the tumor progresses rendering them as better drug delivery vehicles. They can even serve to activate pro-drug into active moiety (drug) at the site of release. Miller et al. showed the activated release of fluorescent Platinum (IV) prodrug conjugated with poly (D,L-lactic-co-glycolic acid)-b-poly (ethylene glycol); PLGA-b-PEG by tumor associated macrophages (TAM). The PLGA-b-PEG self-assembly consisted of a hydrophilic PEG shell to enhance the circulation time of unreacted Pt compound and PLGA formed the hydrophobic core of NPs. Imaging was assisted by a fluorophore which acted as an in vivo image analyzer enabling visualization of controlled drug release kinetics by TAM (Miller et al., 2015). Another experiment using fluorescent imaging was performed by Sehwan et al. and co-workers to show the effective uptake and enhanced bioavailability of Ag NPs by macrophage deployed as delivery vehicle (Kim et al., 2020). Polymeric NPs carrying IL-12 cytokines demonstrated the modification from M2 to pro-inflammatory M1 type macrophages (Wang et al., 2017). Engineered nanomaterials have become the latest emerging area of research for inducing antitumor biological response by stimulating immune cells. In a recent experiment, NP assisted artificially reprogrammed machinery was used to not only enhance the macrophage intrinsic activity for effective tumor targeting but were also proposed to develop intramural immunosuppressive resistant macrophages (Li et al., 2019). Hyaluronic acid (HA) with its high affinity toward CD44 protein rich in macrophage was coated on superparamagnetic iron oxide NPs. Macrophages with hyaluronic acid-superparamagnetic iron oxide nanoparticles (HIONs) showed antitumor action by chemotaxis and magnetic movement. HA acted as a medium for effective internalization and chemical signaling response whereas, iron oxide as a mode of activation through magnetic trigger. Both of these examples demonstrated the production of anti-inflammatory factors for tumor repression by rehabilitating M2 to M1 phenotype (Fang and Zhang, 2009; Li et al., 2019). In a similar study, macrophage-mediated PTT assisted by radioiodine-124-labeled gold NPs with crushed gold shells (124I-Au@AuCBs) was examined for colon cancer in mice. It was noted that 124I-Au@AuCBs were retained when taken up by macrophages and hence imaging of the tissues was possible. Ablation of the cancerous tissues substantiated results that the therapy had a significant antitumor effect. The scheme is shown in Figure 3 (Lee et al., 2019).
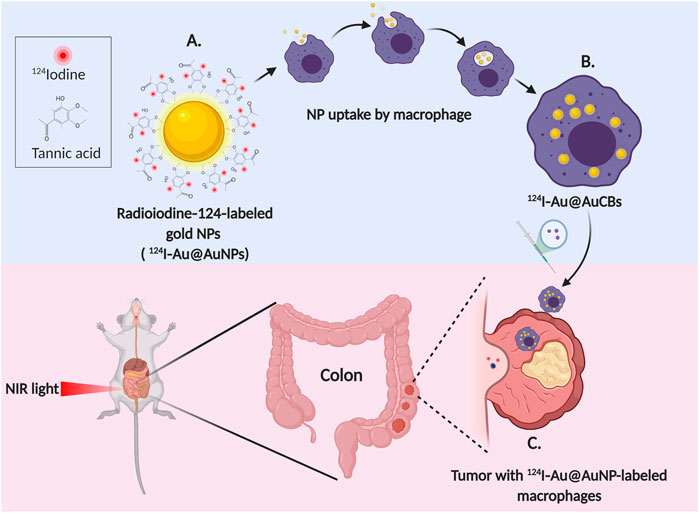
FIGURE 3. Macrophage mediated photothermal therapy and imaging of colon cancer (A) Radioiodine-124-labeled gold nanoparticles with crushed gold shells (124I-Au@AuNPs) (B) Uptake of 124I-Au@AuCBs by macrophage (C) Ablation and imaging of the tumor (Created with BioRender.com).
T cell plays an important role in immune response generation and cancer immunomodulation. One way to achieve T cell activation is by the active cross-presentation of antigen (Tumeh et al., 2014; Rosenberg and Restifo, 2015). For effective cytosolic delivery of antigen to antigen-presenting cell (APC) and then to the targeted cells various NP delivery systems are being explored. PC7A copolymeric NPs labeled with indocyanine blue as an imaging agent have been reported to be an effective tumor antigen delivering agent attaining massive T cell response. This was the cause of increased surface presentation and activation of type I interferon-stimulated genes. Also, when combined with anti-PD-1 antibody, PC7A nanovesicles have shown a significantly higher survival rate in TC-1 tumor model (Luo et al., 2017). The migration, distribution, and kinetics of T-cells can be visualized by labeling with gold NPs as a contrast agent and green fluorescent protein for fluorescence imaging to better understand the physiological interactions between T-cells and tumor cells (Meir et al., 2015). In a study, amphiphilic micelles of conjugated curcumin-polyethylene glycol (CUR-PEG) natural polyphenols were delivered to the tumor site in a murine melanoma model. Combination of CUR-PEG increased CD8+ T-cell cytotoxicity, tumor necrotic factor -α and IFN-γ, with declination of regulatory T-cells (Tregs) was observed (Lu Y. et al., 2016). NK cells are being explored widely in cancer immunotherapy due to their unique feature of distinguishing normal and tumor or infected cells. Unlike T-cell, NK cells don’t rely on tumor antigen or activation response. Ease of extraction, broad specificity, absence of side effect and quick response influence NK cells to adopt with other anticancer therapies (Sun et al., 2009; Vivier et al., 2012). Certain transforming growth factors (TGF) released from tumors inhibits NK cell production and responses. A therapeutic approach has been investigated to downregulate the immunosuppressive signaling pathway blocking the activity of TGF. A Manganese oxide-based nanoparticle system synthesized by reduction and complexation with siRNA has been recently reported. NPs binding to TGFBR2 receptor lead to TGF inactivation causing non-interference in NK cell expression (Adjei et al., 2019). In another research, epidermal growth factor receptor (EGFR) bearing tumor targeted multifunctional engineered PEG-PLGA biocompatible NPs encapsulating drug and NK cell activating agents were nanoconjugated. These NPs designed for controlled release upon systemic administration resulted in activation and accumulation of NK cells mediating antitumor responsive chemoimmunotherapy (Au et al., 2020).
Internalization of Immunostimulatory Cytokines
Long since the role of cytokines manifested in cancer there have been many effective developments as the anticancer advent commenced (West, 1989). The involvement of cytokines in immune cell activation and differentiation has improved the chances of exploration to molecular basis (Lee and Margolin, 2011; Chulpanova et al., 2020). Cytokines including interferons (IFN-γ and IFN-α) and interleukins (GM-CSF, IL-7, IL-12, IL-15, IL-18 and IL-21) can act as competent antitumor mediators whereas, pro-inflammatory cytokines like IL-1, IL-6, IL-10, TNF-α, and TGF-β suppress the immune response thus retarding the antitumor activity (Lee and Margolin, 2011; Burkholder et al., 2014; Yan et al., 2015; Conlon et al., 2019; Chulpanova et al., 2020). IL2 has been investigated for the activation and expansion of NK cells, T-cells, NKT-immune cells (Chung et al., 2014; Boyiadzis et al., 2017; Exley et al., 2017; Yoshida et al., 2017). Individual target cancer immunotherapies involving either stimulation of cytokines antitumor effect or negative regulation of pro-inflammatory immune-suppressive action will assist in enhancing analysis. However, the loopholes of unavoidable systemic pro-inflammatory effects limit cytokines as delivery agents requiring a much more versatile delivery system (Michallet et al., 2004; Pachella et al., 2015). It is also important to include these treatment strategies in combination with immunotherapy to limit toxicity (Melero et al., 2015). In one experiment, cationic polyethylenimine PEI and hydrophilic polyethylene glycol coated dendritic mesoporous silica nanoparticles (PEI-PEG-DMSN) were used to encapsulate TNF-α which had earlier reported to cause undesirable cytotoxicity on normal cells when administered systemically. The pH- responsive copolymer of PEI-PEG carrier system helped in releasing the cytokine cargo into the tumor cell due to acidification digestion. DMSN conjugation with different dye labels of Rhodamine B, Fluorescein and Pacific Blue, tracked delivery and cellular uptake pathway of TNF-α inside the cancerous cell (Kienzle et al., 2017). In another study, hydrophobic TGF- β receptor 1 antagonist was solubilized in methacrylate conjugated β cyclodextrins (CD) which contained hydrolysable ester bond between succinylated-CD and methacrylate group. This in conjunction with a liposome-encapsulated biodegradable polymeric matrix of PLA-PLG through covalent bond facilitated the sustained release of entrapped IL-2 and CD loaded TGF- β. Imaging was carried by rhodamine complexed with functionalized β cyclodextrins (Park et al., 2012). CD137, a member of TNF activates co-stimulatory signal generation of T-cell (Mittler et al., 2004). Liposomes are highly efficient carriers of cytokines (Siegler et al., 2016). Studies have shown that encapsulating IL-2 in multilamellar liposomal carriers significantly increases the circulation times along with decreased hematologic toxicities. The liposomes were able to retain the biologic activity of IL-2 and ensured the same effect while administering 7.5 times higher the dose of free cytokine indicating increase in tissue internalization (Anderson et al., 1992; Siegler et al., 2016). A study to overcome the systemic immunotoxicity of cytokines and circulating lymphocytes was performed through localized delivery involving thiol linkage of IL-2 and anti-CD137 antibody Fc region on the surface of PEGylated Liposome. Here, esterification between InfraRed fluorescent dye and IL-2 or anti-CD137 resulted in tracking tumor penetration (Zhang Y. et al., 2018).
IFNs have well been known in controlling cancers like Kaposi’s sarcoma, melanoma, kidney cancer, and hairy leukemia due to their ability to dually unleash an anti-tumor response and control tumor cell proliferation (Maeda et al., 2014). NPs having an affinity for the anionic membrane of mitochondria, based on a biodegradable poly (lactide-co-glycolide)-b-polyethyleneglycol (PLGA-bPEG) copolymers as a carrier system bonded with triphenylphosphonium (TPP) cation were prepared and linked with zinc phthalocyanine photosensitizer. The system after cascading mitochondria induced apoptotic cell death due to targeted light stimulated delivery of cargo enabled secretion of IL-18 along with IL-12, which eventually led to the production of IFN-γ by DC (Marrache et al., 2013). In a research, silica modified gold nanorods incorporated in cytokine-induced killer (CIK) cells were used for gastric cancer. Through in vivo experiments, it was observed that 4 h post-injection, these CIK cells labeled with gold nanorods could actively target gastric cancer cells MCG803 and image simultaneously using photoacoustic based imaging. An upregulation of cytokines such as IL-1, IL-12, IL-2, IL-4, IL-17, and IFN-γ was observed along with the eradication of gastric cancer tissues by PTT under NIR laser radiation (Yang et al., 2016). Another group studied the combinatorial effect of immunologically active agent cytokine IL-12 and monoclonal antibody cetuximab to treat head and neck carcinoma thus limiting the individual toxicity of cetuximab and IL-12. Preclinical hypothesis suggested safe antitumor action with enhanced immune response by secretion of IFN-γ apart from inducing NK cell-mediated antibody-dependent cellular cytotoxicity (ADCC) (Mcmichael et al., 2019). Comprehensively, cytokines can be delivered efficiently with the help of NPs since they get degraded and cleared rapidly from the system. It is also due to NPs passive accumulation in the leaky vasculature of tumor (Siegler et al., 2016).
Targeting the Tumor Microenvironment
The tumor microenvironment (TME) acts as a major barrier in reaching the targeted site whose negligence leads to inefficient cellular uptake of NPs and connected active moieties accompanying therapy failure. Cancerous heterogeneous cellular complexation with fibroblasts, adipocytes, myofibroblasts, the extracellular matrix (ECM) along with immune cells and the vasculature system complicate the treatment (Albini and Sporn, 2007; Chen et al., 2015). Also, the continuous addition of cells through the upregulation of proangiogenic proteins such as vascular endothelial growth factor (VEGF) brings about endothelial cell migration and proliferation causing an excessive accumulation of endothelial and abnormal perivascular cells in the area of the tumor. Abnormal vascularisation prohibits adequate penetration of anti-cancer agents into the desired site of action ending up in minimal anti-cancer effect (Banerjee et al., 2011; Cesca et al., 2013; Khawar et al., 2014; Klemm and Joyce, 2014). To circumvent this obstacle, various nontoxic biocompatible nanoparticulate delivery systems have been developed. One such stratagem employed by Sengupta et al. involved the development of poly-(lactic-co-glycolic) acid (PLGA) polymeric nanoparticles, where Doxorubicin was covalently bound to the inner core of NP followed by the encapsulation of combretastatin within the outer lipid envelope. On delivery with PLGA (nanocell), combretastatin being an anti-angiogenic agent disrupted cytoskeletal structures leading to a dysfunctional vascular system in TME. This disorientation assisted the unhindered easy entrapment of Doxorubicin inside the cancer cell prolonging therapeutic release for efficient apoptosis by DNA intercalation as compared to only the liposome or the free drug (Sengupta et al., 2005). Anti-fibrotic and extracellular pH targeting can also potentiate cancer therapies like chemotherapy or radiation targeting TME thus stalling tumor progression (Li X. et al., 2017; Hauge and Rofstad, 2020). Solid tumors involve a stiffer ECM which doesn’t allow sufficient penetration of drugs due to the presence of many components. Alteration in one such major constituent, collagen affects tumor cell migration, nutrition supply and oxygen accessibility. Lipoxygenases (LOX) enzymes or matrix metalloproteinases such as LOX-like protein (LOXL2, LOXL4, MMP2, MMP9 and MMP14) and growth factors inducing collagen deposition (eg, VEGF) are HIF-regulated genes and components that can help in remodeling the ECM and tumor fibrosis (Page-McCaw et al., 2007; Kondratiev et al., 2008; Liu T. et al., 2019). Hence, understanding the underlying modification mechanism of ECM components requires insight into sensitizing biomaterial delivery systems (Harisi and Jeney, 2015; Henke et al., 2020). Amphiphilic poly (D, L-lactide-co-glycolide)-block-poly (ethylene glycol); PLGA-b-PEG-COOH copolymer-based NPs were developed by solvent displacement method and then self-assembled for surface modification through carbodiimide chemical bonding covalently linked with LOXAB (LOX inhibiting antibody). NP size of around 220 nm favored passive targeting of tumors, also the LOXAB coating ensured retention and active targeting of the ECM. A higher therapeutic index of almost 50 doses was achieved with lesser processing steps as compared to the soluble anti-LOX anti-bodies without the nanomaterial coating (Kanapathipillai et al., 2012). Another component contributing to the 3D tumor environment in ECM is a polysaccharide, hyaluronic acid (HA). Apart from being utilized as an HA-delivery based platform various anti-HA approaches have been developed to increase tumor infiltration and easy penetration into the TME (Amorim et al., 2020). One such anti-tumor immunotherapy includes hyaluronidase (HAase) supplemented with DCs maturation potentiating PEI/CpG/OVA nanovaccine containing polycationic polyethyleneimine (PEI) delivery vehicle. The nanosystem carried ovalbumin and unmethylated cytosine-phosphate-guanine antigens simultaneously. Thus, provides a promising treatment regime favoring the importance of ECM disrupting agents (Guan et al., 2018).
Tregs are T-cells that prevent the onset of autoimmune diseases by generating tolerance toward autoantigens developing pro-tumorigenic TME. They have found to be associated with the upregulation of immunological biomarkers including immune checkpoint molecules, cytotoxic T-lymphocyte associated protein 4 (CTLA-4), glucocorticoid-induced TNFR family related gene (GITR) and certain T cell activation markers, CD25 and CD69 (Kakita et al., 2012; Schuler et al., 2012; Jie et al., 2013; Lin et al., 2013; Pedroza-Gonzalez et al., 2013; Sugiyama et al., 2013; Han et al., 2014; Scurr et al., 2014; Kurose et al., 2015). A PEG-modified single-walled carbon nanotube possessing glucocorticoid-induced TNFR related receptor (GITR) with labeled NIR-emitting fluorophore was able to efficiently target Tregs in a B16 melanoma model as compared to the splenic Treg or the intratumor non-Treg. Preferential increase in selectivity and efficiency due to the EPR effect along with use of biomarkers enriched in intratumor Treg recognition could be postulated for easy access to targeting T cells in the TME (Sacchetti et al., 2013). Another cell type in TME is Myeloid-derived suppressor cells (MDSCs) which do not mature into immune cells such as granulocytes, DCs, etc but are related to angiogenesis and metastasis (Shvedova et al., 2013; Park et al., 2018; Wang and Mooney, 2018). Indeed, these cells when present in the TME may suppress T cell proliferation and curb the activation of NK cells, while allowing Treg cells to differentiate. So, it becomes necessary to modulate T-cells and precisely design nontoxic NPs performing targeted cargo delivery which can be highly advantageous (Park et al., 2018). Gemcitabine, a potent anticancer agent when modified by Lauroyl contained in PEGylated lipid nanocapsules and tagged by a fluorescent dye, accumulated at the tumor site and spleen resulting in wipe-off populations of MDSCs in EG07- OVA tumors. Moreover, T cell proliferation and CD8+ T cell activation were reported, when the nanosystem was delivered prior to adoptive cellular therapy (Sasso et al., 2016).
Delivery of Antigens for Immune Activation
Vaccines that can work effectively against cancer by stimulating or enhancing an immune response have been trending widely. Cancer vaccines are basically of two types, one is the preventive type and the other is the treatment-based vaccine. The vaccines administered usually are antigen/adjuvant vaccines, viral vectors and DNA vaccines, DC vaccines and whole-cell vaccines (Barra et al., 2019). Among them, DCs act by triggering immune mechanisms against tumors and have been investigated for their potential role in immunogenic vaccines. As primary antigen-presenting cells their major role is in generating an adaptive immune response. Various ways to enhance antigen uptake by DCs thus assisting better antigen recognition for T cell and natural killer cell activation have been explored (Ridge et al., 1998; Kalergis et al., 2001). Various events support evidence that neoantigens could be targeted for an effective antitumor immune response generation (Hu et al., 2018). To maximize the initial response of an anti-cancer immune system, the antigens must be delivered efficiently to lymph nodes for which NPs are being studied as vehicle (Tran et al., 2018; Zhang L.X. et al., 2018).The nanocarrier systems for antigen delivery must display some integral properties. Firstly, a medium-sized nanocarrier ranging from 5-100 nm could help in effective circulation and delivery through the lymphatic vessels to the lymph nodes. Secondly, particle shape turns to play another key role even in antigen delivery. Non-spherical NPs have higher aspect ratios, higher circulation times, better penetration abilities into tumors, and solid tissues along with prolonged margination effects (Manolova et al., 2008). Third, uptake of NPs by cells and activation of immune responses is surface charge-dependent. Positively charged NPs could cause an upsurge in immune responses as compared to negatively charged or neutral carriers. The downside to using them, however, is that they could get immobilized in the negatively charged ECM, reducing their tissue penetration capacities. On the other hand, hemolysis and platelet aggregation could occur in the lymphatic system, also leading to an unpredictable and premature antigen release. Advantageously, DCs in particular take up cationic NPs easily as compared to anionic and neutral carriers (Foged et al., 2005). Li et al. through polymerization reaction synthesized a copolymer named monomethoxy poly (ethylene glycol)-block-poly (2-(diisopropyl amino) ethyl methacrylate)- block-poly (2-(guanidyl) ethyl methacrylate); mPEG-b-PDPA-b-PGEM, PEDG. The nano self-assembly formed in an aqueous solution was reported to get activated in the cationic and acidic environment due to the presence of amino moieties causing a disassembled structure. PEDG nanoparticles represented to be an effective delivering agent by increasing the uptake efficiency of antigens by DCs (Li P. et al., 2017).
In terms of hydrophobicity, PLGA and chitosan have hydrophobic domains that activate the immune system due to their intrinsic adjuvant properties without the need of signal. This suggests hydrophobicity to be another important factor (Da Silva et al., 2009; Shima et al., 2013). The targeted delivery of adjuvants and antigens can be achieved by decorating the surfaces of NPs with specific ligands or antibodies, DNA, siRNA along with different dyes for image-guided combinatorial treatment (Norman Coleman, 1993; Roses and Czerniecki, 2014). In a study, Superparamagnetic iron oxide nanoparticles coupled with doxorubicin, indocyanine green imaging agent were camouflaged with cancer cell membranes providing simultaneous chemotherapy, hyperthermia and radiotherapy. The cancer cell membranes also preserved the surface antigens and other adherent molecules bestowing the system with good biocompatibility and tumor homing ability. The dual-modality imaging ensured the accumulation of the nanosystem in tumor and resulted good anti-cancer effects. It also reprogramed the macrophages from pro-tumor to anti-tumor (Huang Y. et al., 2018). Fluorescently labeled Poly (D, L-lactide-co-glycolide); PLGA NPs carrying toll-like receptor 7 (TLR-7) antagonist imiquimod (R837) in the core was encapsulated within cancer membranes and further reformed by mannose moiety (NP-R@M-M) to enhance uptake by antigen-presenting DCs (Yang et al., 2018). To improvise NP-R@M-M further for limiting tumor relapse, FDA approved PTT agent Indocyanine Green was conjugated, which upon exposure to NIR laser triggered tumor ablation and presented antigen in the form of dead cell fragments, thus helping to establish memory response (Chen Q. et al., 2016). Lipovaxin MM a liposomal formulation consisting multivalent DC-targeted allogenic vaccine for melanoma. Tumor antigens derived from plasma membrane vesicles were modified using a liposomal mixture with a metal chelating lipid 3 (nitrilotriacetic acid)-ditetradecylamine (3NTA-DTDA). A lipid envelope of α-palmitoyl-β-oleoyl-phosphatidylcholine (POPC) enabled superior insertion of the plasma membrane vesicles into the metal chelating lipid. The DMS-5000 DC targeting antibody fragments were then introduced in the metal chelating lipid through the poly-histidine C-terminal tail. The metal chelating linkage in the presence of nickel showed promising results in antigen expression (Gargett et al., 2018).
Targeting Immune Checkpoint Agents
Naoparticles have evolved as delivery agents for immune checkpoint blockade and related activation mechanisms for cancer treatment. Apart from the effective delivery of drugs and vaccines, NPs shielding immune checkpoint inhibitors have been employed to boost immune response with minimized off-target effects. Inhibitors such as Cytotoxic T-lymphocyte associated antigen 4 (CTLA-4) and programmed death-ligand (PD-1/PD-L1) have been major points of focus in tumor immunotherapy due to clinical importance (Deng and Zhang, 2018). Classified in the family of B7 receptor CTLA-4 and PD-1 although have a synergistic inhibitory effect on T-cell activity; differences in distribution, time of action and signaling pathway mark their distinction (Fife and Bluestone, 2008; Keir et al., 2008). Hence, blockage of both CTLA-4 and PD-1 receptor-mediated pathways could result in the depletion of tumor growth through positive regulation of T-cell activity (Leach et al., 1996; Hirano et al., 2005). For example, NP systems like nanovesicles conjugated with PD-1 receptor when bound to tumor cells expressing PD-L1 ligand blocked the PD-1/PD-L1 axis stalling the growth of B16F10 Melanoma. As compared with free nanovesicles; PD-1 nanovesicles (PD-1 NVs) circulated for a longer time with a high accumulation rate in the tumor tissues. Also, the PD-1 NVs were able to reduce the growth of the tumor to a larger extent compared to anti-PD-L1 antibodies in mice with a higher survival rate (Zhang Xud. et al., 2018). Similarly in an investigation, NP based effective immune-checkpoint inhibitor delivery utilized poly (ethylene glycol)-block-poly (D, L-lactide); PEG-PLA nanoparticles comprising of CTLA-4 small interfering RNA (siRNA). Systemic administration of siRNA loaded PEG-PLGA reported an advent in the downregulation of Tregs and an elevation in CD4+ T-cells and CD8+ T-cells (Li et al., 2016). Another study was performed to improve the effect of PDT and deliver small interfering RNA (siRNA) to alter the PD-1/PD-L1 pathway. Blockage of the PD-1 receptor was achieved upon acidic cleavage of self-assembled pH-responsive poly (ethylene glycol)-block-poly (diisopropanol amino ethyl methacrylate-cohydroxyethyl methacrylate) micelleplex, covalently conjugated with Pheophorbide A (PPa) photosensitizer in hydrophobic core called as PDPA-PPa. PPa assisted multimodal imaging and PDT responsiveness. Cationic 1,2-epoxytetradecane alkylated oligoethyleneimine bonded high-affinity anionic siRNA co-self-assembled with PDPA-PPa formed a drug delivery system for Photodynamic cancer immunotherapy (Wang et al., 2016).
In recent work, Emami et al. demonstrated the regime of chemotherapy along with immune checkpoint inhibitors and PTT to increase therapeutic efficacy against cancer (Emami et al., 2019). Amide linkage after PEGylation of lipoic acid conjugated Doxorubicin a potent anticancer drug (LA-PEG-DOX) and anti-PD-L1 antibody (LA-PEG-PD-L1), assisted bond formation. This system was surface coupled with AuNP, a PTT agent through the dithiol covalent attachment and PEG-SH chain coated to stabilize the NPs. Upon NIR irradiation it was observed that chemo-photothermal immunotherapy targeting by PD-L1-AuNP-DOX nanoparticle formulation caused synergistic antitumor effect (Emami et al., 2019). Liposomal hybrid cerasomes containing porphyrin along with cetuximab (anti-EGFR antibody) in conjugation with a dye IRDye8s00CW and an MRI contrast agent DOTA-Gd, this combination of photodynamic therapy and PD-L1 have a synergistic anti-tumor effect, since they not only selectively targeted the tumor by effective accumulation at the site of action but also turned out as a promising strategy in preventing tumor relapse (Li Y. et al., 2018). One more experiment showed the possibility of combining the vaccine with immune checkpoint blockade therapy to retard tumor development (Chen Q. et al., 2016). A recent review comprehends information on emerging therapeutic strategies utilizing the molecular mechanism of reactive oxygen species (ROS) for inducing cancer cell death and apoptosis (Perillo et al., 2020). Oxaliplatin (OxPt), a cancer cell death-inducing agent and dihydroartemisinin (DHA) classified as ROS-producing drug respectively were loaded in core and shell of a nanoscale coordination polymer self-assembled NPs. DHA conjugation with the shell of NCP was supported by cholesterol linked disulfide bond masking it from systemic hydrolysis and reduction. Complete tumor elimination and prolonged memory response resulted when OxPt/DHA NPs along with anti-PD-L1 antibody was co-delivered to a mouse model. Also, the leftovers of cancer cellular content uptake by phagocytes caused activation of immune response thus improving the treatment efficacy (Duan et al., 2019).
Preclinical and Clinical Outcome
After approval of the first liposomal formulation with drug doxorubicin, Doxil® by FDA and EMA, the market has seen the launch of many anticancer regimes (Barenholz, 2012; Leo et al., 2020). Sufficing the emerging need, the paradigm of approval has also come across inclination for theranostics including recently approved Novartis radiopharmaceuticals, Lutathera® ([177Lu]Lu-DOTA-TATE) for treatment of neuroendocrine tumor (Hennrich and Kopka, 2019) and many are being explored in the clinic (Table 2). Along with this, the combination of immunotherapy to widen the effect of nanomedicine for targeting other cells has been in demand to achieve higher order of tumor elimination (Tang et al., 2018; Duan et al., 2019).
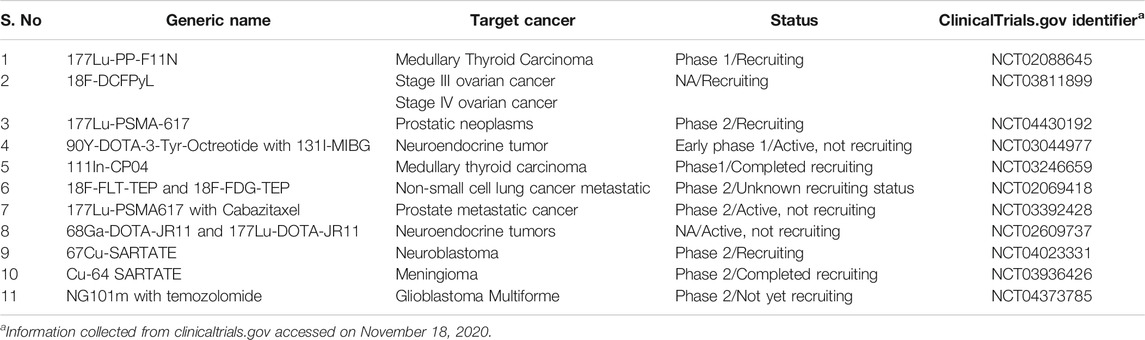
TABLE 2. Recent clinical trials of cancer theranostic as reported by ClinicalTrials.gov.
The relationship between biomaterial and immunotheranostics has opened new investigations for clinical translations. Beyond the challenges of designing simpler nanoformulation incorporating complex multi-functional physicochemical properties of nanomaterial systems including size, shape, charge, composition and stability in preclinical assessment there are other hurdles (Lane et al., 2015). Reproducibility of data with accurate efficacy, scalability, standardization and filtration requires a thorough revision of protocol for improving outcomes in the clinical trial (Faria et al., 2018). Hence, adhering to patient compliance and stratification immunomodulatory nanoformulation should include screening based on acceptable therapeutic potential specifications (van der Meel et al., 2019).
Like all therapeutics, NP clearance has been of fidelity for conducting studies on liver and kidney as major eliminating organs even while assessing their accumulation in undesired areas (Longmire et al., 2008). Toxicity of nanomedicine is the most important criterion to be considered throughout in vitro and in vivo testing among which localized leads systemic delivery as localization produces minimum off-target effect due to focused cellular concentration (Linkov et al., 2008; Wang et al., 2015). More cautiously, systemic toxicity is prioritized when dealing with few antigens like lipopolysaccharides (LPS) (Shetab Boushehri and Lamprecht, 2019; Farhana and Khan, 2020) based on immune cell activation and delivery vehicles having inorganic NPs (Goodman et al., 2004; Maksoudian et al., 2020). Pharmaceutical formulations with biomaterial carrier for anticancer immunotheranostic application after clearance of toxicity test and related profiling which reports desired efficacy in the in vivo studies enters from pre-clinical into clinical phase (van der Meel et al., 2019). The traditional layout of cancer treatments reporting morbidity and mortality is not the only measurable result, but the recurrence rate analysis for rehabilitating healthy conditions has attracted equal importance while performing clinical studies (Chen J. et al., 2016).
Conclusion and Outlook
In this review, we have tried to elaborate the interconnectivity between tailoring nanomaterial for various therapies. Nanoparticle as a single entity can be engineered to transport the therapeutically active agent for killing cancer cells aided by the immune response with real-time visualization tools like bioimaging. However, with diversification of heterogeneous cancerous disease state, treatment measures, and patient profiling the need for biomaterial qualifying for all-purpose has been inclined by a majority. Sophisticated and operational multimodality systems will eventually lead to the path of combining therapeutics and traversing the nanosystems through the complex biological in vivo environment with stability. Hybrid nanosystems demonstrating both therapeutic and diagnosis measures for cancer treatment have been highly influential and incline to expand exponentially.
Immunotherapy with its benefits has been favoring the future outcome due to its role in personalized medicines. Persistence will encompass drift toward synergizing nanomedicine and immunotherapy with relatively cheap, effective, and safer patient compliance than any monotherapy in cancer theranostic. One aspect to achieve this is building a nanobiomaterial system that acts as a carrier with diversified approach to regulate the immune system. The advent of biomaterial selection and design with multifunctional properties is highly recommended for demonstrating satisfactory carrier capability. Although, much of the research has been focused on launching a well-refined immunomodulatory biomaterial system but new emerging therapies and modification to existing strategies will invite revision of in-process protocols. Failure of many theranostics in clinical trial states the need to have better insight relating to immunotherapy and biomaterial for cancer cure. This could be due to the small subset of potential responses received by patients when using immunomodulatory nanohybrid systems. Biosafety and nanotoxicity of biomaterial are few important issues coming in the way of engineering structurally superior delivery systems. Rectification to all this can be achieved by a proper risk assessment of interaction and standardizing adaptable biomaterial to manipulate the immune response bridging the gaps of pre-clinical and clinical trials. A well-engineered biomaterial can even overcome tumor relapse and remission by bringing together the advantages of radiotherapy, chemotherapy, and immunotherapy which remains unexploited. Combined therapies with reduced off-target effects seem to have a much better evolving scope as compared to solely applied conventional methods thus, ushering the world into an advanced era for defeating oncology. Further, reducing immunotoxicity during and after systemic administration targeting tumor site captures more attention toward creating a novel concept in designing next-generation theranostic materials.
Author Contributions
Conceptualization of manuscript, AK; Initial draft, AK, FD, SN, and BS; Final draft, AK; Review, BS, FD, and SN; Final edits, AK.
Funding
This work was supported by the Department of Biotechnology, Government of India.
Conflict of Interest
The authors declare that the research was conducted in the absence of any commercial or financial relationships that could be construed as a potential conflict of interest.
References
Adjei, I. M., Jordan, J., Tu, N., Trinh, T. L., Kandell, W., Wei, S., et al. (2019). Functional recovery of natural killer cell activity by nanoparticle‐mediated delivery of transforming growth factor beta 2 small interfering RNA. J. Interdiscip. Nanomedicine 4, 98–112. doi:10.1002/jin2.63
Agarwal, R., Singh, V., Jurney, P., Shi, L., Sreenivasan, S. V., and Roy, K. (2013). Mammalian cells preferentially internalize hydrogel nanodiscs over nanorods and use shape-specific uptake mechanisms. Proc. Natl. Acad. Sci. U.S.A. 110, 17247–17252. doi:10.1073/pnas.1305000110
Albini, A., and Sporn, M. B. (2007). The tumour microenvironment as a target for chemoprevention. Nat. Rev. Canc. 7 (2), 139–147. doi:10.1038/nrc2067
Almeida, J. P., Figueroa, E. R., and Drezek, R. A. (2014). Gold nanoparticle mediated cancer immunotherapy. Nanomedicine 10, 503–514. doi:10.1016/j.nano.2013.09.011
Alonso, J., Khurshid, H., Devkota, J., Nemati, Z., Khadka, N., K., Srikanth, H., et al. (2016). Superparamagnetic nanoparticles encapsulated in lipid vesicles for advanced magnetic hyperthermia and biodetection. J. Appl. Phys. 119, 083904. doi:10.1063/1.4942618
Amorim, S., Reis, C. A., Reis, R. L., and Pires, R. A. (2020). Extracellular matrix mimics usinghyaluronan-based biomaterials. Trends Biotechnol. 39, 90–104. doi:10.1016/j.tibtech.2020.06.003
Anderson, P. M., Hasz, D., Dickrell, L., and Sencer, S. (1992). Interleukin‐2 in liposomes: increased intravenous potency and less pulmonary toxicity in the rat. Drug Dev. Res. 27, 15–31. doi:10.1002/ddr.430270103
Au, K. M., Par, S. I., and Wa, A. Z. (2020). Trispecific natural killer cell nanoengagers for targeted chemoimmunotherapy. Sci. Adv. 6, eaba8564. doi:10.1126/sciadv.aba8564
Banerjee, D., Harfouche, R., and Sengupta, S. (2011). Nanotechnology-mediated targeting of tumor angiogenesis. Vasc. Cell 3, 3. doi:10.1186/2045-824X-3-3
Barenholz, Y. (2012). Doxil®--the first FDA-approved nano-drug: lessons learned. J. Contr. Release 160, 117–134. doi:10.1016/j.jconrel.2012.03.020
Barra, F., Leone Roberti Maggiore, U., Bogani, G., Ditto, A., Signorelli, M., Martinelli, F., et al. (2019). New prophylactics human papilloma virus (HPV) vaccines against cervical cancer. J. Obstet. Gynaecol. 39, 1–10. doi:10.1080/01443615.2018.1493441
Blanco, E., Shen, H., and Ferrari, M. (2015). Principles of nanoparticle design for overcoming biological barriers to drug delivery. Nat. Biotechnol. 33, 941–951. doi:10.1038/nbt.3330
Boyiadzis, M., Agha, M., Redner, R. L., Sehgal, A., Im, A., Hou, J. Z., et al. (2017). Phase 1 clinical trial of adoptive immunotherapy using "off-the-shelf" activated natural killer cells in patients with refractory and relapsed acute myeloid leukemia. Cytotherapy 19, 1225–1232. doi:10.1016/j.jcyt.2017.07.008
Bray, F., Ferlay, J., Soerjomataram, I., Siegel, R. L., Torre, L. A., and Jemal, A. (2018). Global cancer statistics 2018: GLOBOCAN estimates of incidence and mortality worldwide for 36 cancers in 185 countries. CA Cancer J. Clin. 68, 394–424. doi:10.3322/caac.21492
Burkholder, B., Huang, R. Y., Burgess, R., Luo, S., Jones, V. S., Zhang, W., et al. (2014). Tumor-induced perturbations of cytokines and immune cell networks. Biochim. Biophys. Acta 1845, 182–201. doi:10.1016/j.bbcan.2014.01.004
Cabral, H., Matsumoto, Y., Mizuno, K., Chen, Q., Murakami, M., Kimura, M., et al. (2011). Accumulation of sub-100 nm polymeric micelles in poorly permeable tumours depends on size. Nat. Nanotechnol. 6, 815–823. doi:10.1038/nnano.2011.166
Cao, F., Yan, M., Liu, Y., Liu, L., and Ma, G. (2018). Photothermally controlled MHC class I restricted CD8+ T-cell responses elicited by hyaluronic acid decorated gold nanoparticles as a vaccine for cancer immunotherapy. Adv. Healthcare Mater. 7, e1701439. doi:10.1002/adhm.201701439
Cao, X., Hu, Y., Luo, S., Wang, Y., Gong, T., Sun, X., et al. (2019). Neutrophil-mimicking therapeutic nanoparticles for targeted chemotherapy of pancreatic carcinoma. Acta Pharm. Sin. B. 9, 575–589. doi:10.1016/j.apsb.2018.12.009
Cesca, M., Bizzaro, F., Zucchetti, M., and Giavazzi, R. (2013). Tumor delivery of chemotherapy combined with inhibitors of angiogenesis and vascular targeting agents. Front Oncol. 3, 259–268. doi:10.3389/fonc.2013.00259
Chao, Y., Chen, G., Liang, C., Xu, J., Dong, Z., Han, X., et al. (2019). Iron nanoparticles for low-power local magnetic hyperthermia in combination with immune checkpoint blockade for systemic antitumor therapy. Nano Lett. 19, 4287–4296. doi:10.1021/acs.nanolett.9b00579
Chen, C. W., Syu, W. J., Huang, T. C., Lee, Y. C., Hsiao, J. K., Huang, K. Y., et al. (2017). Encapsulation of Au/Fe3O4 nanoparticles into a polymer nanoarchitecture with combined near infrared-triggered chemo-photothermal therapy based on intracellular secondary protein understanding. J. Mater. Chem. B. 5, 5774–5782. doi:10.1039/c7tb00944e
Chen, H., Zhang, W., Zhu, G., Xie, J., and Chen, X. (2017). Rethinking cancer nanotheranostics. Nat. Rev. Mater. 2, 17024. doi:10.1038/natrevmats.2017.24
Chen, F., Zhuang, X., Lin, L., Yu, P., Wang, Y., Shi, Y., et al. (2015). New horizons in tumor microenvironment biology: challenges and opportunities. BMC Med. 13, 45–13. doi:10.1186/s12916-015-0278-7
Chen, J., Zhang, C., Li, F., Xu, L., Zhu, H., Wang, S., et al. (2016). A meta-analysis of clinical trials assessing the effect of radiofrequency ablation for breast cancer. OncoTargets Ther. 9, 1759–1766. doi:10.2147/OTT.S97828
Chen, Q., Xu, L., Liang, C., Wang, C., Peng, R., and Liu, Z. (2016). Photothermal therapy with immune-adjuvant nanoparticles together with checkpoint blockade for effective cancer immunotherapy. Nat. Commun. 7, 13193–13213. doi:10.1038/ncomms13193
Chen, Q., Liu, L., Lu, Y., Chen, X., Zhang, Y., Zhou, W., et al. (2019). Tumor microenvironment-triggered aggregated magnetic nanoparticles for reinforced image-guided immunogenic chemotherapy. Adv. Sci. 6, 1802134. doi:10.1002/advs.201802134
Cherkasov, V. R., Mochalova, E. N., Babenyshev, A. V., Rozenberg, J. M., Sokolov, I. L., and Nikitin, M. P. (2020). Antibody-directed metal-organic framework nanoparticles for targeted drug delivery. Acta Biomater. 103, 223–236. doi:10.1016/j.actbio.2019.12.012
Chi, J., Ma, Q., Shen, Z., Ma, C., Zhu, W., Han, S., et al. (2020). Targeted nanocarriers based on iodinated-cyanine dyes as immunomodulators for synergistic phototherapy. Nanoscale 12, 11008–11025. doi:10.1039/c9nr10674j
Chulpanova, D. S., Kitaeva, K. V., Green, A. R., Rizvanov, A. A., and Solovyeva, V. V. (2020). Molecular aspects and future perspectives of cytokine-based anti-cancer immunotherapy. Front. Cell Dev. Biol. 8, 402. doi:10.3389/fcell.2020.00402
Chung, M. J., Park, J. Y., Bang, S., Park, S. W., and Song, S. Y. (2014). Phase II clinical trial of ex vivo-expanded cytokine-induced killer cells therapy in advanced pancreatic cancer. Cancer Immunol. Immunother. 63, 939–946. doi:10.1007/s00262-014-1566-3
Conde, J., Bao, C., Tan, Y., Cui, D., Edelman, E. R., Azevedo, H. S., et al. (2015). Dual targeted immunotherapy via. Adv. Funct. Mater. 25, 4183–4194. doi:10.1002/adfm.201501283
Conlon, K. C., Miljkovic, M. D., and Waldmann, T. A. (2019). Cytokines in the treatment of cancer. J. Interferon Cytokine Res. 39, 6–21. doi:10.1089/jir.2018.0019
Da Silva, C. A., Chalouni, C., Williams, A., Hartl, D., Lee, C. G., and Elias, J. A. (2009). Chitin is a size-dependent regulator of macrophage TNF and IL-10 production. J. Immunol. 182, 3573–3582. doi:10.4049/jimmunol.0802113
Davis, M. E., Chen, Z., and Shin, D. M. (2008). Nanoparticle therapeutics: an emerging treatment modality for cancer. Nat. Rev. Drug Discov. 7, 771–782. doi:10.1038/nrd2614
Deng, H., and Zhang, Z. (2018). The application of nanotechnology in immune checkpoint blockade for cancer treatment. J. Contr. Release 290, 28–45. doi:10.1016/j.jconrel.2018.09.026
Duan, X., Chan, C., Han, W., Guo, N., Weichselbaum, R. R., and Lin, W. (2019). Immunostimulatory nanomedicines synergize with checkpoint blockade immunotherapy to eradicate colorectal tumors. Nat. Commun. 10, 1899. doi:10.1038/s41467-019-09221-x
Dutta, D., Sundaram, S. K., Teeguarden, J. G., Riley, B. J., Fifield, L. S., Jacobs, J. M., et al. (2007). Adsorbed proteins influence the biological activity and molecular targeting of nanomaterials. Toxicol. Sci. 100, 303–315. doi:10.1093/toxsci/kfm217
Ehlerding, E. B., England, C. G., McNeel, D. G., and Cai, W. (2016). Molecular imaging of immunotherapy targets in cancer. J. Nucl. Med. 57, 1487–1492. doi:10.2967/jnumed.116.177493
Emami, F., Banstola, A., Vatanara, A., Lee, S., Kim, J. O., Jeong, J. H., et al. (2019). Doxorubicin and anti-PD-L1 antibody conjugated gold nanoparticles for colorectal cancer photochemotherapy. Mol. Pharm. 16(3), 1184-1199. doi:10.1021/acs.molpharmaceut.8b01157
Exley, M. A., Friedlander, P., Alatrakchi, N., Vriend, L., Yue, S., Sasada, T., et al. (2017). Adoptive transfer of invariant NKT cells as immunotherapy for advanced melanoma: A phase I clinical trial. Clin. Canc. Res. 23, 3510–3519. doi:10.1158/1078-0432.CCR-16-0600
Fan, W., Yung, B., Huang, P., and Chen, X. (2017). Nanotechnology for multimodal synergistic cancer therapy. Chem. Rev. 117, 13566–13638. doi:10.1021/acs.chemrev.7b00258
Fang, C., and Zhang, M. (2009). Multifunctional magnetic nanoparticles for medical imaging applications. J. Mater. Chem. 19, 6258–6266. doi:10.1039/b902182e
Fang, F., Li, M., Zhang, J., and Lee, C. S. (2020). Different strategies for organic nanoparticle preparation in biomedicine. ACS Materials Lett. 2, 531–549. doi:10.1021/acsmaterialslett.0c00078
Fang, R. H., Kroll, A. V., Gao, W., and Zhang, L. (2018). Cell membrane coating nanotechnology. Adv. Mater. 30, e1706759. doi:10.1002/adma.201706759
Fang, R. H., and Zhang, L. (2016). Nanoparticle-based modulation of the immune system. Annu. Rev. Chem. Biomol. Eng. 7, 305–326. doi:10.1146/annurev-chembioeng-080615-034446
Farhana, A., and Khan, Y. S. (2020). Biochemistry, lipopolysaccharide. Florida: StatPearls Publishing.
Faria, M., Björnmalm, M., Thurecht, K. J., Kent, S. J., Parton, R. G., Kavallaris, M., et al. (2018). Minimum information reporting in bio–nano experimental literature. Nat. Nanotechnol. 13, 777–785. doi:10.1038/s41565-018-0246-4
Ferrari, M. (2010). Frontiers in cancer nanomedicine: directing mass transport through biological barriers. Trends Biotechnol. 28, 181–188. doi:10.1016/j.tibtech.2009.12.007
Ferreira, C. A., Ni, D., Rosenkrans, Z. T., and Cai, W. (2019). Radionuclide‐activated nanomaterials and their biomedical applications. Angew Chem. Int. Ed. Engl. 58, 13232–13252. doi:10.1002/anie.201900594
Fife, B. T., and Bluestone, J. A. (2008). Control of peripheral T-cell tolerance and autoimmunity via the CTLA-4 and PD-1 pathways. Immunol. Rev. 224, 166–182. doi:10.1111/j.1600-065X.2008.00662.x
Foged, C., Brodin, B., Frokjaer, S., and Sundblad, A. (2005). Particle size and surface charge affect particle uptake by human dendritic cells in an in vitro model. Int. J. Pharm. 298, 315–322. doi:10.1016/j.ijpharm.2005.03.035
Gargett, T., Abbas, M. N., Rolan, P., Price, J. D., Gosling, K. M., Ferrante, A., et al. (2018). Phase I trial of Lipovaxin-MM, a novel dendritic cell-targeted liposomal vaccine for malignant melanoma. Cancer Immunol. Immunother. 67, 1461–1472. doi:10.1007/s00262-018-2207-z
Georgakilas, V., Perman, J. A., Tucek, J., and Zboril, R. (2015). Broad family of carbon nanoallotropes: classification, chemistry, and applications of fullerenes, carbon dots, nanotubes, graphene, nanodiamonds, and combined superstructures. Chem. Rev. 115, 4744–4822. doi:10.1021/cr500304f
George, A. P., Kuzel, T. M., Zhang, Y., and Zhang, B. (2019). The discovery of biomarkers in cancer immunotherapy. Comput. Struct. Biotechnol. J. 17, 484–497. doi:10.1016/j.csbj.2019.03.015
Ghomi, E. R., Khosravi, F., Neisiany, R. E., Singh, S., and Ramakrishna, S. (2020). Future of additive manufacturing in healthcare. Curr. Opin. Biomed. Eng. 17, 100255. 10.1016/j.cobme.2020.100255
Goldberg, M. S. (2015). Immunoengineering: how nanotechnology can enhance cancer immunotherapy. Cell 161, 201–204. doi:10.1016/j.cell.2015.03.037
Gonzalez, H., Hagerling, C., and Werb, Z. (2018). Roles of the immune system in cancer: from tumor initiation to metastatic progression. Genes Dev. 32, 1267–1284. doi:10.1101/GAD.314617.118
Goodman, C. M., McCusker, C. D., Yilmaz, T., and Rotello, V. M. (2004). Toxicity of gold nanoparticles functionalized with cationic and anionic side chains. Bioconjugate Chem. 15, 897–900. doi:10.1021/bc049951i
Gorbet, M. J., and Ranjan, A. (2020). Cancer immunotherapy with immunoadjuvants, nanoparticles, and checkpoint inhibitors: recent progress and challenges in treatment and tracking response to immunotherapy. Pharmacol. Ther. 207, 107456. doi:10.1016/j.pharmthera.2019.107456
Grillo, R., Gallo, J., Stroppa, D. G., Carbó-Argibay, E., Lima, R., Fraceto, L. F., et al. (2016). Sub-micrometer magnetic nanocomposites: insights into the effect of magnetic nanoparticles interactions on the optimization of SAR and MRI performance. ACS Appl. Mater. Interfaces 8, 25777–25787. doi:10.1021/acsami.6b08663
Guan, X., Chen, J., Hu, Y., Lin, L., Sun, P., Tian, H., et al. (2018). Highly enhanced cancer immunotherapy by combining nanovaccine with hyaluronidase. Biomaterials 171, 198–206. doi:10.1016/j.biomaterials.2018.04.039
Guo, L., Yan, D. D., Yang, D., Li, Y., Wang, X., Zalewski, O., et al. (2014). Combinatorial photothermal and immuno cancer therapy using chitosan-coated hollow copper sulfide nanoparticles. ACS Nano 8, 5670–5681. doi:10.1021/nn5002112
Hamid, O., Schmidt, H., Nissan, A., Ridolfi, L., Aamdal, S., Hansson, J., et al. (2011). A prospective phase II trial exploring the association between tumor microenvironment biomarkers and clinical activity of ipilimumab in advanced melanoma. J. Transl. Med. 9, 204. doi:10.1186/1479-5876-9-204
Han, Y., Yang, Y., Chen, Z., Jiang, Z., Gu, Y., Liu, Y., et al. (2014). Human hepatocellular carcinoma-infiltrating CD4+CD69 +Foxp3- regulatory T cell suppresses T cell response via membrane-bound TGF-β1. J. Mol. Med. (Berl.) 92, 539–550. doi:10.1007/s00109-014-1143-4
Harisi, R., and Jeney, A. (2015). Extracellular matrix as target for antitumor therapy. OncoTargets Ther. 8, 1387–1398. doi:10.2147/OTT.S48883
Hauge, A., and Rofstad, E. K. (2020). Antifibrotic therapy to normalize the tumor microenvironment. J. Transl. Med. 18, 1–11. doi:10.1186/s12967-020-02376-y
Henke, E., Nandigama, R., and Ergün, S. (2020). Extracellular matrix in the tumor microenvironment and its impact on cancer therapy. Front. Mol. Biosci. 6, 160. doi:10.3389/fmolb.2019.00160
Hennrich, U., and Kopka, K. (2019). Lutathera®: the first FDA-and EMA-approved radiopharmaceutical for peptide receptor radionuclide therapy. Pharmaceuticals 12, 114. doi:10.3390/ph12030114
Hirano, F., Kaneko, K., Tamura, H., Dong, H., Wang, S., Ichikawa, M., et al. (2005). Blockade of B7-H1 and PD-1 by monoclonal antibodies potentiates cancer therapeutic immunity. Canc. Res. 65, 1089–1097. https://pubmed.ncbi.nlm.nih.gov/15705911/
Hossen, S., Hossain, M. K., Basher, M. K., Mia, M. N. H., Rahman, M. T., and Uddin, M. J. (2019). Smart nanocarrier-based drug delivery systems for cancer therapy and toxicity studies: a review. J. Adv. Res. 15, 1–18. doi:10.1016/j.jare.2018.06.005
Hu, Z., Ott, P. A., and Wu, C. J. (2018). Towards personalized, tumour-specific, therapeutic vaccines for cancer. Nat. Rev. Immunol. 18, 168–182. doi:10.1038/nri.2017.131
Huang, H. C., Pigula, M., Fang, Y., and Hasan, T. (2018). Immobilization of photo-immunoconjugates on nanoparticles leads to enhanced light-activated biological effects. Small 14, e1800236. doi:10.1002/smll.201800236
Huang, Y., Mei, C., Tian, Y., Nie, T., Liu, Z., and Chen, T. (2018). Bioinspired tumor-homing nanosystem for precise cancer therapy via reprogramming of tumor-associated macrophages. NPG Asia Mater. 10, 1002–1015. doi:10.1038/s41427-018-0091-9
Huang, X., Jain, P. K., El-Sayed, I. H., and El-Sayed, M. A. (2007). Gold nanoparticles: interesting optical properties and recent applications in cancer diagnostics and therapy. Nanomedicine 2, 681–693. doi:10.2217/17435889.2.5.681
Huynh, E., Leung, B. Y. C., Helfield, B. L., Shakiba, M., Gandier, J. A., Jin, C. S., et al. (2015). In situ conversion of porphyrin microbubbles to nanoparticles for multimodality imaging. Nat. Nanotechnol. 10, 325–332. doi:10.1038/nnano.2015.25
Jain, V., Jain, S., and Mahajan, S. C. (2015). Nanomedicines based drug delivery systems for anti-cancer targeting and treatment. Curr. Drug Deliv. 12, 177–191. doi:10.2174/1567201811666140822112516
Jiang, W., Von Roemeling, C. A., Chen, Y., Qie, Y., Liu, X., Chen, J., et al. (2017). Designing nanomedicine for immuno-oncology. Nat. Biomed. Eng. 1, 29. doi:10.1038/s41551-017-0029
Jie, H. B., Gildener-Leapman, N., Li, J., Srivastava, R. M., Gibson, S. P., Whiteside, T. L., et al. (2013). Intratumoral regulatory T cells upregulate immunosuppressive molecules in head and neck cancer patients. Br. J. Canc. 109, 2629–2635. doi:10.1038/bjc.2013.645
Jin, Y., Ma, X., Feng, S., Liang, X., Dai, Z., Tian, J., et al. (2015). Hyaluronic acid modified tantalum oxide nanoparticles conjugating doxorubicin for targeted cancer theranostics. Bioconjugate Chem. 26, 2530–2541. doi:10.1021/acs.bioconjchem.5b00551
Kakita, N., Kanto, T., Itose, I., Kuroda, S., Inoue, M., Matsubara, T., et al. (2012). Comparative analyses of regulatory T cell subsets in patients with hepatocellular carcinoma: a crucial role of CD25-FOXP3- T cells. Int. J. Canc. 131, 2573–2583. doi:10.1002/ijc.27535
Kalergis, A. H., Boucheron, N., Doucey, M. A., Palmieri, E., Goyarts, E. C., Vegh, Z., et al. (2001). Efficient T cell activation requires an optimal dwell-time of interaction between the TCR and the pMHC complex. Nat. Immunol. 2, 229–234. doi:10.1038/85286
Kanapathipillai, M., Mammoto, A., Mammoto, T., Kang, J. H., Jiang, E., Ghosh, K., et al. (2012). Inhibition of mammary tumor growth using lysyl oxidase-targeting nanoparticles to modify extracellular matrix. Nano Lett. 12, 3213–3217. doi:10.1021/nl301206p
Kang, T., Zhu, Q., Wei, D., Feng, J., Yao, J., Jiang, T., et al. (2017). Nanoparticles coated with neutrophil membranes can effectively treat cancer metastasis. ACS Nano 11, 1397–1411. doi:10.1021/acsnano.6b06477
Kasten, B. B., Udayakumar, N., Leavenworth, J. W., Wu, A. M., Lapi, S. E., McConathy, J. E., et al. (2019). Current and future imaging methods for evaluating response to immunotherapy in neuro-oncology. Theranostics 9, 5085–5104. doi:10.7150/thno.34415
Kaushik, A., and Nair, M. (2018). Nanotheranostic, next generation prerequisite for better health. J. Nanotheranostics. 1, 1–5. doi:10.3390/jnt1010001
Keir, M. E., Butte, M. J., Freeman, G. J., and Sharpe, A. H. (2008). PD-1 and its ligands in tolerance and immunity. Annu. Rev. Immunol. 26, 677–704. doi:10.1146/annurev.immunol.26.021607.090331
Khawar, I. A., Kim, J. H., and Kuh, H. (2014). Improving drug delivery to solid tumors: priming the tumor microenvironment. J. Contr. Release 201, 78–89. doi:10.1016/j.jconrel.2014.12.018
Kienzle, A., Kurch, S., Schlöder, J., Berges, C., Ose, R., Schupp, J., et al. (2017). Dendritic mesoporous silica nanoparticles for pH-stimuli- responsive drug delivery of TNF-alpha. Adv. Healthc. Mater. 6, 1700012. doi:10.1002/adhm.201700012
Kievit, F. M., and Zhang, M. (2011). Cancer nanotheranostics: improving imaging and therapy by targeted delivery across biological barriers. Adv. Mater. 23, H217–H247. doi:10.1002/adma.201102313
Kim, S., Kang, S. H., Byun, S. H., Kim, H. J., Park, I. K., Hirschberg, H., et al. (2020). Intercellular bioimaging and biodistribution of gold nanoparticle-loaded macrophages for targeted drug delivery. Electronics (Switzerland) 9, 1105. doi:10.3390/electronics9071105
Klemm, F., and Joyce, J. A. (2014). Microenvironmental regulation of therapeutic response in cancer. Trends Cell Biol. 25, 198–213. doi:10.1016/j.tcb.2014.11.006
Kodali, V., Littke, M. H., Tilton, S. C., Teeguarden, J. G., Shi, L., Frevert, C. W., et al. (2013). Dysregulation of macrophage activation profiles by engineered nanoparticles. ACS Nano 7, 6997–7010. doi:10.1021/nn402145t
Kondratiev, S., Gnepp, D. R., Yakirevich, E., Sabo, E., Annino, D. J., Rebeiz, E., et al. (2008). Expression and prognostic role of MMP2, MMP9, MMP13, and MMP14 matrix metalloproteinases in sinonasal and oral malignant melanomas. Hum. Pathol. 39, 337–343. doi:10.1016/j.humpath.2007.07.003
Kranz, L. M., Diken, M., Haas, H., Kreiter, S., Loquai, C., Reuter, K. C., et al. (2016). Systemic RNA delivery to dendritic cells exploits antiviral defence for cancer immunotherapy. Nature 534, 396–401. doi:10.1038/nature18300
Kurose, K., Ohue, Y., Sato, E., Yamauchi, A., Eikawa, S., Isobe, M., et al. (2015). Increase in activated Treg in TIL in lung cancer and in vitro depletion of Treg by ADCC using an antihuman CCR4 mAb (KM2760). J. Thorac. Oncol. 10, 74–83. doi:10.1097/JTO.0000000000000364
Kutova, O. M., Guryev, E. L., Sokolova, E. A., Alzeibak, R., and Balalaeva, I. V. (2019). Targeted delivery to tumors: multidirectional strategies to improve treatment efficiency. Cancers 11, 1–33. doi:10.3390/cancers11010068
Lane, L. A., Qian, X., Smith, A. M., and Nie, S. (2015). Physical chemistry of nanomedicine: understanding the complex behaviors of nanoparticles in vivo. Annu. Rev. Phys. Chem. 66, 521–547. doi:10.1146/annurev-physchem-040513-103718
Le, Q. V., Choi, J., and Oh, Y. K. (2018). Nano delivery systems and cancer immunotherapy. J. Pharm. Investig. 48, 527–539. doi:10.1007/s40005-018-0399-z
Le, Q. V., Yang, G., Wu, Y., Jang, H. W., Shokouhimehr, M., and Oh, Y. K. (2019). Nanomaterials for modulating innate immune cells in cancer immunotherapy. Asian J. Pharm. Sci. 14, 16–29. doi:10.1016/j.ajps.2018.07.003
Leach, D. R., Krummel, M. F., and Allison, J. P. (1996). Enhancement of antitumor immunity by CTLA-4 blockade. Science 271, 1734–1736. doi:10.1126/science.271.5256.1734
Lee, K. W., Jeong, D., and Na, K. (2013). Doxorubicin loading fucoidan acetate nanoparticles for immune and chemotherapy in cancer treatment. Carbohydr. Polym. 94, 850–856. doi:10.1016/j.carbpol.2013.02.018
Lee, S. B., Lee, J. E., Cho, S. J., Chin, J., Kim, S. K., Lee, I. K., et al. (2019). Crushed gold shell nanoparticles labeled with radioactive iodine as a theranostic nanoplatform for macrophage-mediated photothermal therapy. Nano-Micro Lett. 11, 36. doi:10.1007/s40820-019-0266-0
Lee, S., and Margolin, K. (2011). Cytokines in cancer immunotherapy. Cancers 3, 3856–3893. doi:10.3390/cancers3043856
Leo, C. P., Hentschel, B., Szucs, T. D., and Leo, C. (2020). FDA and EMA approvals of new breast cancer drugs—a comparative regulatory analysis. Cancers 12, 437. doi:10.3390/cancers12020437
Li, C. X., Zhang, Y., Dong, X., Zhang, L., Liu, M. D., Li, B., et al. (2019). Artificially reprogrammed macrophages as tumor-tropic immunosuppression-resistant biologics to realize therapeutics production and immune activation. Adv. Math. 31, e1807211. doi:10.1002/adma.201807211
Li, M., Li, S., Zhou, H., Tang, X., Wu, Y., Jiang, W., et al. (2020). Chemotaxis-driven delivery of nano-pathogenoids for complete eradication of tumors post- phototherapy. Nat. Commun. 11, 1126. doi:10.1038/s41467-020-14963-0
Li, P., Zhou, J., Huang, P., Zhang, C., Wang, W., Li, C., et al. (2017). Self-assembled PEG-b-PDPA-b-PGEM copolymer nanoparticles as protein antigen delivery vehicles to dendritic cells: preparation, characterization and cellular uptake. Regen. Biomater. 4, 11–20. doi:10.1093/rb/rbw044
Li, X., Zhu, L., Wang, B., Yuan, M., and Zhu, R. (2017). Drugs and targets in fibrosis. Front. Pharmacol. 8, 855. doi:10.3389/fphar.2017.00855
Li, R., He, Y., Zhang, S., Qin, J., and Wang, J. (2018). Cell membrane-based nanoparticles: a new biomimetic platform for tumor diagnosis and treatment. Acta Pharm. Sin. B. 8, 14–22. doi:10.1016/j.apsb.2017.11.009
Li, Y., Du, Y., Liang, X., Sun, T., Xue, H., Tian, J., et al. (2018). EGFR-targeted liposomal nanohybrid cerasomes: theranostic function and immune checkpoint inhibition in a mouse model of colorectal cancer. Nanoscale 10, 16738–16749. doi:10.1039/c8nr05803b
Li, S., Liu, Y., Xu, C., Shen, S., Sun, R., Du, X., et al. (2016). Restoring anti-tumor functions of T cells via nanoparticle-mediated immune checkpoint modulation. J. Contr. Release 231, 17–28. doi:10.1016/j.jconrel.2016.01.044
Liang, B., Deng, T., Li, J., Ouyang, X., Na, W., and Deng, D. (2020). Biomimetic theranostic strategy for anti-metastasis therapy of breast cancer via the macrophage membrane camouflaged superparticles. Mater. Sci. Eng. C. Mater. Biol. Appl. 115, 111097. doi:10.1016/j.msec.2020.111097
Liang, P., Liu, . J., Zhuo, R. X., and Cheng, S. X. (2013). Self-assembled inorganic/organic hybrid nanoparticles with multi-functionalized surfaces for active targeting drug delivery. J. Mater. Chem. B. 1, 4243–4250. doi:10.1039/c3tb20455c
Lin, Y. C., Mahalingam, J., Chiang, J. M., Su, P. J., Chu, Y. Y., Lai, H. Y., et al. (2013). Activated but not resting regulatory T cells accumulated in tumor microenvironment and correlated with tumor progression in patients with colorectal cancer. Int. J. Canc. 132, 1341–1350. doi:10.1002/ijc.27784
Ling, D., Park, W., Park, S. J., Lu, Y., Kim, K. S., Hackett, M. J., et al. (2014). Multifunctional tumor pH-sensitive self-assembled nanoparticles for bimodal imaging and treatment of resistant heterogeneous tumors. J. Am. Chem. Soc. 136, 5647–5655. doi:10.1021/ja4108287
Linkov, I., Satterstrom, F. K., and Corey, L. M. (2008). Nanotoxicology and nanomedicine: making hard decisions. Nanomedicine: NBM (NMR Biomed.) 4, 167–171. doi:10.1016/j.nano.2008.01.001
Liu, Q., Jia, J., Yang, T., Fan, Q., Wang, L., and Ma, G. (2016). Pathogen-mimicking polymeric nanoparticles based on dopamine polymerization as vaccines adjuvants induce robust humoral and cellular immune responses. Small 12, 1744–1757. doi:10.1002/smll.201503662
Liu, T., Zhou, L., Li, D., Andl, T., and Zhang, Y. (2019). Cancer-associated fibroblasts build and secure the tumor microenvironment. Front. Cell Dev. Biol. 7, 60. doi:10.3389/fcell.2019.00060
Liu, X., Zheng, J., Sun, W., Zhao, X., Li, Y., Gong, N., et al. (2019). Ferrimagnetic vortex nanoring-mediated mild magnetic hyperthermia imparts potent immunological effect for treating cancer metastasis. ACS Nano 13, 8811–8825. doi:10.1021/acsnano.9b01979
Liu, Y., Luo, J., Chen, X., Liu, W., and Chen, T. (2019). Cell membrane coating technology: a promising strategy for biomedical applications. Nano-Micro Lett. 11, 100. doi:10.1007/s40820-019-0330-9
Liu, Y., Wang, X., Hussain, M., Lv, M., Dong, X., Wang, T., et al. (2018). Theranostics applications of nanoparticles in cancer immunotherapy. Med. Sci. 6, 100. doi:10.3390/medsci6040100
Longmire, M., Choyke, P. L., and Kobayashi, H. (2008). Clearance properties of nano-sized particles and molecules as imaging agents: considerations and caveats. Nanomedicine 3, 703–717. doi:10.2217/17435889.3.5.703
Lu, K., He, C., Guo, N., Chan, C., Ni, K., Weichselbaum, R. R., et al. (2016). Chlorin-based nanoscale metal-organic framework systemically rejects colorectal cancers via synergistic photodynamic therapy and checkpoint blockade immunotherapy. J. Am. Chem. Soc. 138, 12502–12510. doi:10.1021/jacs.6b06663
Lu, Y., Miao, L., Wang, Y., Xu, Z., Zhao, Y., Shen, Y., et al. (2016). Curcumin micelles remodel tumor microenvironment and enhance vaccine activity in an advanced melanoma model. Mol. Ther. 24, 364–374. doi:10.1038/mt.2015.165
Luo, M., Wang, H., Wang, Z., Cai, H., Lu, Z., Li, Y., et al. (2017). A STING-activating nanovaccine for cancer immunotherapy. Nat. Nanotechnol. 12, 648–654. doi:10.1038/nnano.2017.52
Madamsetty, V. S., Mukherjee, A., and Mukherjee, S. (2019). Recent trends of the bio-inspired nanoparticles in cancer theranostics. Front. Pharmacol. 10, 1–12. doi:10.3389/fphar.2019.01264
Maeda, S., Wada, H., Naito, Y., Nagano, H., Simmons, S., Kagawa, Y., et al. (2014). Interferon-α acts on the S/G2/M phases to induce apoptosis in the G1 phase of an IFNAR2-expressing hepatocellular carcinoma cell line. J. Biol. Chem. 289, 23786–23795. doi:10.1074/jbc.M114.551879
Maksoudian, C., Saffarzadeh, N., Hesemans, E., Dekoning, N., Buttiens, K., and Soenen, S. J. (2020). Role of inorganic nanoparticle degradation in cancer therapy. Nanoscale Adv. 2, 3734–3763. doi:10.1039/d0na00286k
Manivasagan, P., Bharathiraja, S., Bui, N. Q., Jang, B., Oh, Y. O., Lim, I. G., et al. (2016). Doxorubicin-loaded fucoidan capped gold nanoparticles for drug delivery and photoacoustic imaging. Int. J. Biol. Macromol. 91, 578–588. doi:10.1016/j.ijbiomac.2016.06.007
Manolova, V., Flace, A., Bauer, M., Schwarz, K., Saudan, P., and Bachmann, M. F. (2008). Nanoparticles target distinct dendritic cell populations according to their size. Eur. J. Immunol. 38, 1404–1413. doi:10.1002/eji.200737984
Marrache, S., Tundup, S., Harn, D. A., and Dhar, S. (2013). Ex vivo programming of dendritic cells by mitochondria-targeted nanoparticles to produce interferon-gamma for cancer immunotherapy. ACS Nano 7, 7392–7402. doi:10.1021/nn403158n
Mashinchian, O., Johari-Ahar, M., Ghaemi, B., Rashidi, M., Barar, J., and Omidi, Y. (2014). Impacts of quantum dots in molecular detection and bioimaging of cancer. Bioimpacts 4, 149–166. doi:10.15171/bi.2014.008
Mcmichael, E. L., Benner, B., Atwal, L. S., Courtney, N. B., Mo, X., Davis, M. E., et al. (2019). A phase I/II trial of cetuximab in combination with interleukin-12 administered to patients with unresectable primary or recurrent head and neck squamous cell carcinoma. Clin. Canc. Res. 25, 4955–4966. doi:10.1158/1078-0432.CCR-18-2108
Medina-Reyes, E. I., García-Viacobo, D., Carrero-Martínez, F. A., and Chirino, Y. I. (2017). Applications and risks of nanomaterials used in regenerative medicine, delivery systems, theranostics, and therapy. Crit. Rev. Ther. Drug Carrier Syst. 34, 35–62. doi:10.1615/CritRevTherDrugCarrierSyst.2017016983
Meir, R., Shamalov, K., Betzer, O., Motiei, M., Horovitz-, M., Yehuda, R., et al. (2015). Nanomedicine for cancer immunotherapy : tracking cancer- specific T-cells in vivo with gold nanoparticles and CT imaging. ACS Nano 9, 6363–6372. doi:10.1021/acsnano.5b01939
Melero, I., Berman, D. M., Aznar, M. A., Korman, A. J., Luis, J., Gracia, P., et al. (2015). Evolving synergistic combinations of targeted immunotherapies to combat cancer. Nat. Rev. Canc. 15, 457–472. doi:10.1038/nrc3973
Mendes, L. P., Lima, E. M., and Torchilin, V. P. (2018). “Targeted nanotheranostics for selective drug delivery in cancer,” in The handbook of nanomaterials for cancer theranostics. Editor J. Conde (Amsterdam, Netherlands: Elsevier), 245–277.
Mi, P. (2020). Stimuli-responsive nanocarriers for drug delivery, tumor imaging, therapy and theranostics. Theranostics 10, 4557–4588. doi:10.7150/thno.38069
Michallet, M., Maloisel, F., Delain, M., Hellmann, A., Rosas, A., Silver, R. T., et al. (2004). Pegylated recombinant interferon alpha-2b vs recombinant interferon alpha-2b for the initial treatment of chronic-phase chronic myelogenous leukemia: a phase III study. Leukemia 18, 309–315. doi:10.1038/sj.leu.2403217
Miller, K. D., Fidler‐Benaoudia, M., Keegan, T. H., Hipp, H. S., Jemal, A., and Siegel, R. L. (2020). Cancer statistics for adolescents and young adults. CA Cancer J. Clin. 70, 443–459. doi:10.3322/caac.21637
Miller, M. A., Zheng, Y., Gadde, S., Pfirschke, C., Zope, H., Engblom, C., et al. (2015). Tumour-associated macrophages act as a slow-release reservoir of nano-therapeutic Pt(IV) pro-drug. Nat. Commun. 6, 8692. doi:10.1038/ncomms9692
Mittler, R. S., Foell, J., Mccausland, M., Strahotin, S., Niu, L., Bapat, A., et al. (2004). Anti-CD137 antibodies in the treatment of autoimmune disease and cancer. Immunol. Res. 29, 197–208. doi:10.1385/IR:29:1-3:197
Nam, J., Son, S., Ochyl, L. J., Kuai, R., Schwendeman, A., and Moon, J. J. (2018). Chemo-photothermal therapy combination elicits anti-tumor immunity against advanced metastatic cancer. Nat. Commun. 9, 1074. doi:10.1038/s41467-018-03473-9
Nam, J., Son, S., Park, K. S., Zou, W., Shea, L. D., and Moon, J. J. (2019). Cancer nanomedicine for combination cancer immunotherapy. Nat. Rev. Mater. 4, 398–414. doi:10.1038/s41578-019-0108-1
Nguyen, H. T., Tran, K. K., Sun, B., and Shen, H. (2012). Activation of inflammasomes by tumor cell death mediated by gold nanoshells. Biomaterials 33, 2197–2205. doi:10.1016/j.biomaterials.2011.11.063
Norman Coleman, C. (1993). Beneficial liaisons: radiobiology meets cellular and molecular biology. Radiother. Oncol. 28, 1–15. doi:10.1016/0167-8140(93)90179-C
Obst, K., Yealland, G., Balzus, B., Miceli, E., Dimde, M., Weise, C., et al. (2017). Protein corona formation on colloidal polymeric nanoparticles and polymeric nanogels: impact on cellular uptake, toxicity, immunogenicity, and drug release properties. Biomacromolecules 18, 1762–1771. doi:10.1021/acs.biomac.7b00158
Ou, W., Jiang, L., Thapa, R. K., Soe, Z. C., Poudel, K., Chang, J. H., et al. (2018). Combination of NIR therapy and regulatory T cell modulation using layer-by-layer hybrid nanoparticles for effective cancer photoimmunotherapy. Theranostics 8, 4574–4590. doi:10.7150/thno.26758
Overchuk, M., and Zheng, G. (2018). Overcoming obstacles in the tumor microenvironment: recent advancements in nanoparticle delivery for cancer theranostics. Biomaterials 156, 217–237. doi:10.1016/j.biomaterials.2017.10.024
Pachella, L. A., Madsen, L. T., and Dains, J. E. (2015). The toxicity and benefit of various dosing strategies for interleukin-2 in metastatic melanoma and renal cell carcinoma. J. Adv. Pract. Oncol. 6, 212–221. doi:10.6004/jadpro.2015.6.3.3
Page-McCaw, A., Ewald, A. J., and Werb, Z. (2007). Matrix metalloproteinases and the regulation of tissue remodelling. Nat. Rev. Mol. Cell Biol. 8, 221–233. doi:10.1038/nrm2125
Park, J., Wrzesinski, S. H., Stern, E., Look, M., Criscione, J., Ragheb, R., et al. (2012). Combination delivery of TGF-β inhibitor and IL-2 by nanoscale liposomal polymeric gels enhances tumour immunotherapy. Nat. Mater. 11, 895–905. doi:10.1038/nmat3355
Park, W., Heo, Y.-J., and Han, D. K. (2018). New opportunities for nanoparticles in cancer immunotherapy. Biomater. Res. 22, 24. doi:10.1186/s40824-018-0133-y
Pedroza-Gonzalez, A., Verhoef, C., Ijzermans, J. N. M., Peppelenbosch, M. P., Kwekkeboom, J., Verheij, J., et al. (2013). Activated tumor-infiltrating CD4+ regulatory T cells restrain antitumor immunity in patients with primary or metastatic liver cancer. Hepatology 57, 183–194. doi:10.1002/hep.26013
Peer, D., Karp, J. M., Hong, S., Farokhzad, O. C., Margalit, R., and Langer, R. (2007). Nanocarriers as an emerging platform for cancer therapy. Nat. Nanotechnol. 2, 751–760. doi:10.1038/nnano.2007.387
Perillo, B., Donato, M. D., Pezone, A., Zazzo, E. G., and Migliaccio, A. (2020). ROS in cancer therapy : the bright side of the moon. Exp. Mol. Med. 52, 192–203. doi:10.1038/s12276-020-0384-2
Ridge, J. P., Di Rosa, F., and Matzinger, P. (1998). A conditioned dendritic cell can be a temporal bridge between a CD4 + T-helper and a T-killer cell. Nature 393, 474–478. doi:10.1038/30989
Roma-Rodrigues, C., Pombo, I., Raposo, L., Pedrosa, P., Fernandes, A. R., and Baptista, P. V. (2019). Nanotheranostics targeting the tumor microenvironment. Front. Bioeng. Biotechnol. 7, 197. doi:10.3389/fbioe.2019.00197
Rosenberg, S. A., and Restifo, N. P. (2015). Adoptive cell transfer as personalized immunotherapy for human cancer. Science 348, 62–68. doi:10.1126/science.aaa4967
Roses, R. E., and Czerniecki, B. J. (2014). Radiation as Immunomodulator : implications for dendritic cell-based immunotherapy. Radiat. Res. 182, 211–218. doi:10.1667/RR13495.1
Sacchetti, C., Rapini, N., Magrini, A., Cirelli, E., Bellucci, S., Mattei, M., et al. (2013). In vivo targeting of intratumor regulatory T cells using PEG-modified single-walled carbon nanotubes. Bioconjugate Chem. 24, 852–858. doi:10.1021/bc400070q
Salvati, A., Pitek, A. S., Monopoli, M. P., Prapainop, K., Bombelli, F. B., Hristov, D. R., et al. (2013). Transferrin-functionalized nanoparticles lose their targeting capabilities when a biomolecule corona adsorbs on the surface. Nat. Nanotechnol. 8, 137–143. doi:10.1038/nnano.2012.237
Sano, K., Nakajima, T., Choyke, P. L., and Kobayashi, H. (2014). The effect of photoimmunotherapy followed by liposomal daunorubicin in a mixed tumor model: a demonstration of the super-enhanced permeability and retention effect after photoimmunotherapy. Mol. Canc. Therapeut. 13, 426–432. doi:10.1158/1535-7163.MCT-13-0633
Sasso, M. S., Lollo, G., Pitorre, M., Solito, S., Pinton, L., Valpione, S., et al. (2016). Low dose gemcitabine-loaded lipid nanocapsules target monocytic myeloid-derived suppressor cells and potentiate cancer immunotherapy. Biomaterials 96, 47–62. doi:10.1016/j.biomaterials.2016.04.010
Schuler, P. J., Schilling, B., Harasymczuk, M., Hoffmann, T. K., Johnson, J., Lang, S., et al. (2012). Phenotypic and functional characteristics of CD4+CD39+ FOXP3+ and CD4+CD39+FOXP3neg T-cell subsets in cancer patients. Eur. J. Immunol. 42, 1876–1885. doi:10.1002/eji.201142347
Scurr, M., Ladell, K., Besneux, M., Christian, A., Hockey, T., Smart, K., et al. (2014). Highly prevalent colorectal cancer-infiltrating LAP + Foxp3 - T cells exhibit more potent immunosuppressive activity than Foxp3 + regulatory T cells. Mucosal Immunol. 7, 428–439. doi:10.1038/mi.2013.62
Sengupta, S., Eavarone, D., Capila, I., Zhao, G., Watson, N., Kiziltepe, T., et al. (2005). Temporal targeting of tumour cells and neovasculature with a nanoscale delivery system. Nature 436, 568–572. doi:10.1038/nature03794
Shahbazi, M. A., Shrestha, N., Mäkilä, E., Araújo, F., Correia, A., Ramos, T., et al. (2015). A prospective cancer chemo-immunotherapy approach mediated by synergistic CD326 targeted porous silicon nanovectors. Nano Res. 8, 1505–1521. doi:10.1007/s12274-014-0635-4
Shargh, V. H., Hondermarck, H., and Liang, M. (2016). Antibody-targeted biodegradable nanoparticles for cancer therapy. Nanomedicine 11, 63–79. doi:10.2217/nnm.15.186
Shetab Boushehri, M. A., and Lamprecht, A. (2019). Challenges of using lipopolysaccharides for cancer immunotherapy and potential delivery-based solutions thereto. Ther. Deliv. 10, 165–187. doi:10.4155/tde-2018-0076
Shi, H., Ye, X., He, X., Wang, K., Cui, W., He, D., et al. (2014). Au@Ag/Au nanoparticles assembled with activatable aptamer probes as smart “nano-doctors” for image-guided cancer thermotherapy. Nanoscale 6, 8754–8761. doi:10.1039/c4nr01927j
Shima, F., Akagi, T., Uto, T., and Akashi, M. (2013). Manipulating the antigen-specific immune response by the hydrophobicity of amphiphilic poly(γ-glutamic acid) nanoparticles. Biomaterials 34, 9709–9716. doi:10.1016/j.biomaterials.2013.08.064
Shin, W. K., Cho, J., Kannan, A. G., Lee, Y. S., and Kim, D. W. (2016). Cross-linked composite gel polymer electrolyte using mesoporous methacrylate-functionalized SiO2 nanoparticles for lithium-ion polymer batteries. Sci. Rep. 6, 26332. doi:10.1038/srep26332
Shvedova, A. A., Tkach, A. V., Kisin, E. R., Khaliullin, T., Stanley, S., Gutkin, D. W., et al. (2013). Carbon nanotubes enhance metastatic growth of lung carcinoma via up-regulation of myeloid-derived suppressor cells. Small 9, 1691–1695. doi:10.1002/smll.201201470
Siegel, R. L., Miller, K. D., and Jemal, A. (2020). Cancer statistics. CA Cancer J. Clin. 70, 7–30. doi:10.3322/caac.21590
Siegler, E. L., Kim, Y. J., and Wang, P. (2016). Nanomedicine targeting the tumor microenvironment: therapeutic strategies to inhibit angiogenesis, remodel matrix, and modulate immune responses. J. Cell. Immunother. 2, 69–78. doi:10.1016/j.jocit.2016.08.002
Singh, A., Nandwana, V., Rink, J. S., Ryoo, S. R., Chen, T. H., Allen, S. D., et al. (2019). Biomimetic magnetic nanostructures: a theranostic platform targeting lipid metabolism and immune response in lymphoma. ACS Nano 13, 10301–10311. doi:10.1021/acsnano.9b03727
Soleymani, M., Velashjerdi, M., Shaterabadi, Z., and Barati, A. (2020). One-pot preparation of hyaluronic acid‐coated iron oxide nanoparticles for magnetic hyperthermia therapy and targeting CD44-overexpressing cancer cells. Carbohydr. Polym. 237, 116130. doi:10.1016/j.carbpol.2020.116130
Song, W., Musetti, S. N., and Huang, L. (2017). Nanomaterials for cancer immunotherapy. Biomaterials 148, 16–30. doi:10.1016/j.biomaterials.2017.09.017
Song, W., Shen, L., Wang, Y., Liu, Q., Goodwin, T. J., Li, J., et al. (2018). Synergistic and low adverse effect cancer immunotherapy by immunogenic chemotherapy and locally expressed PD-L1 trap. Nat. Commun. 9, 2237. doi:10.1038/s41467-018-04605-x
Sugiyama, D., Nishikawa, H., Maeda, Y., Nishioka, M., Tanemura, A., Katayama, I., et al. (2013). Anti-CCR4 mAb selectively depletes effector-Type FoxP3+CD4+ regulatory T cells, evoking antitumor immune responses in humans. Proc. Natl. Acad. Sci. U. S. A. 110, 17945–17950. doi:10.1073/pnas.1316796110
Sukumar, U. K., Bose, R. J. C., Malhotra, M., Babikir, H. A., Afjei, R., Robinson, E., et al. (2019). Intranasal delivery of targeted polyfunctional gold–iron oxide nanoparticles loaded with therapeutic microRNAs for combined theranostic multimodality imaging and presensitization of glioblastoma to temozolomide. Biomaterials 218, 119342. doi:10.1016/j.biomaterials.2019.119342
Sun, J. C., Beilke, J. N., and Lanier, L. L. (2009). Adaptive immune features of natural killer cells. Nature 457, 557–561. doi:10.1038/nature07665
Tan, S., Wu, T., Zhang, D., and Zhang, Z. (2015). Cell or cell membrane-based drug delivery systems. Theranostics 5, 863–881. doi:10.7150/thno.11852
Tang, J., Yu, J. X., Hubbard-Lucey, V. M., Neftelinov, S. T., Hodge, J. P., and Lin, Y. (2018). The clinical trial landscape for PD1/PDl1 immune checkpoint inhibitors. Nat. Rev. Drug Discov. 17, 854–855. doi:10.1038/nrd.2018.210
Tao, Y., Ju, E., Liu, Z., Dong, K., Ren, J., and Qu, X. (2014a). Engineered, self-assembled near-infrared photothermal agents for combined tumor immunotherapy and chemo-photothermal therapy. Biomaterials 35, 6646–6656. doi:10.1016/j.biomaterials.2014.04.073
Tao, Y., Ju, E., Ren, J., and Qu, X. (2014b). Immunostimulatory oligonucleotides-loaded cationic graphene oxide with photothermally enhanced immunogenicity for photothermal/immune cancer therapy. Biomaterials 35, 9963–9971. doi:10.1016/j.biomaterials.2014.08.036
Thorat, N. D., Townely, H., Brennan, G., Parchur, A. K., Silien, C., Bauer, J., et al. (2019). Progress in remotely triggered hybrid nanostructures for next-generation brain cancer theranostics. ACS Biomater. Sci. Eng. 5, 2669–2687. doi:10.1021/acsbiomaterials.8b01173
Tian, L., Yi, X., Dong, Z., Xu, J., Liang, C., Chao, Y., et al. (2018). Calcium bisphosphonate nanoparticles with chelator-free radiolabeling to deplete tumor-associated macrophages for enhanced cancer radioisotope therapy. ACS Nano 12, 11541–11551. doi:10.1021/acsnano.8b06699
Tran, T. H., Tran, T. T. P., Nguyen, H. T., Phung, C. D., Jeong, J. H., Stenzel, M. H., et al. (2018). Nanoparticles for dendritic cell-based immunotherapy. Int. J. Pharm. 542, 253–265. doi:10.1016/j.ijpharm.2018.03.029
Tumeh, P. C., Harview, C. L., Yearley, J. H., Shintaku, I. P., Taylor, E. J. M., Robert, L., et al. (2014). PD-1 blockade induces responses by inhibiting adaptive immune resistance. Nature 515, 568–571. doi:10.1038/nature13954
Twibanire, J., and Grindley, T. B. (2014). Polyester dendrimers: smart carriers for drug delivery. Polymers 6, 179–213. doi:10.3390/polym6010179
van der Meel, R., Sulheim, E., Shi, Y., Kiessling, F., Mulder, W. J. M., and Lammers, T. (2019). Smart cancer nanomedicine. Nat. Nanotechnol. 14, 1007–1017. doi:10.1038/s41565-019-0567-y
Vivero-Escoto, J. L., and Huang, Y. T. (2011). Inorganic-organic hybrid nanomaterials for therapeutic and diagnostic imaging applications. Int. J. Mol. Sci. 12, 3888–3927. doi:10.3390/ijms12063888
Vivier, E., Ugolini, S., Blaise, D., Chabannon, C., and Brossay, L. (2012). Targeting natural killer cells and natural killer T cells in cancer. Nat. Rev. Immunol. 12, 239–252. doi:10.1038/nri3174
Waldman, A. D., Fritz, J. M., and Lenardo, M. J. (2020). A guide to cancer immunotherapy: from T cell basic science to clinical practice. Nat. Rev. Immunol. 20, 651–668. doi:10.1038/s41577-020-0306-5
Wang, D., Wang, T., Liu, J., Yu, H., Jiao, S., Feng, B., et al. (2016). Acid-activatable versatile micelleplexes for PD-l1 blockade-enhanced cancer photodynamic immunotherapy. Nano Lett. 16, 5503–5513. doi:10.1021/acs.nanolett.6b01994
Wang, H., and Mooney, D. J. (2018). Biomaterial-assisted targeted modulation of immune cells in cancer treatment. Nat. Mater. 17, 761–772. doi:10.1038/s41563-018-0147-9
Wang, X. (2016). Cancer Moonshot 2020: a new march of clinical and translational medicine. Clin. Transl. Med. 5, 11. doi:10.1186/s40169-016-0091-8
Wang, Ye., Santos, A., Evdokiou, A., and Losic, D. (2015). An overview of nanotoxicity and nanomedicine research: principles, progress and implications for cancer therapy. J. Mater. Chem. B. 3, 7153–7172. doi:10.1039/c5tb00956a
Wang, Yi., Lin, Y. X., Qiao, S. L., An, H. W., Ma, Y., Qiao, Z. Y., et al. (2017). Polymeric nanoparticles enable reversing macrophage in tumor microenvironment for immunotherapy. Biomaterials 112, 153–163. doi:10.1016/j.biomaterials.2016.09.034
Wayne, E. C., Long, C., Haney, M. J., Batrakova, E. V., Leisner, T. M., Parise, L. V., et al. (2019). Targeted delivery of siRNA lipoplexes to cancer cells using macrophage transient horizontal gene transfer. Adv. Sci. 6, 1900582. doi:10.1002/advs.201900582
Wei, P., Chen, J., Hu, Y., Li, X., Wang, H., Shen, M., et al. (2016). Dendrimer-stabilized gold nanostars as a multifunctional theranostic nanoplatform for CT imaging, photothermal therapy, and gene silencing of tumors. Adv. Healthc. Mater 5, 3203–3213. doi:10.1002/adhm.201600923
West, W. H. (1989). Continuous infusion recombinant interleukin-2 (rIL-2) in adoptive cellular therapy of renal carcinoma and other malignancies. Canc. Treat Rev. 16, 83–89. doi:10.1016/0305-7372(89)90027-3
Wu, J., Kamaly, N., Shi, J., Zhao, L., Xiao, Z., Hollett, G., et al. (2014). Development of multinuclear polymeric nanoparticles as robust protein nanocarriers. Angew. Chem. Int. Ed. 53, 8975–8979. doi:10.1002/anie.201404766
Xu, Z., Ma, X., Gao, Y. E., Hou, M., Xue, P., Li, C. M., et al. (2017). Multifunctional silica nanoparticles as a promising theranostic platform for biomedical applications. Mater. Chem. Front. 1, 1257–1272. doi:10.1039/c7qm00153c
Yan, H., Kamiya, T., Suabjakyong, P., and Tsuji, N. M. (2015). Targeting C-type lectin receptors for cancer immunity. Front. Immunol. 6, 408. doi:10.3389/fimmu.2015.00408
Yang, R., Xu, J., Xu, L., Sun, X., Chen, Q., Zhao, Y., et al. (2018). Cancer cell membrane-coated adjuvant nanoparticles with mannose modification for effective anticancer vaccination. ACS Nano 12, 5121–5129. doi:10.1021/acsnano.7b09041
Yang, Y., Zhang, J., Xia, F., Zhang, C., Qian, Q., Zhi, X., et al. (2016). Human CIK cells loaded with Au nanorods as a theranostic platform for targeted photoacoustic imaging and enhanced immunotherapy and photothermal therapy. Nanoscale Res. Lett. 11, 285. doi:10.1186/s11671-016-1468-8
Yao, X., Yang, P., Zin, Z., Jiang, Q., Guo, R., Xie, R., et al. (2019). Multifunctional nanoplatform for photoacoustic imaging-guided combined therapy enhanced by CO induced ferroptosis. Biomaterials 197, 268–283. doi:10.1016/j.biomaterials.2019.01.026
Yoshida, Y., Naito, M., Yamada, T., Aisu, N., Kojima, D., Mera, T., et al. (2017). Clinical study on the medical value of combination therapy involving adoptive immunotherapy and chemotherapy for stage IV colorectal cancer (COMVI Study). Anticancer Res. 37, 3941–3946. doi:10.21873/anticanres.11777
Yuan, J., Hegde, P. S., Clynes, R., Foukas, P. G., Harari, A., Kleen, T. O., et al. (2016). Novel technologies and emerging biomarkers for personalized cancer immunotherapy. J. Immunother. Cancer 4, 3. doi:10.1186/s40425-016-0107-3
Yue, W., Chen, L., Yu, L., Zhou, B., Yin, H., Ren, W., et al. (2019). Checkpoint blockade and nanosonosensitizer-augmented noninvasive sonodynamic therapy combination reduces tumour growth and metastases in mice. Nat. Commun. 10, 2025. doi:10.1038/s41467-019-09760-3
Zavaleta, C., Ho, D., and Chung, E. J. (2018). Theranostic nanoparticles for tracking and monitoring disease state. SLAS Technol. 23, 281–293. doi:10.1177/2472630317738699
Zhang, F., Li, F., Lu, G. H., Nie, W., Zhang, L., Lv, Y., et al. (2019). Engineering magnetosomes for ferroptosis/immunomodulation synergism in cancer. ACS Nano 13, 5662–5673. doi:10.1021/acsnano.9b00892
Zhang, K. L., Zhou, J., Zhou, H., Wu, Y., Liu, R., Wang, L. L., et al. (2017). Bioinspired “active” stealth magneto-nanomicelles for theranostics combining efficient MRI and enhanced drug delivery. ACS Appl. Mater. Interfaces 9, 30502–30509. doi:10.1021/acsami.7b10086
Zhang, R., Gao, S., Wang, Z., Han, D., Liu, L., Ma, Q., et al. (2017). Multifunctional molecular beacon micelles for intracellular mRNA imaging and synergistic therapy in multidrug-resistant cancer cells. Adv. Funct. Mater. 27, 1701027. doi:10.1002/adfm.201701027
Zhang, L. x., Xie, X. x., Liu, D. q., Xu, Z. P., and Liu, R. t. (2018). Efficient co-delivery of neo-epitopes using dispersion-stable layered double hydroxide nanoparticles for enhanced melanoma immunotherapy. Biomaterials 174, 54–66. doi:10.1016/j.biomaterials.2018.05.015
Zhang, L., Jing, D., Wang, L., Sun, Y., Li, J. J., Hill, B., et al. (2018). Unique photochemo-immuno-nanoplatform against orthotopic xenograft oral cancer and metastatic syngeneic breast cancer. Nano Lett. 18, 7092–7103. doi:10.1021/acs.nanolett.8b03096
Zhang, Xud., Wang, C., Wang, J., Hu, Q., Langworthy, B., Ye, Y., et al. (2018). PD-1 blockade cellular vesicles for cancer immunotherapy. Adv. Mater. 30, e1707112. doi:10.1002/adma.201707112
Zhang, Xue., Wu, F., Men, K., Huang, R., Zhou, B., Zhang, R., et al. (2018). Modified Fe3O4 magnetic nanoparticle delivery of CpG inhibits tumor growth and spontaneous pulmonary metastases to enhance immunotherapy. Nanoscale Res. Lett. 13, 240. doi:10.1186/s11671-018-2661-8
Zhang, Y., Li, N., Suh, H., and Irvine, D. J. (2018). Nanoparticle anchoring targets immune agonists to tumors enabling anti-cancer immunity without systemic toxicity. Nat. Commun. 9, 6. doi:10.1038/s41467-017-02251-3
Keywords: immunomodulation, nanomedicine, cancer, nanomaterials, theranostics, imaging, clinical trials
Citation: Khan A, Dias F, Neekhra S, Singh B and Srivastava R (2021) Designing and Immunomodulating Multiresponsive Nanomaterial for Cancer Theranostics. Front. Chem. 8:631351. doi: 10.3389/fchem.2020.631351
Received: 19 November 2020; Accepted: 22 December 2020;
Published: 29 January 2021.
Edited by:
Sudip Mukherjee, Rice University, United StatesReviewed by:
Anik Karan, Louisiana Tech University, United StatesVijay Sagar Madamsetty, Mayo Clinic Florida, United States
Copyright © 2021 Khan, Dias, Neekhra, Singh and Srivastava. This is an open-access article distributed under the terms of the Creative Commons Attribution License (CC BY). The use, distribution or reproduction in other forums is permitted, provided the original author(s) and the copyright owner(s) are credited and that the original publication in this journal is cited, in accordance with accepted academic practice. No use, distribution or reproduction is permitted which does not comply with these terms.
*Correspondence: Rohit Srivastava, cnNyaXZhc3RhQGlpdGIuYWMuaW4=