Aerobic Oxidations in Asymmetric Synthesis: Catalytic Strategies and Recent Developments
- Department of Chemistry and Biotechnology, School of Science, Tallinn University of Technology, Tallinn, Estonia
Despite the remarkable advances in the area of asymmetric catalytic oxidations over the past decades, the development of sustainable and environmentally benign enantioselective oxidation techniques, especially with the efficiency level similar to natural enzymes, still represents a challenge. The growing demand for enantiopure compounds and high interest to industry-relevant green technological advances continue to encourage the research pursuits in this field. Among various oxidants, molecular oxygen is ubiquitous, being available at low cost, environmentally benign and easy-to-handle material. This review highlights recent achievements in catalytic enantioselective oxidations utilizing molecular oxygen as the sole oxidant, with focus on the mechanisms of dioxygen activation and chirogenesis in these transformations.
Introduction
In modern organic synthesis, asymmetric oxidations are of fundamental importance. The early works of Sharpless, (Katsuki and Sharpless, 1980; Jacobsen et al., 1988; Katsuki and Martin, 2004), Jacobsen (Zhang et al., 1990; Jacobsen et al., 1988) and Shi (Tu et al., 1996) on the stereoselective epoxidation and dihydroxylation of alkenes have established prominent benchmarks, with a strong impact to the whole field of enantioselective catalysis. Owing to the valuable contributions of numerous esteemed research groups, contemporary methods of asymmetric oxidation constitute a powerful and multifunctional tool for the preparation of versatile chiral molecules. However, widespread utilization of hazardous oxidants with poor atom economy (e.g. hypochlorites, iodine(III), and iodine(V) reagents, quinones, peroxy compounds) constitutes a serious shortcoming in the view of current green chemistry paradigm (Anastas and Warner, 1998).
As a consequence, the asymmetric catalytic oxidations with molecular oxygen as a terminal oxidant can be considered as the next Frontier in organic synthesis, (Mukaiyama and Yamada, 1995; Jiao and Stahl, 2019; Sterckx et al., 2019), being especially attractive for large-scale industrial applications (Caron et al., 2006; Hone et al., 2017). Dioxygen itself is an abundant compound presented in air (21% by volume) and vital for the metabolism of aerobic organisms, thus representing an inexpensive and eco-friendly oxidizing agent. It also offers excellent atom economy: as a rule, aerobic oxidation usually produces low molecular weight by-products (e.g. H2O) or even proceeds with 100% atom efficiency (e.g. formation of peroxides). Hence, atmospheric oxygen nicely fits the green chemistry requirements, being a nearly “ideal” oxidant.
Besides the mentioned green chemistry benefits, recent accomplishments in asymmetric synthesis with atmospheric oxygen often represent prominent examples in the catalytic reaction engineering. Indeed, to ensure reliable stereodiscrimination of a prochiral substrate and enhanced reactivity of oxygen, the design of enantioselective aerobic oxidation process should commonly comprise two main components: an effective oxygen activation pathway and a proper method of chirality induction (termed “chirogenesis”) (Borovkov et al., 2001).
In its ground state, dioxygen molecule represents a stable biradical (triplet oxygen, 3O2). Although the oxidation of organic compounds is thermodynamically favorable, the reaction of triplet oxygen with usually singlet-state organic molecules is spin forbidden, hence imposing a kinetic limitation on this reaction pathway (Miller et al., 1990). As a consequence, the organic substrates can only be oxidized with triplet oxygen via a radical autoxidation process (Doering and Haines, 1954; Ingold, 1961; Porter, 2013; Poon and Pratt, 2018). Alternatively, transformation of 3O2 into another type of the oxidative species must be implemented to overcome the corresponding kinetic constraint. As one option, activation of triplet oxygen can be achieved with the aid of photocatalysis, resulting in generation of highly reactive singlet oxygen 1O2 (Figure 1A) (Zamadar and Greer, 2009; Ghogare and Greer, 2009). Another activation pathway is based on single electron transfer from a photoredox catalyst (Romero and Nicewicz, 2016) (or another monoelectronic reductant) yielding superoxide anion radical O2− (Figure 1B). Furthermore, various transition metal complexes can shuttle oxygen atoms even at ambient conditions via generation of the corresponding oxo- and peroxo species, or transfer electrons in the oxidation reactions (Figure 1C) (Miller et al., 1990; Parmeggiani and Cardona, 2012; Allen et al., 2013). The chirogenic process in transition metal-catalyzed transformations is conventionally induced by a judicious choice of chiral ligand, while photocatalytic approaches are commonly merged with asymmetric organocatalysis.
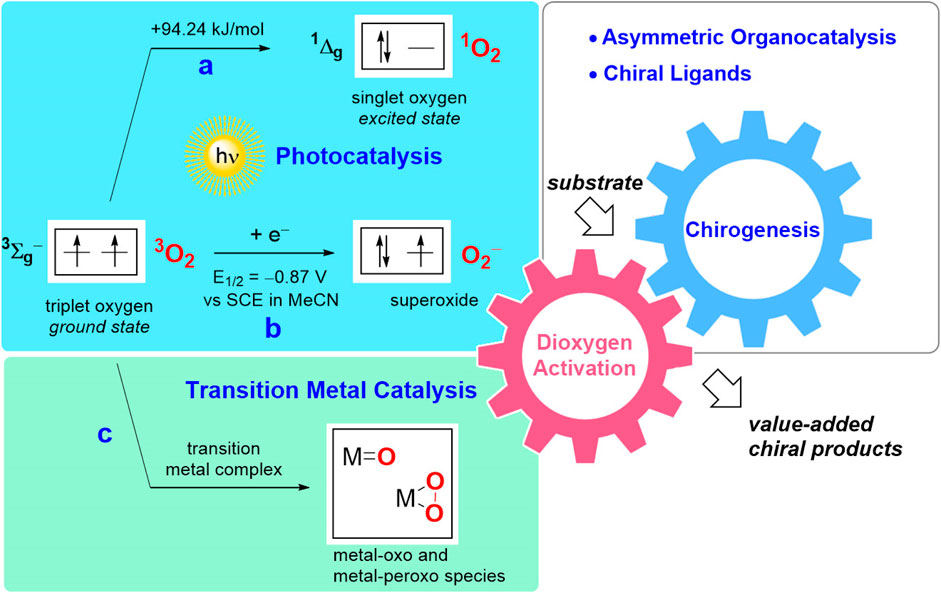
FIGURE 1. Schematic representation of O2 activation pathways and chirogenesis in enantioselective catalytic aerobic oxidations: (A) generation of singlet oxygen; (B) photoredox catalysis yielding a superoxide anion; (C) transition metal catalysis.
It is remarkable that the mechanisms outlined above are also relevant for the operation of iron- (Meunier et al., 2004), copper- (Festa and Thiele, 2011), manganese- (Dismukes, 1996), and metal-free flavin-containing enzymes, which provide an inspiration for designing the analogs of the enzymatic reactions (de Gonzalo and Fraaije, 2013; Petsi and Zografos, 2018). Many of these transformations involve synergetic or dual catalytic cycles, featuring masterpieces in the catalytic reaction engineering.
The main aim of the current review is to outline the key catalytic strategies and latest innovative concepts in design of the asymmetric reactions with molecular oxygen as a terminal oxidant. Therefore, the most prominent examples have been judiciously selected among the research works published in the last 20 years. However, for a more detailed overview of the field, especially earlier contributions, a number of comprehensive reviews (Uchida and Katsuki, 2013; Bryliakov, 2015; Bryliakov, 2017; Ottenbacher et al., 2018), and personal accounts (Mukaiyama and Yamada, 1995; Irie and Katsuki, 2004) can be recommended for further reading.
The first part of the review describes transformations mediated by transition metals. Besides asymmetric oxidations mediated by chiral metal complexes, dual catalytic processes are also mentioned, in which the aerobic oxidation step is used solely to generate a prochiral intermediate for the subsequent asymmetric reaction. The second part focuses on metal-free aerobic oxidations, commonly operating via dual photo-organocatalytic cycles. It worth mentioning that enantioselective oxidations mediated by enzymes, (Endoma et al., 2002; Boyd and Bugg, 2006; Gandomkar et al., 2019), heterogeneous catalysts, (Miyamura et al., 2013), and nanoparticles (Miyamura et al., 2015; Hadian-Dehkordi and Hosseini-Monfared, 2016) also represent an important cluster of valuable synthetic methodologies, however these approaches are out of the scope of this review.
Transition Metal-Catalyzed Transformations
Transition metal-catalyzed transformations constitute the mainstream approach in enantioselective aerobic oxidations. In general, the corresponding catalytic cycles mimic enzymatic activity of metal-containing oxidases and oxygenases (Figure 2), (Bugg, 2003; McCann and Stahl, 2015) with asymmetry typically induced by a chiral environment. Besides being an oxidant, transition metal can also act as a Lewis acid to enable substrate coordination and to facilitate stereodiscrimination of the enantiomeric pairs in oxidative kinetic resolution process.
Among the recent advances, synergetic transition metal-photocatalysis (Ding et al., 2017) can be mentioned as a more intricate reaction design, in which photochemically-generated singlet oxygen is exploited.
The transformations presented in this section are subdivided according to the catalytic strategy and origin of chirogenesis. While the majority of enantioselective metal-catalyzed aerobic oxidations relies on the use of a single chiral transition metal catalyst, several cascade or dual catalytic transformations are also known, in which aerobic oxidation cycle is used solely to generate a reactive prochiral intermediate (Figure 3). In the latter approach, chirality is typically induced in an additional catalytic cycle, as presented in the second subsection.
Asymmetric Aerobic Oxidations Mediated by Chiral Metal Complexes
Application of the transition metal complexes as chiral oxygen carriers in asymmetric aerobic oxidations was pioneered by the Mukaiyama’s group in 1990s, (Mukaiyama and Yamada, 1995) which designed a number of optically active MnIII complexes to perform the enantioselective epoxidation of olefins (Yamada et al., 1992; Mukaiyama et al., 1993; Nagata et al., 1994; Yamada et al., 1994) and oxidation of sulfides to chiral sulfoxides (Imagawa et al., 1995; Nagata et al., 1995). These keynote contributions were followed by the discovery of chiral nickel (Kureshy et al., 1998) and ruthenium complexes, (Kureshy et al., 1997; Lai et al., 1998), which are capable to mediate the enantioselective aerobic epoxidation of olefins, and works of the Bolm’s group on the copper-catalyzed Baeyer-Villiger oxidation of cyclic ketones (Bolm et al., 1994; Bolm and Schlingloff, 1995; Bolm et al., 1997). It should be noted that these prominent early contributions have been extensively discussed in several reviews (Mukaiyama and Yamada, 1995; Bryliakov, 2017; Ottenbacher et al., 2018). The use of chiral metal complexes still represent a prevalent approach among the known aerobic oxidation methods.
In 2000, Katsuki et al. reported a highly efficient protocol for chemo- and enantioselective aerobic oxidation of secondary alcohols 1 under visible-light irradiation, mediated by optically active (nitroso)-salen ruthenium complex 2 (Scheme 1) (Masutani et al., 2000). The reaction mechanism was suggested on the basis of kinetics and kinetic isotope effect studies (Shimizu et al., 2005). It was suggested that irradiation triggers the dissociation of complex 2 and release of NO ligand, thus providing a free coordination site for the alcohol substrate. The produced RuIII complex A captures molecular oxygen to afford RuIV peroxo complex B, where the peroxo ligand accepts α-carbynol hydrogen of the alcohol ligand via a hydrogen atom transfer (HAT) process. The resulting ketone-anchoring RuIII species C finally recovers the complex A via replacement of the ketone ligand with the next molecule of alcohol. In a more recent study the same group demonstrated that a broad range of alcoholic substrates can be employed in aerobic oxidative kinetic resolution even without irradiation, just with the aid of (aqua)-salen ruthenium complex 3 (Mizoguchi et al., 2014). Applications of chiral (NO)Ru-salen complexes in enantioselective aerobic oxidations showed a great potential for further development and have been expanded far beyond the kinetic resolution of alcohols in subsequent works of the same group (Irie and Katsuki, 2004). Thus, similar ruthenium-based catalysts have been successfully used in the enantioselective oxidative couplings of 2-naphthols, (Irie et al., 2000), radical cyclization of 2,2′-dihydroxystilbene, (Masutani et al., 2002), and asymmetric desymmetrization of meso-diols (Shimizu et al., 2002; Shimizu and Katsuki, 2003).
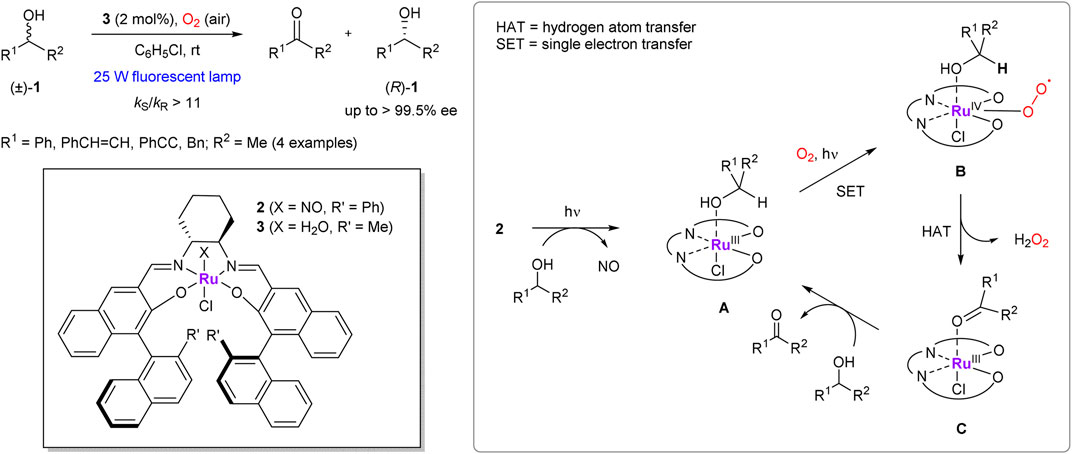
SCHEME 1. Aerobic oxidative kinetic resolution of secondary alcohols with Ru-salen complexes 2, 3 and a simplified representation of its mechanism (Masutani et al., 2000; Shimizu et al., 2005).
Katsuki’s chiral Ru-salen complexes are also capable to mediate the “oxygenase type” transformations, such as epoxidation of olefins and oxidation of sulfides (representative examples 6 and 7 are shown in Scheme 2) (Tanaka et al., 2010; Koya et al., 2012). In comparison with early works on the enantioselective Ru-catalyzed aerobic epoxidations of olefins, (Kureshy et al., 1997; Lai et al., 1998), Katsuki’s protocol did not require an aldehyde co-reductant or high oxygen pressure and operates at ambient conditions. Interestingly, water was found to be an essential additive, which serves as a proton transfer mediator.
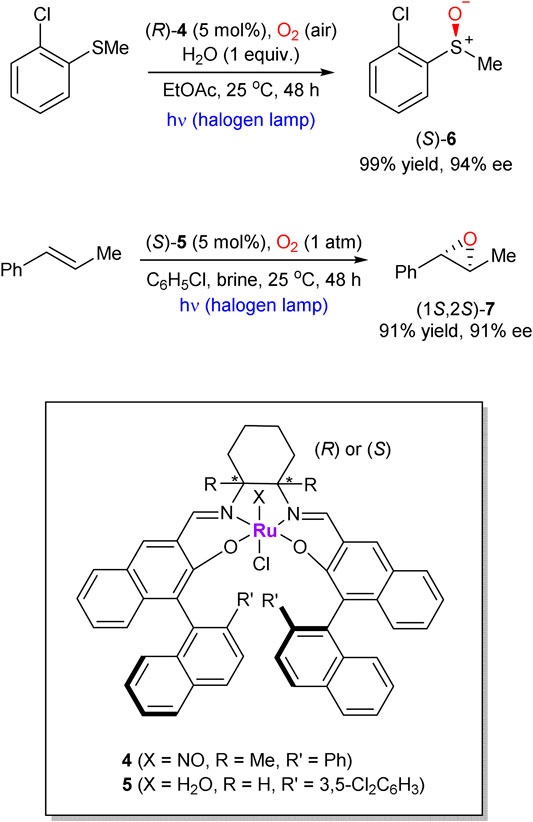
SCHEME 2. Photo-promoted Ru-catalyzed enantioselective sulfide oxidation and alkene epoxidation with molecular oxygen (Tanaka et al., 2010; Koya et al., 2012).
Palladium is one of the most versatile and prominent transition metals in catalysis, including its well-documented role in aerobic oxidative transformations (Stahl, 2004). In 2001, the enantioselective palladium-catalyzed oxidation of secondary alcohols with molecular oxygen was independently reported by Stoltz’s (Ferreira and Stoltz, 2001) and Sigman’s groups (Jensen et al., 2001) (Scheme 3). The chirality was enabled by (–)-sparteine ligand, which also acted as a base. Later, Stoltz and co-workers presented application of this methodology to obtain pharmaceutically potent building blocks (Caspi et al., 2004) and in enantioselective synthesis of several alkaloids (Scheme 3) (Krishnan et al., 2008). The precursors 8–11 of the targeted natural products were prepared with exceptional enantioselectivity (90–96% ee) and respectable yields (compared to 50% theoretical maximum), enabling facile preparation of the required chiral motifs. Noteworthy, several functional groups within the substrates (e.g., free hydroxyls, esters) were compatible with the reaction conditions.
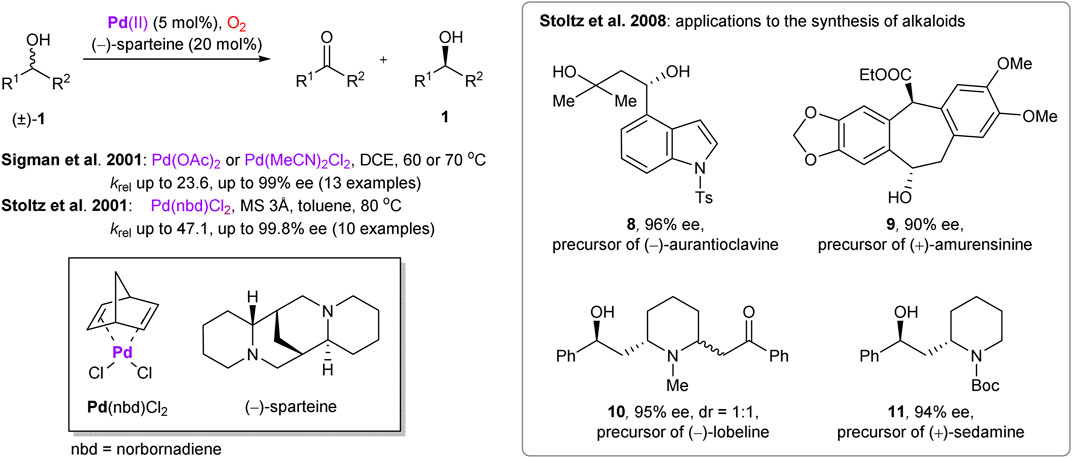
SCHEME 3. Palladium-catalyzed aerobic oxidative kinetic resolution of secondary alcohols and its application in the total synthesis of alkaloids (Ferreira and Stoltz, 2001; Jensen et al., 2001; Caspi et al., 2004; Krishnan et al., 2008).
In recent decades, investigation of the catalytic reactions with earth-abundant transition metals as sustainable alternatives to precious metal catalysts has become a general trend. Several chiral complexes of the first-row transition metals have been applied in the catalytic oxidative kinetic resolutions of secondary alcohols with molecular oxygen as an oxidant. In these transformations, transition metals acted as oxygen carriers and/or chiral Lewis acids to enable stereodifferentiation of racemic substrates.
Thus, Chen et al. developed a series of chiral N-salicylidene vanadyl carboxylate complexes to perform catalytic kinetic resolution of α-hydroxycarboxylic esters, thioesters, amides (Weng et al., 2006; Chen et al., 2007; Salunke et al., 2011) and α-hydroxyketones (Chen et al., 2011) by using molecular oxygen as the terminal oxidant at ambient conditions. It was found that aerobic oxidation of (S)-alcohols proceeds more readily than their (R)-counterparts in the presence of vanadium complex 12 and its congeners (Scheme 4). As a notable example illustrating high oxidation selectivity, (R)-isomer of N-benzylamide 13 was obtained in 50% yield and 98% ee (Weng et al., 2006). Drastically slower rate of the oxidation for (R)-isomer (krel = 458) was rationalized based on the results of single crystal X-ray analysis of the corresponding catalyst-substrate adduct 14. It was found that α-carbinol hydrogen of (R)-13 is shielded with a neighboring tert-butyl group of the chiral ligand, thus making it barely accessible for elimination through a two-electron oxidation process (Weng et al., 2006). However, the shielding is absent in the diastereomeric complex with (S)-13, what results in its fast transformation into the α-ketoamide oxidation product and low-valent vanadium complex. Reoxidation of the latter with molecular oxygen recovers the reactive vanadium (V) species. As a further development, reusable polystyrene-supported vanadium catalysts have also been prepared to promote oxidations of α-hydroxy (thio)esters and amides with enantioselectivities of up to 99% ee (Salunke et al., 2011).
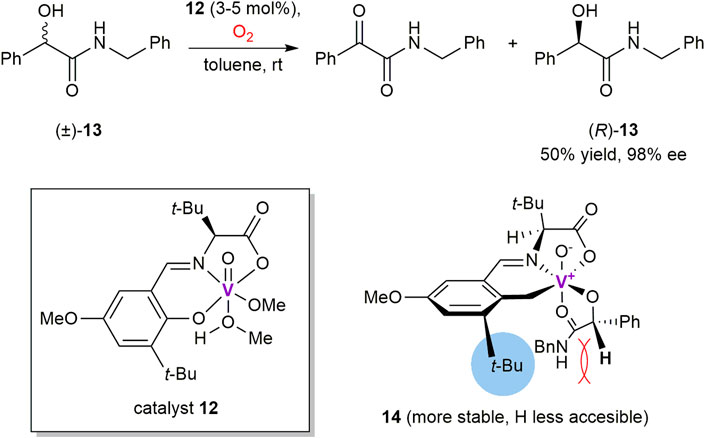
SCHEME 4. Kinetic resolution of N-benzyl mandelamide 13 via vanadium-mediated aerobic oxidation (Weng et al., 2006).
Alamsetti and Sekar reported the first cobalt-catalyzed oxidative kinetic resolution of α-hydroxyesters 15 with molecular oxygen as the sole oxidant (Scheme 5) (Alamsetti and Sekar, 2010). However, 2,2,6,6-tetramethylpiperidin-1-oxyl (TEMPO) was required as a co-catalyst (Wertz and Studer, 2013). Among several cobalt salts and chiral ligands screened, Co(OAc)2 and salen ligand 16 provided the best reaction outcome with racemic methyl mandelate 15 (R = Me, Ar = Ph). The developed conditions were also suitable for a range of α-hydroxyesters, with the maximum selectivity of 99.9% ee achieved for allyl mandelate. Although no mechanistic studies have been performed and a role of cobalt as a potent oxygen carrier has not been manifested, coordination of 15 to the chiral metal complex producing diastereomeric complexes with the different rates of TEMPO-mediated aerobic oxidation could be suggested as a plausible scenario.
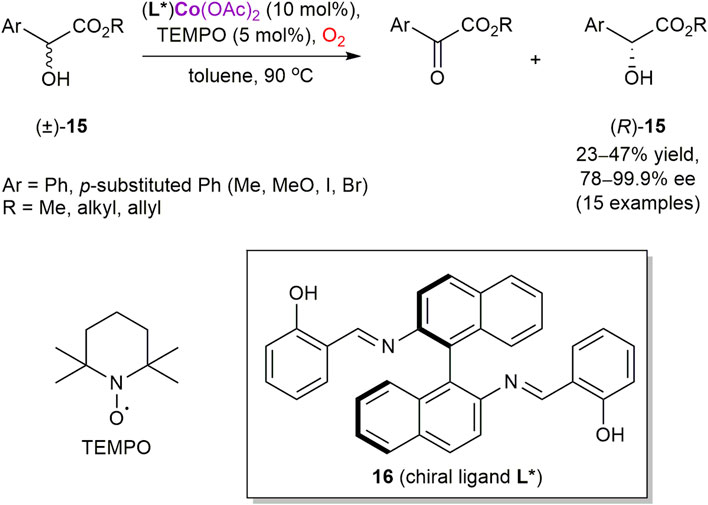
SCHEME 5. Cobalt-catalyzed enantioselective aerobic oxidation of α-hydroxy esters (Alamsetti and Sekar, 2010).
The same group applied similar methodology for the kinetic resolution of benzoin 17 via the TEMPO-catalyzed aerobic oxidation in the presence of a chiral zinc complex, generated in situ from ZnSO4 and salen ligand 18 (Scheme 6) (Muthupandi and Sekar, 2011). This approach yields enantiomerically enriched 17 with low 43% ee. Since zinc possesses a single stable oxidation state +2 in its compounds, complex 17 could only play a role of chiral Lewis acid, while the oxidation should occur as a nitroxide-catalyzed process (Wertz and Studer, 2013).
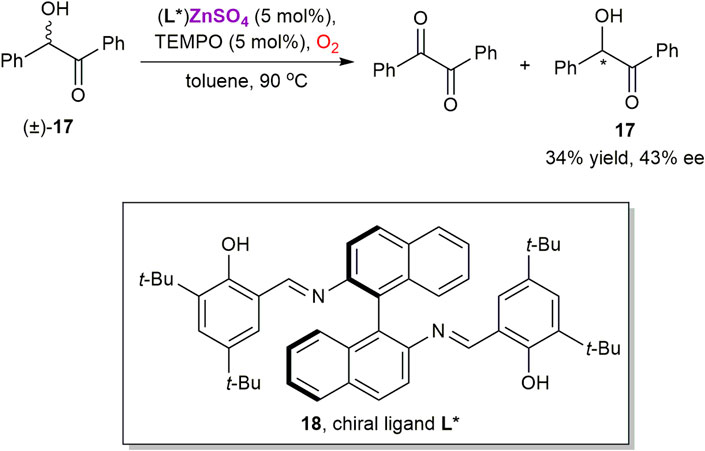
SCHEME 6. Oxidative kinetic resolution of (±)-benzoin 17 using chiral zinc catalyst (Muthupandi and Sekar, 2011).
Dioxygen has also been utilized as an oxidant in the asymmetric dehydrogenative C–C coupling reactions. A notable example of such transformation was developed by Egami and Katsuki, who reported in 2009 an aerobic oxidative coupling of 2-naphthols mediated by Fe(salen) complexes (Scheme 7) (Egami and Katsuki, 2009). Chiral iron(III) complex 19 was found to be especially effective for the asymmetric coupling of 3-substituted 2-naphthols 20, featuring bi-2-naphthols (R)-21 with enantioselectivities greater than 90% ee, with the only exception of methyl-substituted compound (R = Me, 77% ee). The mechanistic studies suggested the coordination of a naphthol substrate to FeIII in a chiral complex, followed by the “oxidase mode” SET oxidation with dioxygen as a rate-determining step. This process generates the radical cationic naphthol species, undergoing subsequent cross-coupling with the anionic 2-naphthol counterpart (Matsumoto et al., 2012; Uchida and Katsuki, 2013).
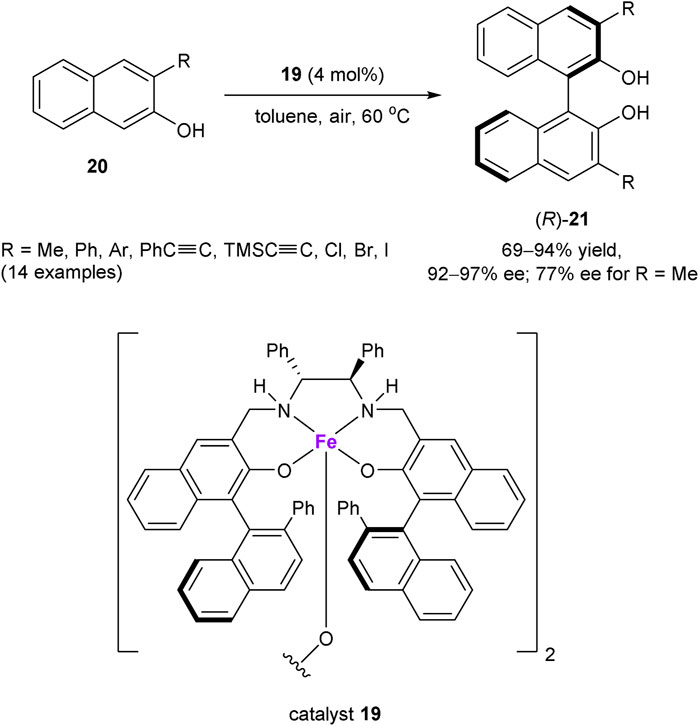
SCHEME 7. Iron-catalyzed aerobic oxidative coupling of 2-naphtols (Egami and Katsuki, 2009).
Chiral binuclear copper oxo-complex 22, generated in situ from (–)-sparteine, Cu(CH3CN)4PF6 and molecular oxygen, was introduced by the Porco group to perform asymmetric dearomatization of phenolic compounds (representative example is shown in Scheme 8). The approach was successfully applied as a key step in asymmetric synthesis of natural products, such as azaphilones (Zhu et al., 2005; Zhu and Porco, 2006; Qian et al., 2007; Germain et al., 2011).
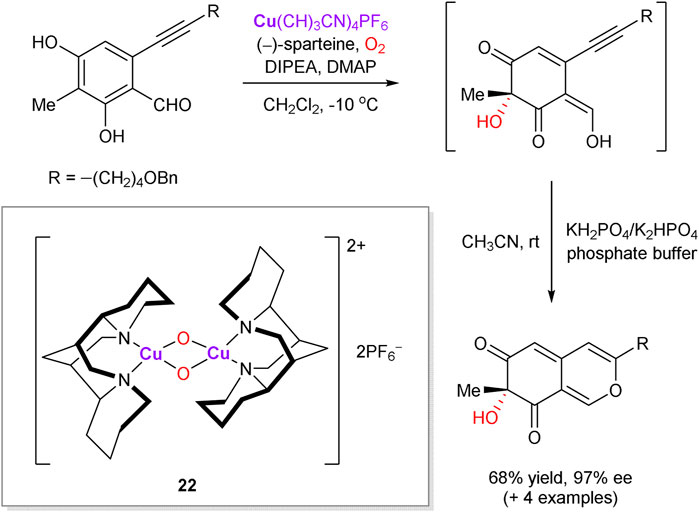
SCHEME 8. Copper-mediated enantioselective oxidative dearomatization of phenols (Zhu et al., 2005).
In contrast to the mainstream use of transition metals as oxygen carriers or redox catalysts, Xiao et al. developed a synergetic transition metal-photocatalytic approach, which was applied for highly enantioselective α-hydroxylation of β-ketoesters and β-ketoamides 23 (Scheme 9) (Ding et al., 2017). A family of visible-light-responsive chiral ligands was prepared by grafting a photosensitive thioxanthone motif onto a chiral bisoxazoline scaffold. In the catalytic complex, the thioxanthone motif acts as a triplet-state sensitizer to enable photogeneration of singlet oxygen, while the Ni2+ cation acts as a Lewis acid to coordinate β-keto ester substrate 23 in the enolate form. Besides the preparation of indanone-derived α-hydroxylated esters and amides (e.g. 24a and 24b), the reaction protocol was also suitable for α-hydroxylation of heterocyclic and even aliphatic substrates (e.g. 24c and 24d). Several functional groups prone to oxidation (alkynyl, vinyl, thiophene, etc.) were inert under the reaction conditions. Asymmetric induction model was proposed, assuming the most preferable coordination of 23 with the most distant position of the bulky adamantyl group (1Ad) from the chiral ligand. Such mode of the coordination enables the Re-face attack of oxidants (either activated 1O2 or peroxide 25), since the Si-face is blocked by the rear phenyl groups of the ligand. The model agrees with the experimentally assigned (R)-configuration of product 24a.
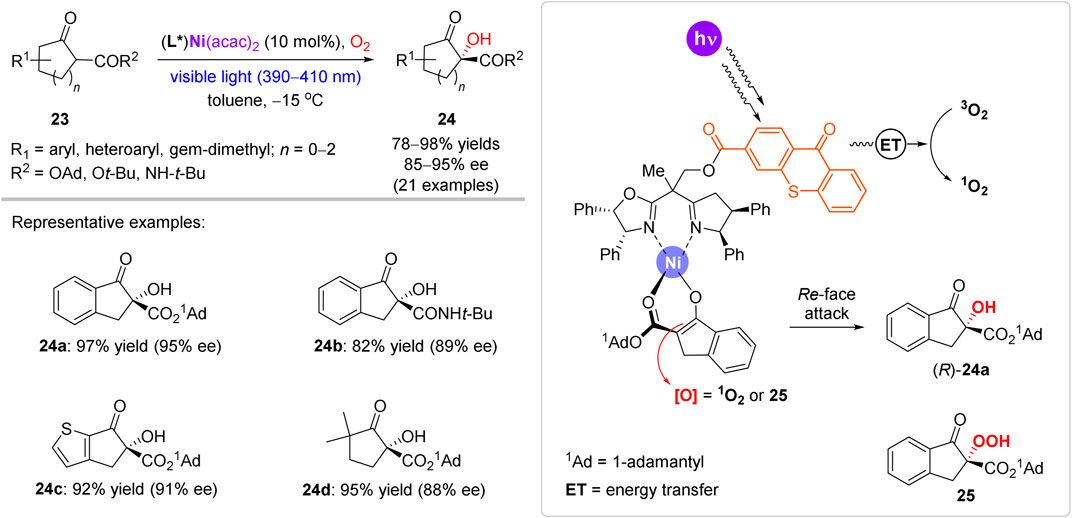
SCHEME 9. Enantioselective aerobic oxidation of β-ketoesters mediated by a chiral nickel complex (Ding et al., 2017).
Dual Catalytic Transformations With a Complementary Asymmetric Step
Another important group of synthetic methods is based on the generation of a prochiral oxidized intermediate, which is subsequently involved into a complementary asymmetric catalytic cycle (Figure 3). Commonly, but not necessarily, the first oxidative catalytic step is transition metal-mediated, while the second asymmetric step is organocatalytic.
Hence, the enantioselective synthesis of 4H-chromenes 26 from 2-alkyl-substituted phenols 27 and β-diketones 28 was developed by the Schneider group (Scheme 10) (Gebauer et al., 2017). The process consists of two stages and features a relay catalysis approach with two in situ generated manganese catalysts. In particular, the corresponding β-diketonato complexes of MnIII, generated from Mn(dbm)3 pre-catalyst and β-diketones 28, were identified as superior oxygen carriers to perform in situ generation of transient ortho-quinone methides 29 by oxidation of phenols 27. At the second stage, asymmetric Michael addition of 28 to 29 was occurred and mediated by another type of the chiral MnIII complexes, generated from MnIII diketonates and enantiopure phosphoric acid HX*. The process furnished 4H-chromenes 26 in up to 79% yield and 74% ee. However, the reaction outcome was found to be very sensitive to structural changes in the substrates 27 and 28.
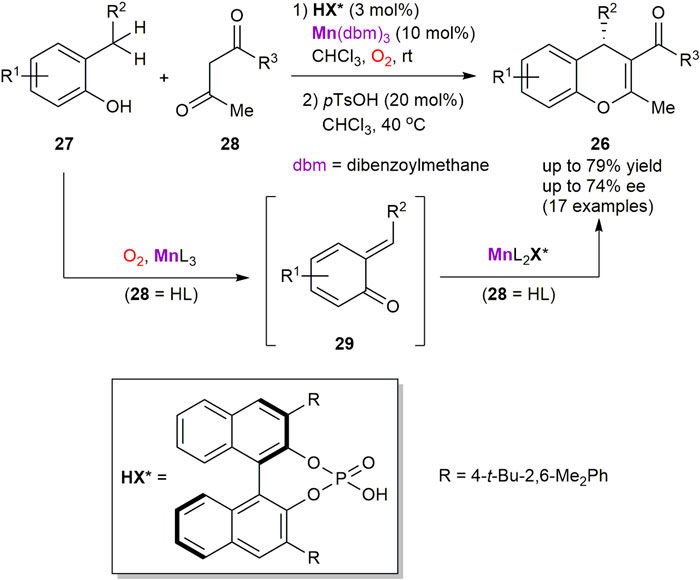
SCHEME 10. Asymmetric synthesis of 4H-chromenes (Gebauer et al., 2017).
In another representative example, Kananovich’s group developed a two-step and one-pot protocol for the asymmetric synthesis of epoxy ketones 30 from easily available tertiary cyclopropanols 31 (Scheme 11) (Elek et al., 2017). Oxidation of 31 with atmospheric oxygen proceeded readily in THF in the presence of manganese(III) acetylacetonate catalyst, to afford prochiral 1,2-dioxolanes 32a (equilibrating with β-peroxo ketones 32b) in nearly quantitative yields. The intermediates 32 were further converted into chiral epoxy ketones 30 by treatment with 1,8-diazabicyclo[5.4.0]undec-7-ene (DBU) in the presence of immobilized on silica gel poly-L-leucine (PLL) catalyst. The experimental protocol is operationally simple and affords 30 in generally high yields and enantioselectivities (80–97% ee). Several functionalities in the R group of 31 tolerate the reaction conditions, except of the metal-coordinating (e.g. amine) and base-sensitive moieties. The α-helical structure of PLL catalyst is responsible for the supramolecular binding of prochiral substrate 32 and favors the corresponding transition state (TS≠) leading to the R-enantiomer of 30 (Kelly and Roberts, 2004; Berkessel et al., 2006). The developed transformation was successfully applied in a short and stereodivergent synthesis of chlamydocin, a natural histone deacetylase inhibitor with chiral epoxy ketone warhead (Elek et al., 2019).
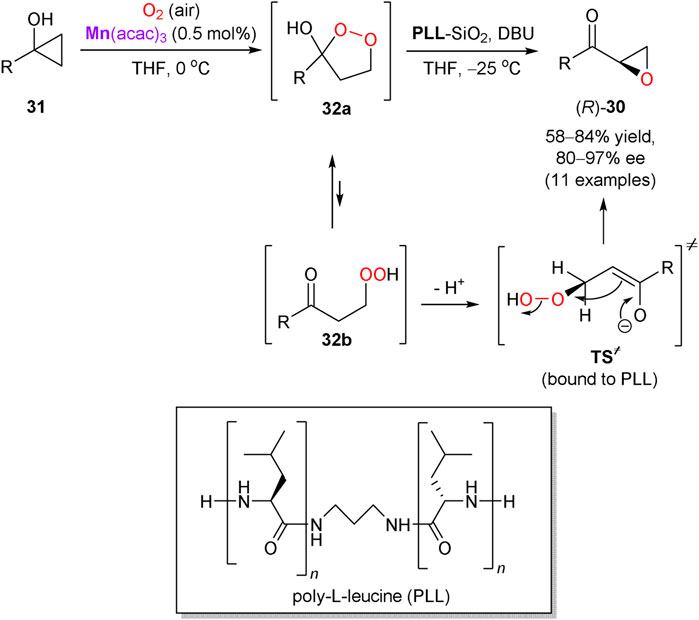
SCHEME 11. Asymmetric synthesis of epoxy ketones via aerobic oxidation of cyclopropanols (Elek et al., 2017).
Several enantioselective cascade transformations with reactive intermediates generated by aerobic oxidation in the presence of copper salts have also been reported. Thus, Whiting (Chaiyaveij et al., 2011) and Alaniz (Frazier et al., 2011; Frazier et al., 2012) independently disclosed a convenient protocol for the generation of nitrosocarbonyl compounds (e.g. 33, Scheme 12B) by copper-catalyzed aerobic oxidation of N-protected hydroxylamines 34. These reactive electrophilic nitroso compounds have been utilized in a number of robust transformations, including the asymmetric ene reactions (Scheme 12A), (Frazier et al., 2011) inter- and intramolecular hetero-Diels–Alder reactions, (Chaiyaveij et al., 2011; Frazier et al., 2012) α-amination of silyl enol ethers, (Sandoval et al., 2015) β-dicarbonyl compounds (Scheme 12B), (Xu et al., 2014) and α-hydroxylation of β-ketophosphonates 35 (Scheme 12C) (Maji and Yamamoto, 2014; Maji and Yamamoto, 2015). The asymmetric α-amination of β-ketocarbonyl compounds under aerobic conditions, developed by Luo et al., can represent a notable example (Scheme 12B) (Xu et al., 2014). In this transformation, aerobic generation of nitrosocarbonyls was beneficially merged with enamine catalysis enabled by a chiral primary amine 36. The process featured high chemo- and enantioselectivity for a broad range of β-ketocarbonyl substrates, for example β-ketoester 37 furnished the corresponding amination adduct (R)-38 in 97% yield and 96% ee. Based on the absolute configuration of product 38, as well as X-ray crystal and solution structures of enamine intermediate 39, the transition state was suggested to account for the observed stereoselectivity, in which the hydrogen-bonding network between 39 and 33 facilitates the Re-face attack of enamine to give adduct 38 with the (R)-configuration.
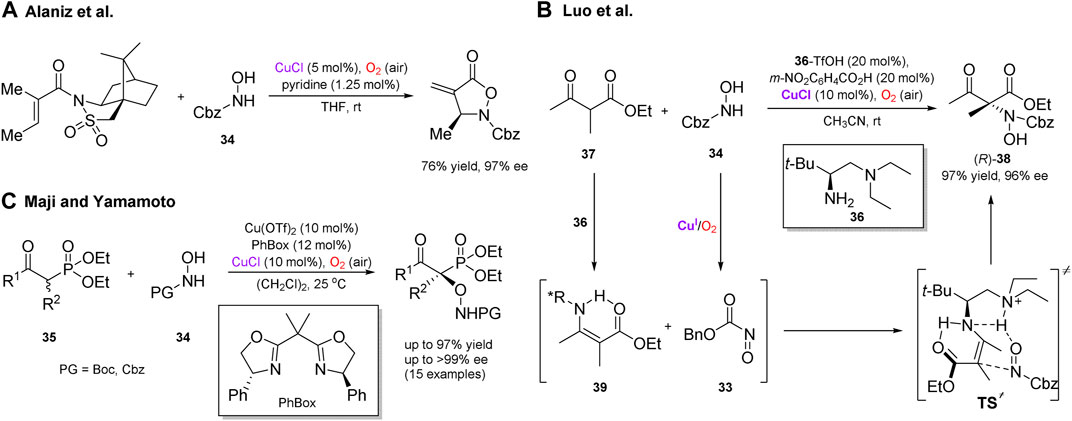
SCHEME 12. Versatile asymmetric transformations with nitroso compounds generated in situ by aerobic oxidation (Frazier et al., 2011; Xu et al., 2014; Maji and Yamamoto, 2015).
An interesting example of the enantioselective assembly of structurally complex 5,5,5-tricyclic products 40 with eight stereocenters via the multicomponent cascade reaction was described (Scheme 13) (Potowski et al., 2015). The cascade process is triggered by copper-catalyzed aerobic oxidation of cyclopentadiene 41 to cyclopentadienone 42. It was supposed that the allylic C–H oxidation of 41 is mediated by the copper peroxo-complex 43, formed by trapping molecular oxygen by Cu(CH3CN)4BF4 in the presence of (R)-Fesulphos ligand 44. The subsequent double catalytic asymmetric 1,3-dipolar cycloaddition of azomethine ylides, derived from glycine ester imines 45, delivers the final tricyclic product 40. In turn, azomethine ylides are generated by deprotonation of the glycine ester imine ligand in the chiral copper complex 46. The step-wise double cycloaddition proceeds via the endo transition state with 42 (or intermediate 47) approaching from the Re-face of ylide (with respect to C=N) to avoid unfavorable interactions with the t-Bu group of the ligand 44.
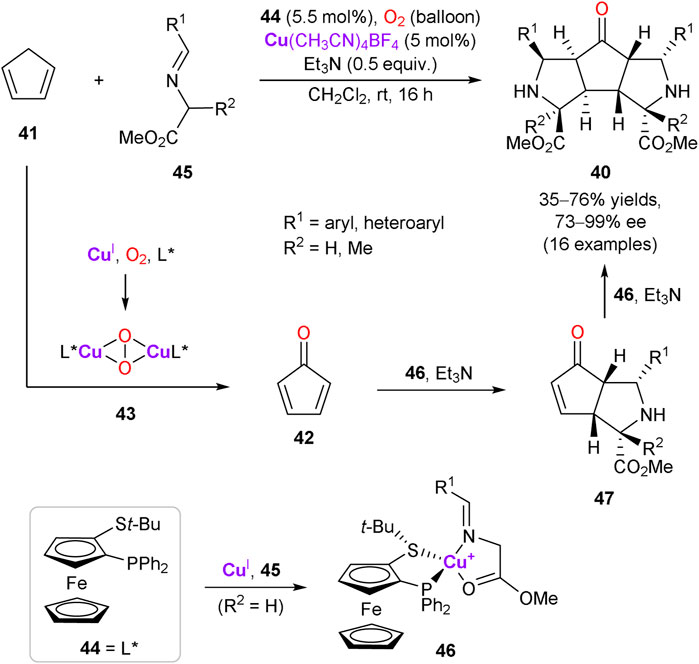
SCHEME 13. Catalytic aerobic oxidation of cyclopentadiene and tandem enantioselective cycloaddition (Potowski et al., 2015).
In 2017, Khan et al. described a visible-light enabled enantioselective cross dehydrogenative coupling between tetrahydroisoquinoline derivatives 48 and alkynes 49, using molecular oxygen as the sole oxidant (Scheme 14) (Kumar et al., 2017). The reaction was mediated by in situ generated copper complex with chiral salen ligand 50 and Rose Bengal as a complementary photo-redox catalyst, furnishing alkynylation products 51 in up to 90% yield and up to 99% ee. According to the suggested reaction mechanism, prochiral iminium ion 52 is produced by oxidation of 48 in the photo-redox catalytic cycle. In turn, the chiral copper complex (L*)CuOTf activates terminal alkyne 49 and serves as a precursor of chiral acetylide species 53, which affords the optically active coupling product 51 after addition to the intermediate imine 52.
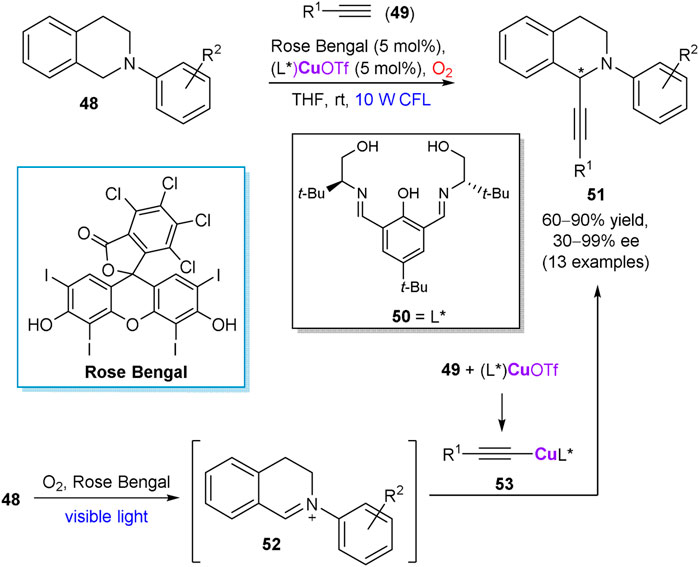
SCHEME 14. Enantioselective cross dehydrogenative coupling of alkynes 49 with tetrahydroisoquinoline derivatives 48 (Kumar et al., 2017).
Copper salts are also able to assist in the recovery of catalytically active nitroxide radical species by oxidation with molecular oxygen. Hence, Einhorn et al. disclosed kinetic resolution of oxazolidines 54 by the oxidative ring-opening, catalyzed by chiral N-hydroxyphthalimides (NHPI) and copper(I) chloride (Scheme 15) (Nechab et al., 2007). Fast reaction rates for the aerobic oxidation of 54 and selectivity factors s > 20 were observed for a range of the substrates with the most efficient chiral NHPI catalyst 55. As notable examples, o-bromophenyl-substituted oxazolidine (R)-54a was obtained in 97% ee at 58% conversion, while o-iodophenyloxazolidine 54b had the ee value of 60% at 39% conversion that corresponds to s > 50. The reaction proceeds via intermediate phthalimide N-oxyl radical (PINO), which enables a hydrogen atom transfer from the substrate 54. While a precise role of copper is unclear, it could be attributed to the formation of μ-oxocopper(II) species by the reaction of CuCl with dioxygen, which are capable to generate PINO from 55 (Nechab et al., 2004).
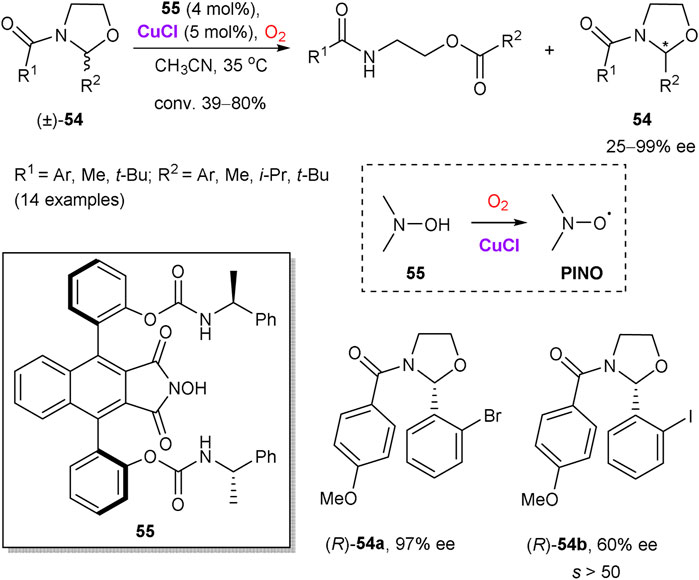
SCHEME 15. Copper-catalyzed oxidative kinetic resolution of oxazolidines 54 (Nechab et al., 2007).
The last representative example in this subtopic is based on the work of Wang et al., who demonstrated that coordinatively unsaturated RuCl3 can act as a synergistic co-catalyst in tandem with chiral N-heterocyclic carbenes (NHC) (Wang et al., 2018). The authors performed the aerobic [3 + 3] annulation reaction of β-dicarbonyl compounds 56 and enals 57 to access enantiomerically enriched lactones 58 in the presence of carbene precursor 59 (Scheme 16). The developed methodology exhibits a broad substrate scope, such as enals with complicated skeletons and diketones with exceptional structural diversity (23 examples in total). The obtained chemical yields were good-to-excellent along with the enantiomeric purity of up to 94% ee. According to the mechanistic studies, the reaction proceeds via acyl azolium intermediate D, generated by the ruthenium-catalyzed aerobic oxidation of homoenolate E. As a further extension, azolium dienolate intermediates can be generated from β,β-disubstituted enals, which smoothly afford δ-chiral lactones with 80–84% ee upon the [4 + 2] annulation reaction with trifluoromethyl ketones.
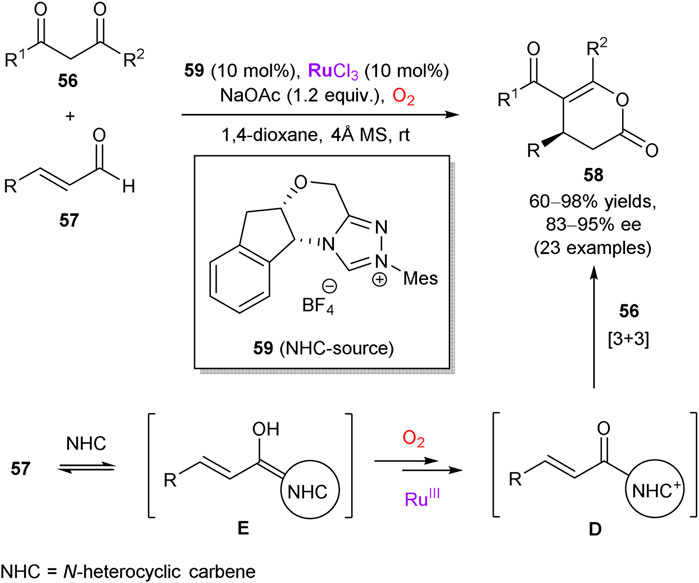
SCHEME 16. Aerobic oxidation/annulation cascade through synergistic catalysis of RuCl3 and N-heterocyclic carbene (NHC) precursor 59 (Wang et al., 2018).
Metal-Free Catalytic Systems
Another important synthetic strategy of asymmetric aerobic oxidations is based on the metal-free catalytic systems. The research activity in this field was commenced in the late 1980s by Shioiri, who described the first α-hydroxylation of achiral ketones with molecular oxygen in a two-phase system, which was mediated by chiral phase transfer catalysts (PTC) and triethyl phosphite as a co-reductant (Masui et al., 1988). In 1995, Brussee utilized chiral aza-crown ethers for the same purpose (de Vries et al., 1995). The chiral phase transfer catalysis still represent the most frequently exploited approach among the organocatalytic aerobic oxidation methods. Importantly, a specific dioxygen activation process may not be required, since many enolizable carbonyl compounds can readily react with molecular oxygen under basic conditions (Doering and Haines, 1954; Gardner et al., 1968; Konen et al., 1975). As a rule, the organocatalytic methods are tolerant to both air and water what makes them especially attractive for various practical applications.
Following the seminal works of Shioiri (Masui et al., 1988) and Brussee, (de Vries et al., 1995) in 2008, Itoh group reported asymmetric hydroxylation of oxindoles 60 in the biphasic solvent system (toluene/water), mediated by chiral cinchonidine-derived PTC 61 (Scheme 17) (Sano et al., 2008). In this transformation, a set of 3-alkyl-, alkenyl-, and alkynyl-substituted oxindoles 60 were hydroxylated by a simple reaction of oxindole-derived enolates with molecular oxygen and triethyl phosphite, acting as a reductant for peroxide intermediates. The process furnished the corresponding 3-hydroxylated oxindole products 62 in up to quantitative yields and 67–93% enantioselectivities. The (R)absolute configuration at the stereogenic center in 62 (for R = allyl) was confirmed by chemical correlation with the known indole compound. In 2012, Tan and Jiang designed similar transformation, utilizing pentanidinium PTC 63 (Yang et al., 2012). In comparison to the Itoh’s work, in this case a lower catalyst loading (5 mol%) was sufficient and no additional reductant such as triethyl phosphite was necessary. In 2015, Zhao et al. followed a similar phase-transfer catalytic strategy with 1,2-bis(diphenylphosphino)ethane or triethyl phosphite as reductants to perform the aerobic enantioselective α-hydroxylation of cyclic and acyclic ketones with higher yields and enantioselectivities achieved for cyclic ketones (representative examples are shown in Scheme 18). Dimeric PTC 64 was utilized and provided excellent stereoinduction at only 5 mol% loading (Sim et al., 2015).
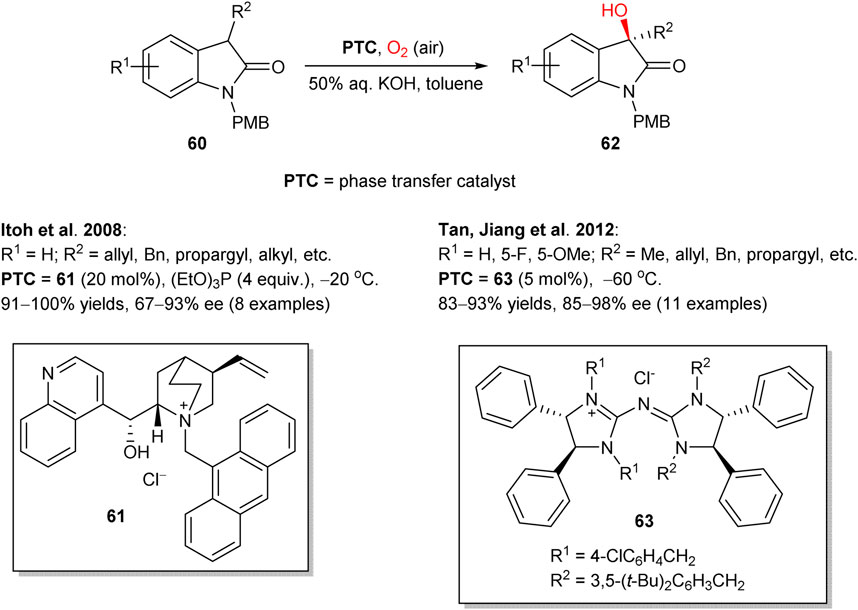
SCHEME 17. Asymmetric hydroxylation of oxindoles 60 under phase transfer catalysis (Sano et al., 2008; Yang et al., 2012).
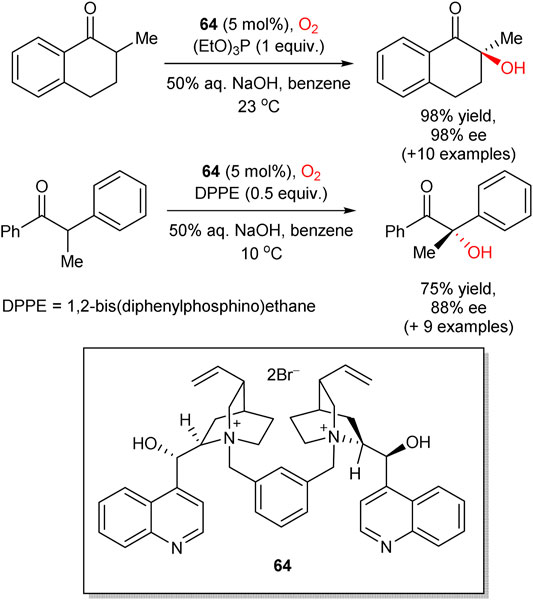
SCHEME 18. Enantioselective α-hydroxylation of cyclic and acyclic ketones (Sim et al., 2015).
Several recent developments relied on combined photo-organocatalysis to produce more reactive oxygen species, as schematically outlined in Figure 4. The photochemical activation of dioxygen delivers highly reactive singlet oxygen in the presence of organic dyes acting as photosensitizers. Alternatively, a photoredox catalytic cycle can be involved. In such a case, the generation of superoxide anion (O2−) in an oxidative quenching cycle can be considered as a plausible O2 activation pathway. Subsequent asymmetric induction and activation of a substrate take place in a complementary organocatalytic cycle, which may include different types of chiral organocatalytic species, e.g. secondary amines, hydrogen bonding catalysts, phase transfer catalysts. Notable, an organocatalytic molecule and an organic dye can be covalently linked to form a bifunctional catalyst.
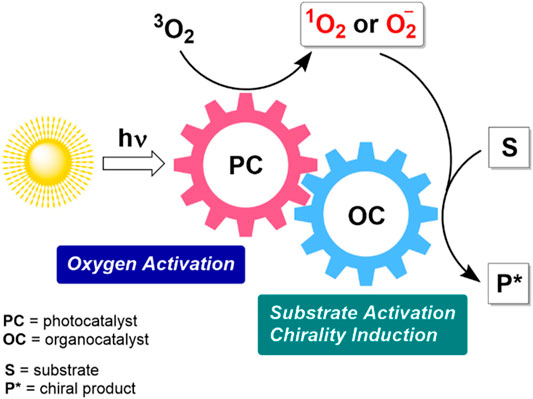
FIGURE 4. Schematic representation of tandem photo-organocatalysis in asymmetric metal-free aerobic oxidations.
The first example of photochemically-driven asymmetric organocatalytic oxidation with singlet oxygen was described by Córdova group, in 2004 (Sundén et al., 2004). They found that singlet oxygen, generated in the presence of tetraphenylporphyrin (TPP) as a photosensitizer, furnished the enantioselective α-oxidation of ketones in the presence of several natural amino acids as organocatalysts. For example, cyclohexanone 65 produced α-hydroxyketone (S)-66 in 93% yield and 56% ee in the presence of L-alanine (20 mol%, Scheme 19A). The key steps of the proposed reaction mechanism imply the intermediate formation of enamine 67, which undergoes the Re-face addition of singlet oxygen producing (2S)-α-hydroperoxide intermediate 68. In the case of a cyclic L-amino acid (e.g. L-proline) the addition of 1O2 occurred to the Si-face of corresponding enamine, thus providing opposite (R)-enantiomer of 66.
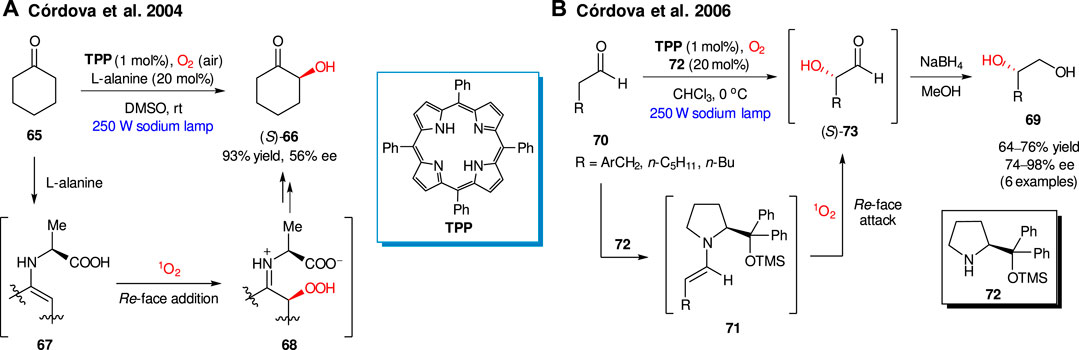
SCHEME 19. Enantioselective α-hydroxylations of carbonyl compounds with singlet oxygen (Sundén et al., 2004; Ibrahem et al., 2006).
As further expansion of the method utility, in 2006 the Córdova group presented the synthesis of 1,2-diols 69 via organocatalytic enantioselective α-oxidation of aldehydes 70 (Scheme 19B) (Ibrahem et al., 2006). The process relies on the generation of enamine species 71, formed in situ from aldehyde 70 and secondary amine organocatalyst 72. The addition of 1O2 occurs from the more sterically accessible Re-face of enamine 71. Reduction of produced α-hydroxy aldehydes 73 with NaBH4 furnished 1,2-diols 69 in 64–76% yields and 74–98% ee.
Works of the Meng group represent a notable recent example of chirality induction in the α-hydroxylation reactions by means of phase transfer catalysis and visible light activation of dioxygen (Lian et al., 2012; Wang et al., 2016; Tang et al., 2018; Tang et al., 2019a; Tang et al., 2019b). The method can be considered as complementary to the Ni-mediated transformation developed by the Xiao group (Scheme 9) (Ding et al., 2017). For example, the aerobic oxidation of ester 74 furnished hydroxylation product 24a in 98% yield and 90% ee in the presence of 2.5 mol% of phase transfer catalyst 75 (Scheme 20) (Wang et al., 2016). The reaction conditions are mild, being applicable for a range of the β-carbonyl compounds (30 examples in total). Furthermore, the synthetic protocol is scalable and suitable for preparation of 24a in gram quantities without the loss of enantioselectivity (Wang et al., 2016).
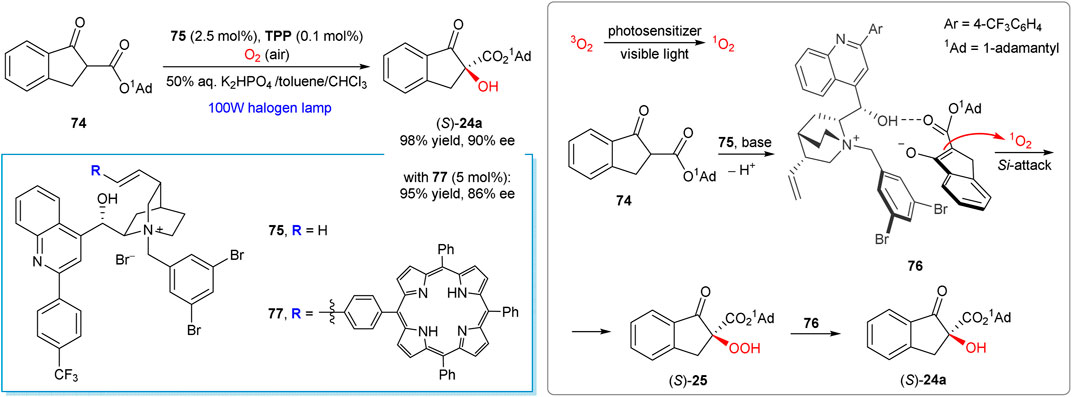
SCHEME 20. Enantioselective α-hydroxylation of β-keto esters with singlet oxygen under chiral phase transfer catalysis (Wang et al., 2016; Tang et al., 2018).
The key step of the suggested reaction mechanism (Scheme 20) implies the addition of singlet oxygen to the chiral enolate-PTC complex 76, in which ion-paring, hydrogen bonding and π-π stacking between PTC and substrate were suggested as key supramolecular interactions (Wang et al., 2016). The produced hydroperoxide intermediate 25 then rapidly reacts with the enolate complex 76 furnishing the final product 24a. The stereochemical outcome of the oxidation could be explained by the Si-face attack of 1O2 or hydroperoxide 25, since the opposite Re-face of enolate in the complex 76 is shielded by 1-adamantyl group.
A bifunctional photo-organocatalyst 77 has also been developed by the same group, with a tetraphenylporphyrin unit grafted to the cinchona alkaloid-derived phase-transfer catalyst (Tang et al., 2018). With the catalyst 77 (5 mol%), visible-light induced aerobic oxidation of 74 afforded (S)-24a in 95% yield and 86% ee. Further methodological improvements, such as greatly reduced reaction times, were achieved by using a flow photomicroreactor technology (Tang et al., 2019a; Tang et al., 2019b).
Besides α-hydroxylation of carbonyl compounds, the asymmetric metal-free oxidations with molecular oxygen can also be applied for the synthesis of other valuable chiral products, although such examples are less common.
In 2013, Shibata group reported a highly enantioselective aerobic epoxidation of β-trifluoromethyl β,β-disubstituted enones in the presence of methylhydrazine and catalytic amounts (5 mol%) of cinchona alkaloid-derived PTC 78 (Scheme 21) (Kawai et al., 2013). The use of methylhydrazine was essential to enable a unique dioxygen activation pathway, in which highly pure hydrogen peroxide was produced in situ by reduction of molecular oxygen. The subsequent asymmetric Weitz-Scheffer reaction of enones 79 with H2O2 afforded chiral epoxide products 80.
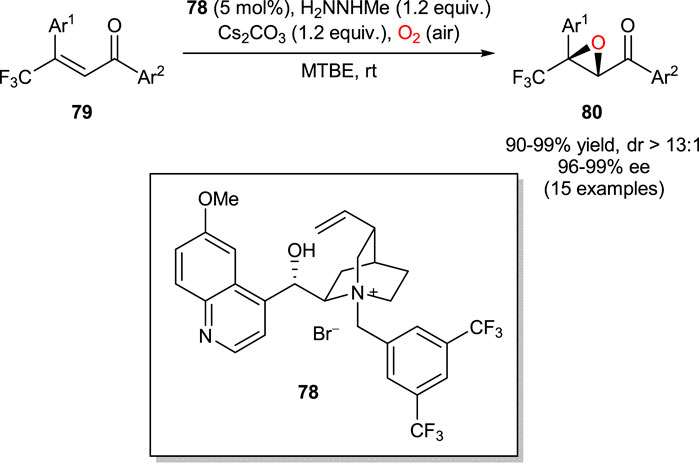
SCHEME 21. Enantioselective aerobic epoxidation of enones 79 (Kawai et al., 2013).
The enantioselective aerobic oxidation of 2-aryl-3-alkylsubstituted indoles 81, accompanied with the semipinacol rearrangement, was reported by Zhao and Jiang in 2018 (Scheme 22) (Bu et al., 2018). The developed cooperative catalytic approach involved organophotoredox 82 and hydrogen bonding 83 catalysts. The preliminary mechanistic studies proved the formation of 3-hydroxylated compound 84 at the first step of aerobic oxidation. It was shown that chiral phosphoric acid 83 provided stereocontrol already at the first step, since the intermediate 84 was formed in its enantiomerically enriched form (59% ee at 65% conversion). However, higher enantioselectivity was observed in the subsequent pinacol rearrangement step (92% ee for 85; Ar = R2 = Ph), indicating that the generated 3-hydroxy intermediate 84 could be engaged at this step and hence affected the enantioselectivity of this rearrangement. The reaction mechanism is apparently similar to that mediated by Ru(bpy)3Cl2 as a photocatalyst, which was thoroughly investigated by Xiao and Lu (Ding et al., 2014) and could involve the reaction between photochemically generated indole cation radical and superoxide radical anion, formed from molecular oxygen. While the generation of singlet oxygen cannot be completely excluded, it is unlikely a dominant pathway.
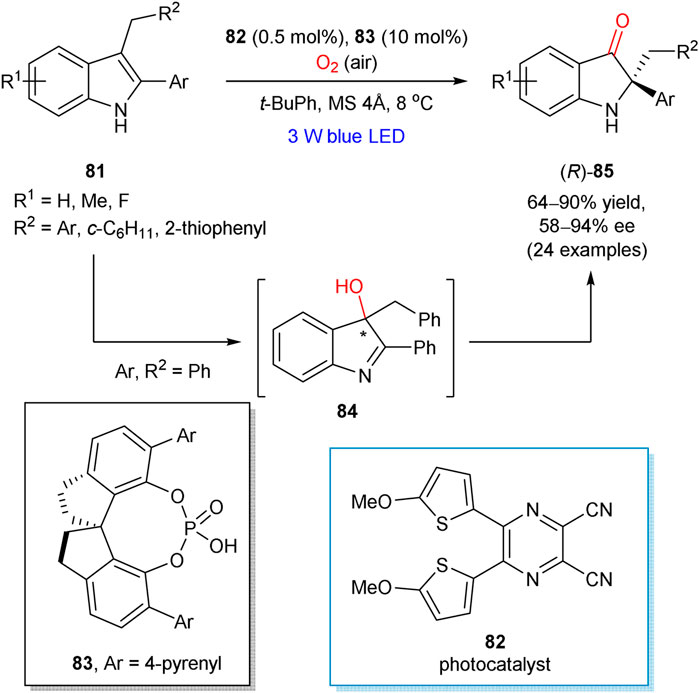
SCHEME 22. Enantioselective aerobic oxidation of 2-aryl-3-alkylsubstituted indoles 81 (Bu et al., 2018).
In 2018, Maity et al. presented an example of kinetic resolution in the aerobic visible-light induced oxidation of isoquinolinium salt 86 (Scheme 23) (Motaleb et al., 2018). The process was mediated by the TADDOL-based phosphite catalyst 87, which led to the oxidation product (S)-88 in 70% ee at 45% conversion (s = 12.3). The proposed reaction mechanism implies the initial formation of an adduct between 86 and organocatalyst 87, which further converts into the organocatalyst-bound α-aminoalkyl radical intermediate. The latter furnishes the oxidation product 88 upon reaction with oxygen or superoxide anion.
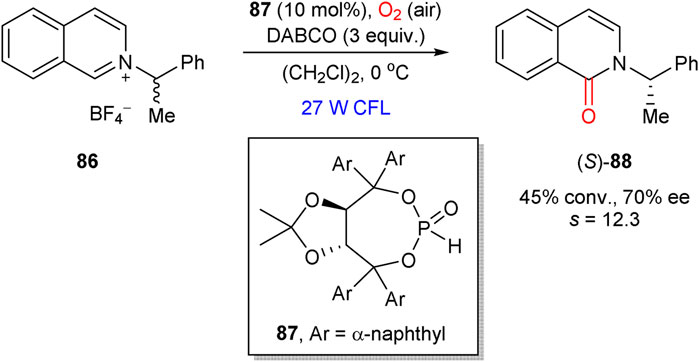
SCHEME 23. Kinetic resolution in aerobic oxidation of isoquinolinium salt 86, mediated by a chiral phosphite catalyst 87 (Motaleb et al., 2018).
Conclusion and Outlook
The progress in enantioselective aerobic oxidations in the last decades clearly demonstrates that molecular oxygen can be considered as a viable oxidant in the asymmetric transformations, being especially attractive for the development of sustainable oxidation protocols. Importantly, an efficient way of chirogenesis must be enabled along with a complementary O2-activation cycle to ensure high yields, extended substrate scope, and excellent optical purity of the oxidation products. In turn, the activation of O2 can be achieved with either transition metal catalysis as a mainstream approach or with the help of photocatalytic methods as another direction. The use of electrosynthesis can also be foreseen (Kawamata et al., 2017; Sambiagio et al., 2017), although it does not have any asymmetric applications yet. Dual catalytic processes have been commonly utilized, as with the aid of custom-designed bifunctional ligands and organocatalysts and with an additional catalytic cycle. Despite of the promising potential of tandem catalytic processes for further application, the design of task-specific catalysts and merging two complementary catalytic cycles into a tandem process are still arduous tasks.
For the forthcoming successful developments in the field, several existing challenges must be properly addressed. Although aerobic oxidations themselves are considered as “green-by-design” processes, their safety and energy efficiency characteristics are often not satisfactory for industrial applications. The use of air and especially pure oxygen in the large-scale manufacturing is hampered by incompatibility with flammable solvents, along with accompanying problems such as heat- and mass transfers in a liquid-gas reaction media (Osterberg et al., 2015; Sterckx et al., 2019). Furthermore, non-catalytic radical autooxidation events may represent an additional obstacle (Sterckx et al., 2019). Fortunately, it is possible to attenuate the impact of these negative phenomena with the aid of modern flow chemistry techiques (Gemoets et al., 2016; Hone and Kappe, 2018). Complications for the industrial use of light-driven reactions arise from their significant running cost and high energy demands (Protti et al., 2009) that eventually results in enhanced CO2 emissions, especially in the case of fossil fuel as a source of energy. This problem can be attenuated and eventually circumvented by further technology advances, such as design of more energy-efficient light sources, photoreactors, and use of clean energy. As for the latter, direct use of sunlight is especially appealing (Haggiage et al., 2009; Cambié et al., 2017; Cambié et al., 2019). Although the concept is more than a century old (Ciamician, 1912), its renaissance has begun only recently.
Besides the technology-related issues outlined above, chemical diversity of the available transformations must be considerably expanded. Despite rapid progress in the field of aerobic oxidations in general, the contribution of the corresponding asymmetric reactions is rather low and mostly limited to the α-hydroxylation of reactive carbonyl compounds, epoxidations of alkenes, and oxidative kinetic resolutions of secondary alcohols. As one of the solutions, an inspiration for the development of new highly chemo- and enantioselective transformations could arise from enzymatic reactions, of which many have no artificial analogs, such as efficient enantioselective hydroxylation of inactivated C–H bonds (Chang et al., 2000), including hydrocarbons (Adam et al., 2000a; Adam et al., 2000b), and cis-dihydroxylation of aromatic compounds (Endoma et al., 2002; García-Urdiales et al., 2005; Boyd and Bugg, 2006).
Albeit the enantioselective oxidation reactions with molecular oxygen are still in their infancy and cannot be considered as dominant among the asymmetric oxidation methods, their sustainable design and prominent potential for environmentally benign chemical industry will certainly stimulate further research activity in the field.
Author Contributions
DK and GE performed a literature search and prepared a draft. DK, GE, ML, and VB wrote the paper. All authors contributed to the article and approved the submitted version.
Funding
Funding from the Centre of Excellence in Molecular Cell Engineering (Grant 2014-2020.4.01.15-0013), the Estonian Ministry of Education and Research (Grant IUT19-32), and Tallinn University of Technology (Grant B58) is gratefully acknowledged.
Conflict of Interest
The authors declare that the research was conducted in the absence of any commercial or financial relationships that could be construed as a potential conflict of interest.
References
Adam, W., Lukacs, Z., Harmsen, D., Saha-Möller, C. R., and Schreier, P. (2000a). Biocatalytic asymmetric hydroxylation of hydrocarbons with the topsoil-microorganism Bacillus megaterium. J. Org. Chem. 65 (3), 878–882. doi:10.1021/jo991725s
Adam, W., Lukacs, Z., Saha-Möller, C. R., Weckerle, B., and Schreier, P. (2000b). Microbial asymmetric CH oxidations of simple hydrocarbons: a novel monooxygenase activity of the topsoil MicroorganismBacillus megaterium. Eur. J. Org. Chem. 2000 (16), 2923–2926. doi:10.1002/1099-0690(200008)2000:16<2923::aid-ejoc2923>3.0.co;2-l
Alamsetti, S. K., and Sekar, G. (2010). Chiral cobalt-catalyzed enantioselective aerobic oxidation of α-hydroxy esters. Chem. Commun. (Camb) 46 (38), 7235–7237. doi:10.1039/c0cc01917h
Allen, S. E., Walvoord, R. R., Padilla-Salinas, R., and Kozlowski, M. C. (2013). Aerobic copper-catalyzed organic reactions. Chem. Rev. 113 (8), 6234–6458. doi:10.1021/cr300527g
Anastas, P. T., and Warner, J. C. (1998). Green chemistry: theory and practice. Oxford, United Kingdom: Oxford University Press.
Berkessel, A., Koch, B., Toniolo, C., Rainaldi, M., Broxterman, Q. B., and Kaptein, B. (2006). Asymmetric enone epoxidation by short solid-phase bound peptides: further evidence for catalyst helicity and catalytic activity of individual peptide strands. Biopolymers 84 (1), 90–96. doi:10.1002/bip.20413
Bolm, C., Khanh Luong, T., and Schlingloff, G. (1997). Enantioselective metal-catalyzed baeyer-villiger oxidation of cyclobutanones. Synlett 1997 (10), 1151–1152. doi:10.1055/s-1997-977
Bolm, C., and Schlingloff, G. (1995). Metal-catalysed enantiospecific aerobic oxidation of cyclobutanones. J. Chem. Soc. Chem. Commun. 12, 1247–1248. doi:10.1039/c39950001247
Bolm, C., Schlingloff, G., and Weickhardt, K. (1994). Optically active lactones from a baeyer-villiger-type metal-catalyzed oxidation with molecular oxygen. Angew. Chem. Int. Ed. Engl. 33 (18), 1848–1849. doi:10.1002/anie.199418481
Borovkov, V. V., Lintuluoto, J. M., and Inoue, Y. (2001). Supramolecular chirogenesis in zinc porphyrins: mechanism, role of guest structure, and application for the absolute configuration determination. J. Am. Chem. Soc. 123 (13), 2979–2989. doi:10.1021/ja0032982
Boyd, D. R., and Bugg, T. D. (2006). Arene cis-dihydrodiol formation: from biology to application. Org. Biomol. Chem. 4 (2), 181–192. doi:10.1039/b513226f
Bryliakov, K. (2015). Environmentally sustainable catalytic asymmetric oxidations. Cleveland, OH: CRC Press.
Bryliakov, K. P. (2017). Catalytic asymmetric oxygenations with the environmentally benign oxidants H2O2 and O2. Chem. Rev. 117 (17), 11406–11459. doi:10.1021/acs.chemrev.7b00167
Bu, L., Li, J., Yin, Y., Qiao, B., Chai, G., Zhao, X., et al. (2018). Organocatalytic asymmetric cascade aerobic oxidation and semipinacol rearrangement reaction: a visible light-induced approach to access chiral 2,2-disubstituted indolin-3-ones. Chem. Asian J. 13 (17), 2382–2387. doi:10.1002/asia.201800446
Bugg, T. D. H. (2003). Dioxygenase enzymes: catalytic mechanisms and chemical models. Tetrahedron 59 (36), 7075–7101. doi:10.1016/s0040-4020(03)00944-x
Cambié, D., Zhao, F., Hessel, V., Debije, M. G., and Noël, T. (2017). A leaf-inspired luminescent solar concentrator for energy-efficient continuous-flow photochemistry. Angew. Chem. Int. Ed. Engl. 56 (4), 1050–1054. doi:10.1002/anie.201611101
Cambié, D., Dobbelaar, J., Riente, P., Vanderspikken, J., Shen, C., Seeberger, P. H., et al. (2019). Energy‐efficient solar photochemistry with luminescent solar concentrator based photomicroreactors. Angew. Chem. Int. Ed. 58 (40), 14374–14378. doi:10.1002/anie.201908553
Caron, S., Dugger, R. W., Ruggeri, S. G., Ragan, J. A., and Ripin, D. H. (2006). Large-scale oxidations in the pharmaceutical industry. Chem. Rev. 106 (7), 2943–2989. doi:10.1021/cr040679f
Caspi, D. D., Ebner, D. C., Bagdanoff, J. T., and Stoltz, B. M. (2004). The resolution of important pharmaceutical building blocks by palladium-catalyzed aerobic oxidation of secondary alcohols. Adv. Synth. Catal. 346 (2–3), 185–189. doi:10.1002/adsc.200303188
Chaiyaveij, D., Cleary, L., Batsanov, A. S., Marder, T. B., Shea, K. J., and Whiting, A. (2011). Copper(II)-catalyzed room temperature aerobic oxidation of hydroxamic acids and hydrazides to acyl-nitroso and azo intermediates, and their Diels-Alder trapping. Org. Lett. 13 (13), 3442–3445. doi:10.1021/ol201188d
Chang, D., Witholt, B., and Li, Z. (2000). Preparation of (S)-N-substituted 4-hydroxy-pyrrolidin-2-ones by regio- and stereoselective hydroxylation with sphingomonas sp. HXN-200. Org. Lett. 2 (24), 3949–3952. doi:10.1021/ol006735q
Chen, C.-T., Bettigeri, S., Weng, S.-S., Pawar, V. D., Lin, Y.-H., Liu, C.-Y., et al. (2007). Asymmetric aerobic oxidation of α-hydroxy acid derivatives byC4-symmetric, vanadate-centered, tetrakisvanadyl(V) clusters derived fromN-Salicylidene-α-aminocarboxylates. J. Org. Chem. 72 (22), 8175–8185. doi:10.1021/jo070575f
Chen, C. T., Kao, J. Q., Salunke, S. B., and Lin, Y. H. (2011). Enantioselective aerobic oxidation of α-hydroxy-ketones catalyzed by oxidovanadium(V) methoxides bearing chiral, N-salicylidene-tert-butylglycinates. Org. Lett. 13 (1), 26–29. doi:10.1021/ol1024053
Ciamician, G. (1912). The photochemistry of the future. Science 36 (926), 385–394. doi:10.1126/science.36.926.385
de Gonzalo, G., and Fraaije, M. W. (2013). Recent developments in flavin-based catalysis. ChemCatChem 5 (2), 403–415. doi:10.1002/cctc.201200466
de Vries, E. F. J., Ploeg, L., Colao, M., Brussee, J., and van der Gen, A. (1995). Enantioselective oxidation of aromatic ketones by molecular oxygen, catalyzed by chiral monoaza-crown ethers. Tetrahedron: Asymmetry 6 (5), 1123–1132. doi:10.1016/0957-4166(95)00138-f
Ding, W., Lu, L. Q., Zhou, Q. Q., Wei, Y., Chen, J. R., and Xiao, W. J. (2017). Bifunctional photocatalysts for enantioselective aerobic oxidation of β-ketoesters. J. Am. Chem. Soc. 139 (1), 63–66. doi:10.1021/jacs.6b11418
Ding, W., Zhou, Q.-Q., Xuan, J., Li, T.-R., Lu, L.-Q., and Xiao, W.-J. (2014). Photocatalytic aerobic oxidation/semipinacol rearrangement sequence: a concise route to the core of pseudoindoxyl alkaloids. Tetrahedron Lett. 55 (33), 4648–4652. doi:10.1016/j.tetlet.2014.06.102
Dismukes, G. C. (1996). Manganese enzymes with binuclear active sites. Chem. Rev. 96 (7), 2909–2926. doi:10.1021/cr950053c
Doering, W., and Haines, R. M. (1954). Alkoxide-catalyzed autoxidative cleavage of ketones and Esters1. J. Am. Chem. Soc. 76 (2), 482–486. doi:10.1021/ja01631a044
Egami, H., and Katsuki, T. (2009). Iron-catalyzed asymmetric aerobic oxidation: oxidative coupling of 2-naphthols. J. Am. Chem. Soc. 131 (17), 6082–6083. doi:10.1021/ja901391u
Elek, G. Z., Borovkov, V., Lopp, M., and Kananovich, D. G. (2017). Enantioselective one-pot synthesis of α,β-epoxy ketones via aerobic oxidation of cyclopropanols. Org. Lett. 19 (13), 3544–3547. doi:10.1021/acs.orglett.7b01519
Elek, G. Z., Koppel, K., Zubrytski, D. M., Konrad, N., Järving, I., Lopp, M., et al. (2019). Divergent access to histone deacetylase inhibitory cyclopeptides via a late-stage cyclopropane ring cleavage strategy. Org. Lett. 21 (20), 8473–8478. doi:10.1021/acs.orglett.9b03305
Endoma, M. A., Bui, V. P., Hansen, J., and Hudlicky, T. (2002). Medium-scale preparation of useful metabolites of aromatic compounds via whole-cell fermentation with recombinant organisms. Org. Process. Res. Dev. 6 (4), 525–532. doi:10.1021/op020013s
Ferreira, E. M., and Stoltz, B. M. (2001). The palladium-catalyzed oxidative kinetic resolution of secondary alcohols with molecular oxygen. J. Am. Chem. Soc. 123 (31), 7725–7726. doi:10.1021/ja015791z
Festa, R. A., and Thiele, D. J. (2011). Copper: an essential metal in biology. Curr. Biol. 21 (21), R877–R883. doi:10.1016/j.cub.2011.09.040
Frazier, C. P., Bugarin, A., Engelking, J. R., and Read de Alaniz, J. (2012). Copper-catalyzed aerobic oxidation of N-substituted hydroxylamines: efficient and practical access to nitroso compounds. Org. Lett. 14 (14), 3620–3623. doi:10.1021/ol301414k
Frazier, C. P., Engelking, J. R., and Read de Alaniz, J. (2011). Copper-catalyzed aerobic oxidation of hydroxamic acids leads to a mild and versatile acylnitroso ene reaction. J. Am. Chem. Soc. 133 (27), 10430–10433. doi:10.1021/ja204603u
Gandomkar, S., Jost, E., Loidolt, D., Swoboda, A., Pickl, M., Elaily, W., et al. (2019). Biocatalytic enantioselective oxidation of sec-allylic alcohols with flavin-dependent oxidases. Adv. Synth. Catal. 361 (22), 5264–5271. doi:10.1002/adsc.201900921
García-Urdiales, E., Alfonso, I., and Gotor, V. (2005). Enantioselective enzymatic desymmetrizations in organic synthesis. Chem. Rev. 105 (1), 313–354. doi:10.1021/cr040640a
Gardner, J. N., Carlon, F. E., and Gnoj, O. (1968). A one-step procedure for the preparation of tertiary alpha-ketols from the corresponding ketones. J. Org. Chem. 33 (8), 3294–3297. doi:10.1021/jo01272a055
Gebauer, K., Reuß, F., Spanka, M., and Schneider, C. (2017). Relay catalysis: manganese(III) phosphate catalyzed asymmetric addition of β-dicarbonyls to ortho-quinone methides generated by catalytic aerobic oxidation. Org. Lett. 19 (17), 4588–4591. doi:10.1021/acs.orglett.7b02185
Gemoets, H. P., Su, Y., Shang, M., Hessel, V., Luque, R., and Noël, T. (2016). Liquid phase oxidation chemistry in continuous-flow microreactors. Chem. Soc. Rev. 45 (1), 83–117. doi:10.1039/c5cs00447k
Germain, A. R., Bruggemeyer, D. M., Zhu, J., Genet, C., O’Brien, P., and Porco, J. A. (2011). Synthesis of the azaphilones (+)-Sclerotiorin and (+)-8-O-methylsclerotiorinamine utilizing (+)-Sparteine surrogates in copper-mediated oxidative dearomatization. J. Org. Chem. 76 (8), 2577–2584. doi:10.1021/jo102448n
Ghogare, A. A., and Greer, A. (2016). Using singlet oxygen to synthesize natural products and drugs. Chem. Rev. 116 (17), 9994–10034. doi:10.1021/acs.chemrev.5b00726
Hadian-Dehkordi, L., and Hosseini-Monfared, H. (2016). Enantioselective aerobic oxidation of olefins by magnetite nanoparticles at room temperature: a chiral carboxylic acid strategy. Green. Chem. 18 (2), 497–507. doi:10.1039/c5gc01774b
Haggiage, E., Coyle, E. E., Joyce, K., and Oelgemöller, M. (2009). Green photochemistry: solarchemical synthesis of 5-amido-1,4-naphthoquinones. Green. Chem. 11 (3), 318–321. doi:10.1039/b816676e
Hone, C. A., and Kappe, C. O. (2018). The use of molecular oxygen for liquid phase Aerobic oxidations in continuous flow. Top. Curr. Chem. (Cham) 377 (1), 2. doi:10.1007/s41061-018-0226-z
Hone, C. A., Roberge, D. M., and Kappe, C. O. (2017). The use of molecular oxygen in pharmaceutical manufacturing: is flow the way to go? ChemSusChem 10 (1), 32–41. doi:10.1002/cssc.201601321
Ibrahem, I., Zhao, G.-L., Sundén, H., and Córdova, A. (2006). A route to 1,2-diols by enantioselective organocatalytic α-oxidation with molecular oxygen. Tetrahedron Lett. 47 (27), 4659–4663. doi:10.1016/j.tetlet.2006.04.133
Imagawa, K., Nagata, T., Yamada, T., and Mukaiyama, T. (1995). Asymmetric oxidation of sulfides with molecular oxygen catalyzed byβ-oxo aldiminato manganese(III) complexes. Chem. Lett. 24 (5), 335–336. doi:10.1246/cl.1995.335
Ingold, K. U. (1961). Inhibition of the autoxidation of organic substances in the liquid phase. Chem. Rev. 61 (6), 563–589. doi:10.1021/cr60214a002
Irie, R., and Katsuki, T. (2004). Selective aerobic oxidation of hydroxy compounds catalyzed by photoactivated ruthenium-salen complexes (selective catalytic aerobic oxidation). Chem. Rec. 4 (2), 96–109. doi:10.1002/tcr.20001
Irie, R., Masutani, K., and Katsuki, T. (2000). Asymmetric aerobic oxidation coupling of 2-naphthol derivatives catalyzed by photo-activated chiral (NO)Ru(II)-Salen complex. Synlett 2000 (10), 1433–1436. doi:10.1055/s-2000-7654
Jacobsen, E. N., Marko, I., Mungall, W. S., Schroeder, G., and Sharpless, K. B. (1988). Asymmetric dihydroxylation via ligand-accelerated catalysis. J. Am. Chem. Soc. 110 (6), 1968–1970. doi:10.1021/ja00214a053
Jensen, D. R., Pugsley, J. S., and Sigman, M. S. (2001). Palladium-catalyzed enantioselective oxidations of alcohols using molecular oxygen. J. Am. Chem. Soc. 123 (30), 7475–7476. doi:10.1021/ja015827n
Katsuki, T., and Martin, V. (2004). Asymmetric epoxidation of allylic alcohols: the katsuki–sharpless epoxidation reaction. Org. React. 48, 1–299. doi:10.1002/0471264180.or048.01
Katsuki, T., and Sharpless, K. B. (1980). The first practical method for asymmetric epoxidation. J. Am. Chem. Soc. 102 (18), 5974–5976. doi:10.1021/ja00538a077
Kawai, H., Okusu, S., Yuan, Z., Tokunaga, E., Yamano, A., Shiro, M., et al. (2013). Enantioselective synthesis of epoxides having a tetrasubstituted trifluoromethylated carbon center: methylhydrazine-induced aerobic epoxidation of β,β-disubstituted enones. Angew. Chem. Int. Ed. Engl. 52 (8), 2221–2225. doi:10.1002/anie.201209355
Kawamata, Y., Yan, M., Liu, Z., Bao, D. H., Chen, J., Starr, J. T., et al. (2017). Scalable, electrochemical oxidation of unactivated C-H bonds. J. Am. Chem. Soc. 139 (22), 7448–7451. doi:10.1021/jacs.7b03539
Kelly, D. R., and Roberts, S. M. (2004). The mechanism of polyleucine catalysed asymmetric epoxidation. Chem. Commun. 18, 2018–2020. doi:10.1039/B404390C
Konen, D. A., Silbert, L. S., and Pfeffer, P. E. (1975). .alpha.-Anions. VII. Direct oxidation of enolate anions to 2-hydroperoxy- and 2-hydroxycarboxylic acids and esters. J. Org. Chem. 40 (22), 3253–3258. doi:10.1021/jo00910a020
Koya, S., Nishioka, Y., Mizoguchi, H., Uchida, T., and Katsuki, T. (2012). Asymmetric epoxidation of conjugated olefins with dioxygen. Angew. Chem. Int. Ed. Engl. 51 (33), 8243–8246. doi:10.1002/anie.201201848
Krishnan, S., Bagdanoff, J. T., Ebner, D. C., Ramtohul, Y. K., Tambar, U. K., and Stoltz, B. M. (2008). Pd-catalyzed enantioselective aerobic oxidation of secondary alcohols: applications to the total synthesis of alkaloids. J. Am. Chem. Soc. 130 (41), 13745–13754. doi:10.1021/ja804738b
Kumar, G., Verma, S., Ansari, A., Khan, N.-u. H., and Kureshy, R. I. (2017). Enantioselective cross dehydrogenative coupling reaction catalyzed by Rose bengal incorporated-Cu(I)-Dimeric chiral complexes. Catal. Commun. 99, 94–99. doi:10.1016/j.catcom.2017.05.026
Kureshy, R. I., Khan, N. H., Abdi, S. H. R., Iyer, P., and Bhatt, A. K. (1998). Aerobic, enantioselective epoxidation of non-functionalized olefins catalyzed by Ni(II) chiral schiff base complexes. J. Mol. Catal. A Chem. 130 (1), 41–50. doi:10.1016/s1381-1169(97)00195-7
Kureshy, R. I., Khan, N. H., Abdi, S. H. R., and Iyer, P. (1997). Chiral Ru(III) metal complex-catalyzed aerobic enantioselective epoxidation of styrene derivatives with Co-oxidation of aldehyde. J. Mol. Catal. A Chem. 124 (2), 91–97. doi:10.1016/s1381-1169(97)00076-9
Lai, T.-S., Zhang, R., Cheung, K.-K., Che, C.-M., Lai, T.-S., and Kwong, H.-L. (1998). Aerobic enantioselective alkene epoxidation by a chiral trans-dioxo(D4-Porphyrinato)Ruthenium(VI) complex. Chem. Commun. 15, 1583–1584. doi:10.1039/a802009d
Lian, M., Li, Z., Cai, Y., Meng, Q., and Gao, Z. (2012). Enantioselective photooxygenation of β-keto esters by chiral phase-transfer catalysis using molecular oxygen. Chem. Asian J. 7 (9), 2019–2023. doi:10.1002/asia.201200358
Maji, B., and Yamamoto, H. (2015). Asymmetric synthesis of tertiary α-hydroxy phosphonic acid derivatives under aerobic oxidation conditions. Synlett 26 (11), 1528–1532. doi:10.1055/s-0034-1380290
Maji, B., and Yamamoto, H. (2014). Copper-catalyzed asymmetric synthesis of tertiary α-hydroxy phosphonic acid derivatives with in situ generated nitrosocarbonyl compounds as the oxygen source. Angew Chem. Int. Ed. Engl. 53 (52), 14472–14475. doi:10.1002/anie.201408893
Masui, M., Ando, A., and Shioiri, T. (1988). New methods and reagents in organic synthesis. 75. asymmetric synthesis of α-hydroxy ketones using chiral phase transfer catalysts. Tetrahedron Lett. 29 (23), 2835–2838. doi:10.1016/0040-4039(88)85224-9
Masutani, K., Irie, R., and Katsuki, T. (2002). Asymmetric CyclizationviaOxygen cation radical: enantioselective synthesis ofcis-4b,9b-Dihydrobenzofuro[3,2-b]benzofurans. Chem. Lett. 31 (1), 36–37. doi:10.1246/cl.2002.36
Masutani, K., Uchida, T., Irie, R., and Katsuki, T. (2000). Catalytic asymmetric and chemoselective aerobic oxidation: kinetic resolution of sec-alcohols. Tetrahedron Lett. 41 (26), 5119–5123. doi:10.1016/s0040-4039(00)00787-5
Matsumoto, K., Egami, H., Oguma, T., and Katsuki, T. (2012). What factors influence the catalytic activity of iron-salan complexes for aerobic oxidative coupling of 2-naphthols? Chem. Commun. 48 (47), 5823–5825. doi:10.1039/c2cc18090a
McCann, S. D., and Stahl, S. S. (2015). Copper-catalyzed aerobic oxidations of organic molecules: pathways for two-electron oxidation with a four-electron oxidant and a one-electron redox-active catalyst. Acc. Chem. Res. 48 (6), 1756–1766. doi:10.1021/acs.accounts.5b00060
Meunier, B., de Visser, S. P., and Shaik, S. (2004). Mechanism of oxidation reactions catalyzed by cytochrome P450 enzymes. Chem. Rev. 104 (9), 3947–3980. doi:10.1021/cr020443g
Miller, D. M., Buettner, G. R., and Aust, S. D. (1990). Transition metals as catalysts of “autoxidation” reactions. Free Radic. Biol. Med. 8 (1), 95–108. doi:10.1016/0891-5849(90)90148-c
Miyamura, H., Choo, G. C., Yasukawa, T., Yoo, W. J., and Kobayashi, S. (2013). A heterogeneous layered bifunctional catalyst for the integration of aerobic oxidation and asymmetric C-C bond formation. Chem. Commun. (Camb) 49 (85), 9917–9919. doi:10.1039/c3cc46204h
Miyamura, H., Suzuki, A., Yasukawa, T., and Kobayashi, S. (2015). Integrated process of aerobic oxidation-olefination-asymmetric C-C bond formation catalyzed by robust heterogeneous gold/palladium and chirally modified rhodium Nanoparticles. Adv. Synth. Catal. 357 (18), 3815–3819. doi:10.1002/adsc.201500529
Mizoguchi, H., Uchida, T., and Katsuki, T. (2014). Ruthenium-catalyzed oxidative kinetic resolution of unactivated and activated secondary alcohols with air as the hydrogen acceptor at room temperature. Angew. Chem. Int. Ed. Engl. 53 (12), 3178–3182. doi:10.1002/anie.201310426
Motaleb, A., Bera, A., and Maity, P. (2018). An organocatalyst bound α-aminoalkyl radical intermediate for controlled aerobic oxidation of iminium ions. Org. Biomol. Chem. 16 (28), 5081–5085. doi:10.1039/c8ob01032c
Mukaiyama, T., Yamada, T., Nagata, T., and Imagawa, K. (1993). Asymmetric aerobic epoxidation of unfunctionalized olefins catalyzed by optically active α-Alkoxycarbonyl-β-ketoiminato manganese(III) complexes. Chem. Lett. 22 (2), 327–330. doi:10.1246/cl.1993.327
Mukaiyama, T., and Yamada, T. (1995). Recent advances in aerobic oxygenation. Bull. Chem. Soc. Jpn. 68 (1), 17–35. doi:10.1246/bcsj.68.17
Muthupandi, P., and Sekar, G. (2011). Zinc-catalyzed aerobic oxidation of benzoins and its extension to enantioselective oxidation. Tetrahedron Lett. 52 (6), 692–695. doi:10.1016/j.tetlet.2010.12.004
Nagata, T., Imagawa, K., Yamada, T., and Mukaiyama, T. (1994). Enantioselective aerobic epoxidation of acyclic simple olefins catalyzed by the optically active β-ketoiminato manganese(III) complex. Chem. Lett. 23 (7), 1259–1262. doi:10.1246/cl.1994.1259
Nagata, T., Imagawa, K., Yamada, T., and Mukaiyama, T. (1995). Enantioselective aerobic oxidation of sulfides catalyzed by optically activeβ-oxo aldiminatomanganese(III) complexes. Bull. Chem. Soc. Jpn. 68 (11), 3241–3246. doi:10.1246/bcsj.68.3241
Nechab, M., Einhorn, C., and Einhorn, J. (2004). New aerobic oxidation of benzylic compounds: efficient catalysis by N-Hydroxy-3,4,5,6-Tetraphenylphthalimide (NHTPPI)/CuCl under mild conditions and low catalyst loading. Chem. Commun. 13, 1500–1501. doi:10.1039/b403004d
Nechab, M., Kumar, D. N., Philouze, C., Einhorn, C., and Einhorn, J. (2007). Variable C2-symmetric analogues of N-hydroxyphthalimide as enantioselective catalysts for aerobic oxidation: kinetic resolution of oxazolidines. Angew. Chem. Int. Ed. Engl. 46 (17), 3080–3083. doi:10.1002/anie.200603780
Osterberg, P. M., Niemeier, J. K., Welch, C. J., Hawkins, J. M., Martinelli, J. R., Johnson, T. E., et al. (2015). Experimental limiting oxygen concentrations for nine organic solvents at temperatures and pressures relevant to aerobic oxidations in the pharmaceutical industry. Org. Process. Res. Dev. 19 (11), 1537–1543. doi:10.1021/op500328f
Ottenbacher, R. V., Talsi, E. P., and Bryliakov, K. P. (2018). Catalytic asymmetric oxidations using molecular oxygen. Russ. Chem. Rev. 87 (9), 821. doi:10.1070/rcr4825
Parmeggiani, C., and Cardona, F. (2012). Transition metal based catalysts in the aerobic oxidation of alcohols. Green. Chem. 14 (3), 547–564. doi:10.1039/c2gc16344f
Petsi, M., and Zografos, A. L. (2018). Advances in catalytic aerobic oxidations by activation of dioxygen-monooxygenase enzymes and biomimetics. Synthesis 50 (24), 4715–4745. doi:10.1055/s-0037-1610297
Poon, J. F., and Pratt, D. A. (2018). Recent insights on hydrogen atom transfer in the inhibition of hydrocarbon autoxidation. Acc. Chem. Res. 51 (9), 1996–2005. doi:10.1021/acs.accounts.8b00251
Porter, N. A. (2013). A perspective on free radical autoxidation: the physical organic chemistry of polyunsaturated fatty acid and sterol peroxidation. J. Org. Chem. 78 (8), 3511–3524. doi:10.1021/jo4001433
Potowski, M., Merten, C., Antonchick, A. P., and Waldmann, H. (2015). Catalytic aerobic oxidation and tandem enantioselective cycloaddition in cascade multicomponent synthesis. Chemistry 21 (13), 4913–4917. doi:10.1002/chem.201500125
Protti, S., Dondi, D., Fagnoni, M., and Albini, A. (2009). Assessing photochemistry as a green synthetic method. Carbon-carbon bond forming reactions. Green. Chem. 11 (2), 239–249. doi:10.1039/b810594d
Qian, W. J., Wei, W. G., Zhang, Y. X., and Yao, Z. J. (2007). Total synthesis, assignment of absolute stereochemistry, and structural revision of chlorofusin. J. Am. Chem. Soc. 129 (20), 6400–6401. doi:10.1021/ja072225g
Romero, N. A., and Nicewicz, D. A. (2016). Organic photoredox catalysis. Chem. Rev. 116 (17), 10075–10166. doi:10.1021/acs.chemrev.6b00057
Salunke, S. B., Babu, N. S., and Chen, C.-T. (2011). Asymmetric aerobic oxidation of α-hydroxy acid derivatives catalyzed by reusable, polystyrene-supported chiral N-salicylidene oxidovanadium tert-leucinates. Adv. Synth. Catal. 353 (8), 1234–1240. doi:10.1002/adsc.201100062
Sambiagio, C., Sterckx, H., and Maes, B. U. W. (2017). Electrosynthesis: a new frontier in aerobic oxidation? ACS Cent. Sci. 3 (7), 686–688. doi:10.1021/acscentsci.7b00275
Sandoval, D., Samoshin, A. V., and Read de Alaniz, J. (2015). Asymmetric electrophilic α-amination of silyl enol ether derivatives via the nitrosocarbonyl hetero-ene reaction. Org. Lett. 17 (18), 4514–4517. doi:10.1021/acs.orglett.5b02208
Sano, D., Nagata, K., and Itoh, T. (2008). Catalytic asymmetric hydroxylation of oxindoles by molecular oxygen using a phase-transfer catalyst. Org. Lett. 10 (8), 1593–1595. doi:10.1021/ol800260r
Shimizu, H., Onitsuka, S., Egami, H., and Katsuki, T. (2005). Ruthenium(Salen)-Catalyzed aerobic oxidative desymmetrization of meso-diols and its kinetics. J. Am. Chem. Soc. 127 (15), 5396–5413. doi:10.1021/ja047608i
Shimizu, H., and Katsuki, T. (2003). (Salen)ruthenium-catalyzed desymmetrization ofmeso-diols (2). Apical ligand effect on enantioselectivity. Chem. Lett. 32 (6), 480–481. doi:10.1246/cl.2003.480
Shimizu, H., Nakata, K., and Katsuki, T. (2002). (Salen)ruthenium-Catalyzed desymmetrization ofMeso-diols: catalytic aerobic asymmetric oxidation under photo-irradiation. Chem. Lett. 31 (11), 1080–1081. doi:10.1246/cl.2002.1080
Sim, S.-B. D., Wang, M., and Zhao, Y. (2015). Phase-transfer-catalyzed enantioselective α-hydroxylation of acyclic and cyclic ketones with oxygen. ACS Catal. 5 (6), 3609–3612. doi:10.1021/acscatal.5b00583
Stahl, S. S. (2004). Palladium oxidase catalysis: selective oxidation of organic chemicals by direct dioxygen-coupled turnover. Angew Chem Int Ed Engl. 43 (26), 3400–3420. doi:10.1002/anie.200300630
Sterckx, H., Morel, B., and Maes, B. U. W. (2019). Catalytic aerobic oxidation of C (sp3)−H bonds. Angew. Chem. Int. Ed. 58 (24), 7946–7970. doi:10.1002/anie.201804946
Sundén, H., Engqvist, M., Casas, J., Ibrahem, I., and Córdova, A. (2004). Direct amino acid catalyzed asymmetric α oxidation of ketones with molecular oxygen. Angew. Chem. Int. Edition 43 (47), 6532–6535. doi:10.1002/anie.200460295
Tanaka, H., Nishikawa, H., Uchida, T., and Katsuki, T. (2010). Photopromoted Ru-catalyzed asymmetric aerobic sulfide oxidation and epoxidation using water as a proton transfer mediator. J. Am. Chem. Soc. 132 (34), 12034–12041. doi:10.1021/ja104184r
Tang, X.-F., Feng, S.-H., Wang, Y.-K., Yang, F., Zheng, Z.-H., Zhao, J.-N., et al. (2018). Bifunctional metal-free photo-organocatalysts for enantioselective aerobic oxidation of β-dicarbonyl compounds. Tetrahedron 74 (27), 3624–3633. doi:10.1016/j.tet.2018.05.023
Tang, X. F., Zhao, J. N., Wu, Y. F., Zheng, Z. H., Feng, S. H., Yu, Z. Y., et al. (2019a). Enantioselective photooxygenation of β-dicarbonyl compounds in batch and flow photomicroreactors. Org. Biomol. Chem. 17 (34), 7938–7942. doi:10.1039/c9ob01379b
Tang, X. F., Zhao, J. N., Wu, Y. F., Feng, S. H., Yang, F., Yu, Z. Y., et al. (2019b). Visible‐light‐driven enantioselective aerobic oxidation of β‐dicarbonyl compounds catalyzed by cinchona‐derived phase transfer catalysts in batch and semi‐flow. Adv. Synth. Catal. 361 (22), 5245–5252. doi:10.1002/adsc.201900777
Tu, Y., Wang, Z.-X., and Shi, Y. (1996). An efficient asymmetric epoxidation method fortrans-olefins mediated by a fructose-derived ketone. J. Am. Chem. Soc. 118 (40), 9806–9807. doi:10.1021/ja962345g
Uchida, T., and Katsuki, T. (2013). Green asymmetric oxidation using air as oxidant. J. Synth. Org. Chem. Jpn. 71 (11), 1126–1135. doi:10.5059/yukigoseikyokaishi.71.1126
Wang, Q., Chen, J., and Huang, Y. (2018). Aerobic oxidation/annulation cascades through synergistic catalysis of RuCl3 and N-heterocyclic carbenes. Chem. Eur. J. 24 (49), 12806–12810. doi:10.1002/chem.201803254
Wang, Y., Zheng, Z., Lian, M., Yin, H., Zhao, J., Meng, Q., et al. (2016). Photo-organocatalytic enantioselective α-hydroxylation of β-keto esters and β-keto amides with oxygen under phase transfer catalysis. Green. Chem. 18 (20), 5493–5499. doi:10.1039/c6gc01245k
Weng, S. S., Shen, M. W., Kao, J. Q., Munot, Y. S., and Chen, C. T. (2006). Chiral N-salicylidene vanadyl carboxylate-catalyzed enantioselective aerobic oxidation of alpha-hydroxy esters and amides. Proc. Natl. Acad. Sci. USA 103 (10), 3522–3527. doi:10.1073/pnas.0511021103
Wertz, S., and Studer, A. (2013). Nitroxide-catalyzed transition-metal-free aerobic oxidation processes. Green. Chem. 15 (11), 3116–3134. doi:10.1039/c3gc41459k
Xu, C., Zhang, L., and Luo, S. (2014). Merging aerobic oxidation and enamine catalysis in the asymmetric α-amination of β-ketocarbonyls UsingN-hydroxycarbamates as nitrogen sources. Angew Chem. Int. Ed. Engl. 53 (16), 4149–4153. doi:10.1002/anie.201400776
Yamada, T., Imagawa, K., Nagata, T., and Mukaiyama, T. (1994). Aerobic enantioselective epoxidation of unfunctionalized olefins catalyzed by optically active salen-manganese(III) complexes. Bull. Chem. Soc. Jpn. 67 (8), 2248–2256. doi:10.1246/bcsj.67.2248
Yamada, T., Imagawa, K., Nagata, T., and Mukaiyama, T. (1992). Enantioselective epoxidation of unfunctionalized olefins with molecular oxygen and aldehyde catalyzed by optically active manganese(III) complexes. Chem. Lett. 21 (11), 2231–2234. doi:10.1246/cl.1992.2231
Yang, Y., Moinodeen, F., Chin, W., Ma, T., Jiang, Z., and Tan, C. H. (2012). Pentanidium-catalyzed enantioselective α-hydroxylation of oxindoles using molecular oxygen. Org. Lett. 14 (18), 4762–4765. doi:10.1021/ol302030v
Zamadar, M., and Greer, A. (2009). Singlet oxygen as a reagent in organic synthesis. Handbook Synth. Photochemistry 16, 353–386. doi:10.1002/9783527628193.ch11
Zhang, W., Loebach, J. L., Wilson, S. R., and Jacobsen, E. N. (1990). Enantioselective epoxidation of unfunctionalized olefins catalyzed by salen manganese complexes. J. Am. Chem. Soc. 112 (7), 2801–2803. doi:10.1021/ja00163a052
Zhu, J., Grigoriadis, N. P., Lee, J. P., and Porco, J. A. (2005). Synthesis of the azaphilones using copper-mediated enantioselective oxidative dearomatization. J. Am. Chem. Soc. 127 (26), 9342–9343. doi:10.1021/ja052049g
Keywords: oxygen, aerobic oxidation, asymmetric synthesis, enantioselective catalysis, chirality, organocatalysis, transition metals
Citation: Kananovich D, Elek GZ, Lopp M and Borovkov V (2021) Aerobic Oxidations in Asymmetric Synthesis: Catalytic Strategies and Recent Developments. Front. Chem. 9:614944. doi: 10.3389/fchem.2021.614944
Received: 07 October 2020; Accepted: 10 February 2021;
Published: 30 March 2021.
Edited by:
Hiroyuki Miyake, Osaka City University, JapanReviewed by:
Mainak Banerjee, Birla Institute of Technology and Science, IndiaHideki Sugimoto, Osaka University, Japan
Copyright © 2021 Kananovich, Elek, Lopp and Borovkov. This is an open-access article distributed under the terms of the Creative Commons Attribution License (CC BY). The use, distribution or reproduction in other forums is permitted, provided the original author(s) and the copyright owner(s) are credited and that the original publication in this journal is cited, in accordance with accepted academic practice. No use, distribution or reproduction is permitted which does not comply with these terms.
*Correspondence: Dzmitry Kananovich, dzmitry.kananovich@taltech.ee; Victor Borovkov, victor.borovkov@taltech.ee