- 1Key Laboratory of Molecular Target and Clinical Pharmacology, State Key Laboratory of Respiratory Disease, School of Pharmaceutical Science and Fifth Affiliated Hospital, Guangzhou Medical University, Guangzhou, China
- 2Department of Biomedical Engineering, School of Basic Medical Sciences, Guangzhou Medical University, Guangzhou, China
- 3School of Nursing, Tung Wah College, Hung Hom, Hong Kong, SAR China
Photodynamic therapy (PDT) is a mini-invasive therapy on malignancies via reactive oxygen species (ROS) induced by photosenitizer (PS) upon light irradiation. However, poor target of PS to tumor limits the clinical application of PDT. Compared with normal tissues, tumor tissues have a unique enzymatic environment. The unique enzymatic environment in tumor tissues has been widely used as a target for developing smart materials to improve the targetability of drugs to tumor. Enzyme-responsive materials (ERM) as a smart material can respond to the enzymes in tumor tissues to specifically deliver drugs. In PDT, ERM was designed to react with the enzymes highly expressed in tumor tissues to deliver PS in the target site to prevent therapeutic effects and avoid its side-effects. In the present paper, we will review the application of ERM in PDT and discuss the challenges of ERM as carriers to deliver PS for further boosting the development of PDT in the management of malignancies.
Introduction
Cancer is one of the most threatening diseases to humans (Zhang et al., 2019). Chemotherapy is one of the main treatments in the management of malignancies, but chemotherapy induced side effects have severely affected the therapeutic outcome due to non-target accumulation and drug resistance (Xing et al., 2019; Jiang et al., 2020). In particular, the systemic toxicity of conventional chemical drugs significantly limited the development of chemotherapy (Li et al., 2021). Photodynamic therapy (PDT) is being paid wide attention as a non-invasive, low-toxicity, and high-efficient treatment approach (Chi Zhang et al., 2019; Izumoto et al., 2020). As early as thousands of years ago, the ancients used herbs and sunlight together, but they did not cause concern (Bown, 2013; Zhao and He, 2014). Until the beginning of the twentieth century, scientists discovered that exposing paramecium that swallowed photosensitizer (PS) to visible light caused death (Dolmans et al., 2003; Diogo et al., 2015; Mansoori et al., 2019). PDT is based on the production of a large amount of ROS to kill tumor cells through light-activated PS (Fan et al., 2016; Wu et al., 2019; Zhou et al., 2020). As Figure 1A shown, while the PS is exposed to light with a specific wavelength, the outermost electron in the molecular orbital transitions from the ground state S0 to the excited singlet state (Ackroyd et al., 2001). Then, intersystem crossing or spin reversal occurs, and the molecule transforms into an excited triplet state with a longer lifetime (Mansoori et al., 2019). These two unstable states of PS lose energy through the emission of fluorescence or phosphorescence and the internal conversion into heat (Muniyandi et al., 2020). The PS in the excited triplet state can produce photochemical reactions, including type I reaction or type II reaction. The excited PS reacts with the molecules (including oxygen) in the surrounding environment through the electron transfer process, resulting in free radicals production (Schalch et al., 2019). These free radicals produce toxic effects by destroying biological macromolecules in tumor cells. It is termed as the type I reaction (Debele et al., 2015). On the other hand, in the type II reaction the activated PS generates excited singlet oxygen (1O2) through the direct energy transfer from the triplet state PS to the triplet oxygen (Dang et al., 2017). Therefore, the accumulation of PS in tumor cells is the premise of PDT, and light and molecular oxygen are indispensable conditions for PDT (Pucelik et al., 2020).
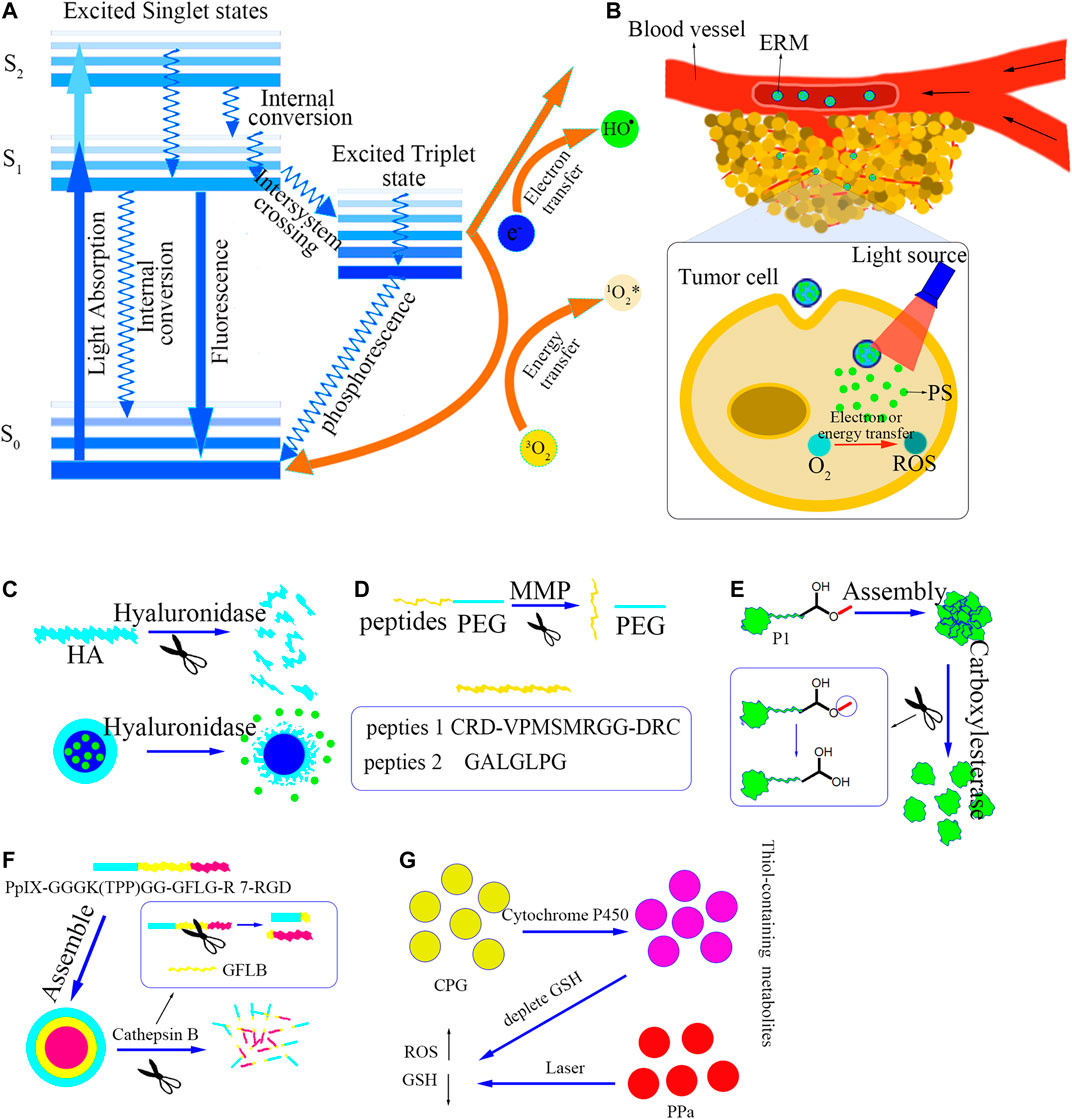
FIGURE 1. (A) The principle of ROS generated by photosensitizer excitation. (B) The process of ERM delivering PS. (C-G) The response principles of the five ERMs, followed by hyaluronidase-responsive, Matrix metalloproteinase-responsive, Carboxylesterase-responsive, Cathepsin B-responsive and Cytochrome P450-responsive.
PDT has recently shown promise in the treatment malignancies because of its high efficiency and safety. However, the hydrophobicity and poor targeting of PS need to be urgently addressed (Sivasubramanian et al., 2019). There are two main ways to solve them. Structural modification is a commonly used method to develop a new type of small molecule PS with excellent properties. At present, the use of responsive materials as delivery carrier provides noval strategy for addressing the hydrophobicity and poor targeting of PS (Bowman and Leong, 2006; Sheng et al., 2016; Zhang et al., 2018; Lin et al., 2021). Especially, tumor microenvironment-responsive material (TMRM) as an interesting delivery carrier is attracting extensive attention (Liu et al., 2020). TMRM can respond to the unique physiological environment of tumors, thereby selectively releasing PS and enhancing PDT (Torchilin, 2014). TMRMs mainly include hypoxia-responsive materials, pH-responsive materials, redox-responsive materials and enzyme-responsive materials (ERMs), etc (Wells et al., 2019).
Enzymes are essential for biological activities, and some uncontrolled and highly expressed enzymes in tumors are potential targets for stimulus response (Mura et al., 2013; Du and Tung, 2020). A large number of ERMs have been designed for use in drug delivery systems (Li et al., 2020; Mi, 2020). In PDT, ERM can also be used to deliver photosensitizers selectively to the tumor site, subsequently promoting the accumulation of PS in the tumor tissues and enhancing PDT efficiency through the enzymatic response to cause the transformation of intratumoral substances. In the present paper, we will review the application of ERM in PDT and discuss the challenges of ERM as carriers to deliver PS for further boosting the development of PDT in the management of malignancies.
Enzyme-Responsive Materials
ERM is a smart material that can respond to different enzymatic environments. ERMs have been widely used as a carrier for delivering PS to tumor tissues. As Figure 1B shown, after the PS-loaded ERM enters the tumor tissues, the PS can be specially released from the ERM by responding to the unique enzymes in the tumor tissues, and then the use of light irradiation activates the PS to produce ROS to kill the tumor cells. Recently, the reported ERMs used for PS delivery mainly include hyaluronidase-responsive material, matrix metalloproteinase-responsive material, carboxylesterase-responsive material, cathepsin B-responsive material, cytochrome P450-responsive material, etc. Their detailed information of the response sites and response principles is shown in Table 1.
Hyaluronidase-Responsive Material
Hyaluronidase is an enzyme that can decompose high-molecular hyaluronic acid into small-molecule hyaluronic acid (Lv et al., 2015). Growing evidence shows that hyaluronidase is a potential cancer biomarker and therapeutic target (McAtee et al., 2014). Hyaluronic acid (HA) is a water-soluble mucopolysaccharide, which is a ligand specifically binding to CD44 overexpressed in cancer cells (Choi et al., 2011; Yu et al., 2013; Dosio et al., 2016; Liu et al., 2020). Therefore, it is a reasonable choice to use materials containing hyaluronic acid to target tumor cells and/or to release photosensitizers in response to hyaluronidase in tumor tissues. Zhou (Zhou et al., 2020) constructed a mesoporous TiO2 doped with MnO2 (MTM) through an improved sol-gel method to develop co-doped photosensitizer TiO2 for enhancing PDT in the treatment of melanoma. MTM was also loaded with the antioxidant scavenger Au 25 Sv 9 and then modified by hyaluronic acid on the surface of MTM to avoid accidental release of Au 25 Sv 9. As Figure 1C shown, after the HA-modified MTM entering the tumor tissues, hyaluronidase decomposes hyaluronic acid to release Au 25 Sv 9 to remove thioredoxin reductase in tumor cells, MnO2 catalyzes H2O2 to produce oxygen to improve hypoxia environment, which promoted the killing effect of photodynamic action through light-excited TiO2. The authors found that the composite materials could improve hypoxia in tumor tissues and enhance PDT efficacy.
The use of hyaluronic acid as a sealing material of mesoporous silica can not only target CD44 receptors overexpressed in tumor cells, but also control the release of photosensitizers through enzyme response. Cheng (Cheng et al., 2021) reported a new type of nanohybrid based on GSH-responsive mesoporous organic silica nanoparticles (GMON), which could exert significant anti-tumor activity under near-infrared light irradiation. The nanosystem was composed of GSH-responsive mesoporous silica, photosensitizer IR820, hypoxia-responsive prodrug tirapazamine (TPZ) and hyaluronic acid. After administration, the nanosystem was endocytosed into tumor cells due to HA targeting CD44 receptor. The GMONs in tumor cells were degraded by the action of GSH and hyaluronidase, releasing TPZ and photosensitizer. After laser irradiation IR820 consumed oxygen to produce ROS, which further enhances the toxic effect of TPZ to tumor cells. HA has dual ability to target CD44 overexpressed in cancer cells and response to hyaluronidase overexpressed in cancer cells, which endows HA-modified materails double functions with targeted release and delivery of PS. At present, electrostatic absorption is a main method used for the modification of HA on the surface of nanomaterials. The electrostatic force is very weak and the modified nanomaterials are easily affected by the surrounding envirnoments. After entering the systemic circulation, HA on the surface of the nanomaterials is easy to drop from the materials and cause accidental release of PS. Therefore, if HA is modified on the nanomaterials through chemical bonds, the physicochemical properties and biological activity will be much improved.
Matrix Metalloproteinase-Responsive Material
Matrix metalloproteinases (MMP) are overexpressed in a variety of tumors, and are closely related to tumor growth, invasion and metastasis (Shinagawa et al., 1994; Coussens et al., 2002; Natarajan et al., 2006; Roy et al., 2008). A large number of anti-cancer strategies related to MMP have been proposed, especially MMP sensitive or responsive substances have been widely used to deliver drugs and reduce the side effects of drugs (Winer et al., 2018). In PDT, MMP is also used as an excellent stimulus source to targetedly deliver photosensitizers. As an important subcellular organelle, mitochondria are related to cell growth, differentiation and apoptosis. The development of photosensitizers with mitochondrial targeting is an effective way to enhance photodynamic therapy. (Li et al., 2017) synthesized a cationic oligomer (p-phenylene vinylene-copolymerized benzothiazole) (OPVBT) that could accumulate in mitochondria via electrostatic interactions. OPVBT could generate a large amount of ROS upon light irradiation and exhibited significant photodynamic activity. In their studies MMP-sensitive PEG peptide (CRD-VPMSMRGG-DRC) hydrogel was used to deliver OPVBT for improving tumor selectivity and reducing side effects. Due to the high level of MMP in tumor cells, hydrogels containing MMP-cleavable (Figure 1D) substrates could gradually be degraded, locally releasing OPVBT in tumor tissues. Their results showed that the hydrogel had no toxicity to normal cells, but it could be effectively decomposed after entering tumor tissues, to release the photosensitizer, showing high selectivity and low toxicity.
MMP-2 is one of MMPs, which are overexpressed in a variety of tumor cells (Wang et al., 2019; Stephens et al., 2020). (Gao et al., 2019) designed MMP-2 responsive exfoliable prodrug vesicles (EAPV) containing pegylated photosensitizer and NLG919 prodrug (NPC). PEG was modified on the surface of the vesicle through the MMP-responsive linker—GALGLPG (A short peptide), and when the vesicles reached tumor site, the GALGLPG peptide (Figure 1D) was cleaved by MMP-2 and then removed PEG to increase the endocytosis of the vesicles. After entering tumor cells, the loaded drug NLG-919 was released in response to GSH to inactivate indoleamine 2,3-dioxygenase 1, thereby overcoming the adaptive immune resistance caused by PDT. The results demonstrated that the EAPV nanoviscles exhibited uniform size distribution, good serum stability, and dual responsiveness to MMP-2 and GSH. EAPV could also penetrate deeply into tumor tissues and be easily absorbed by tumor cells. The in-vitro and in-vivo experiments confirmed that EAPV could significantly produce ROS, induce tumor cell ICD, and ultimately enhance the immune recognition of dendritic cells to tumor cells.
Carboxylesterase-Responsive Material
Carboxylesterase (CE) is a member of α/β hydrolase folding protein, which is mainly located in the endoplasmic reticulum of various mammalian cells (Barthel et al., 2009; Löser et al., 2013). CE participates in the hydrolysis of many xenobiotics and endogenous ester or amide compounds, and plays a key role in the metabolism and biotransform of some chemotherapeutics (Ke et al., 2020). Studies have shown that CEs are highly expressed in a variety of human tumor cells, so CE is also used as a target stimulus source to deliver drugs (Sanghani et al., 2003). Cai (Cai et al., 2020) modified the tetrachloroethylene monoimine core by β-alanine methyl ester, synthesized the CE-responsive precursor P1 as the substrate of CE and the electron donor of the system, and galactose as the tumor targeting ligand and hydrophilic group. The synthesized near-infrared (NIR)-activated amphiphile P1 and folate-modified human serum albumin (HSA) could assemble into nanoclusters (FHP) with a size of about 100 nm. As Figure 1E shown, when the methyl carboxylate of P1 was selectively hydrolyzed by CE at the tumor site to remove the methyl group, FHP decreased from ∼100 to ∼10 nm, which was conducive to the penetration of FHP in the deep tissues of the tumor. At the same time, the enzyme-triggered decomposition of FHP enhanced near-infrared fluorescence (NIRF) and promoted the generation of singlet oxygen (1O2), thereby improving imaging-guided PDT.
Cathepsin B-Responsive Material
The expression of cathepsin B is highly upregulated in malignant tumors and precancerous lesions, so cathepsin B can be used as a stimulus response target (Melancon and Li, 2011; ShiJie; Wang et al., 2020). Given that high levels of Cathepsin in tumor tissues can specifically cleave a tetra-peptide linker GFLG (Özel et al., 2015), Cheng (Cheng et al., 2020) constructed a tumor cell and mitochondrial dual-targeting nano-platform based on PpIX-GGGK (TPP)GG-GFLG-R7-RGD for the co-delivery of DOX and protoporphyrin IX (PpIX). The self-assembled nano-platform consisted of a hydrophobic PpIX-peptide conjugate (PpIX-GGGK (TPP)GG), a hydrophilic bioactive peptide with tumor-targeting RGD, cell membrane-penetrating R8 and cathepsin B. The cleaved linker GFLG is composed of self-assembled nanomicelles with a core-shell structure using hydrophilic-hydrophobic interactions, and DOX is loaded into the core through hydrophobic force and π-π interactions to form DOX@pPAC. The constructed nanosystem (DOX@pPAC) enhanced the uptake of PpIX and DOX in tumor cells through endocytosis mediated by integrin receptors. Later, the up-regulated lysosomal protease cathepsin B triggered the hydrolysis of the GFLG bond, followed by the dissociation of the DOX@ pPAC nanomicelles (Figure 1F). Then, the TPP cation of the PpIX-peptide conjugate greatly promoted the accumulation of PpIX in mitochondria, and significantly enhanced the PDT effect of PpIX on tumor cells. In this article, the researchers cleverly designed a multifunctional amphiphilic macromolecule that could self-assemble, integrating PDT, DOX, tumor targeting, mitochondrial targeting and enzyme response. The successful combination of chemotherapy and PDT amplifies the efficacy of chemotherapy and photodynamic therapy and accelerates the death of tumor cells.
Cytochrome P450-Responsive Material
Cytochrome P450 (CYP) is a multi-gene superfamily of constitutive and inducible heme-containing monooxygenases, involved in the metabolism of various xenobiotics and endogenous substrates (Blake et al., 2013; Wang et al., 2017; Dutkiewicz and Mikstacka, 2018). The CYP enzyme (P450) is located in the mitochondria and endoplasmic reticulum (Hayran et al., 2020). These enzymes use molecular oxygen and equivalent electrons transferred from the NADPH-P450 reductase in the endoplasmic reticulum or the ferredoxin and ferredoxin reductase in the mitochondria to catalyze the monooxygenase reaction (Renaud et al., 2017). Clopidogrel (CPG) is a classic antiplatelet prodrug widely used to treat thrombosis. Cytochrome P450 (CYP2C19) enzyme mediates the metabolism of CPG to form thiol-containing metabolites (Kazui et al., 2010). The formation of disulfide bonds between the cysteine residues of GSH and the thiol-containing metabolites of CPG to consume intracellular GSH is related to the antiplatelet mechanism of CPG. Inspired by the mechanism of CPG, Wang (Wang et al., 2020) used CPG to consume intracellular GSH in cancer cells expressing CYP2C19 to enhance the efficacy of PDT (Figure 1G). They firstly co-assembled CPG and photosensitizer pyropheophytin (PPa) to form nanoparticles, and then used DSPE-PEG to encapsulate the nanoparticles to form CPG/PPa NPs in order to enhance its stability. CPG and PPa formed stable and nearly spherical nanostructures through strong π-π stacking, hydrophobic interaction, hydrogen bonding and electrostatic interaction. Compared with free PPa, CPG/PPa NPs showed higher cytotoxicity to cancer cells and greater accumulation at the tumor site. After CPG/PPa NPs entering into tumor cell, CPG responded to CYP2C19 to form metabolites containing mercapto alcohols and destroy the redox homeostasis of tumor cells by consuming GSH, showed synergistic anti-tumor effect, and then enhance the efficacy of PDT in the 4T1 breast tumor xenograft model. However, the responsive materials were only modified using PEG. Although PEG modification would enhance the stability and half life of the nanoparticles in the systemic circulation, the negative charge of the PEG might make the modified nanoparticles difficult to enter into the cell. Therefore, the use of ligand molecules such as folic acid, biotin on the surface of nanoparticles might further improve the efficiency of the responsive materials in the PS delivery.
Summary and Outlook
Enzymes play an important role in physiological and pathological conditions of human body. Enzymes have excellent specificity and selectivity to bind and cleave their substrates, and the reactions are very fast and highly efficient, which provides noval strategy for controlled and targeted drug delivery (Mu et al., 2018). Recent studies have demonstrated that many enzymes are overexpressed in malignant cells and tissues. These overexpressed enzymes provide a unique platform to design ERMs to selectively delivering PSs to tumor tissues and cells. Up to now, series of ERMs, including hyaluronidase-responsive material, matrix metalloproteinase-responsive material, carboxylesterase-responsive material, cathepsin B-responsive material, and cytochrome P450-responsive material, have been researched and developed. Accumulating evidence shows that the ERMs exhibit many fascinating advantages, which not only possess captivating functions of nanomaterials, and also have enzyme-responsive specificity (Mu et al., 2018). These fascinating features make ERMs have promising potential in promoting targeted delivery of PSs and enhancing photodynamic efficacy. In gerenal, enzyme-responsive substrates used in the ERMs are biomolecules such as proteins and peptides. These biomolecules have good biocompatibility and biosafety. However, proteins and peptides easily degaded by exogenous and endogenous enzymes. Thus, the stability of ERMs in vitro and in vivo should be concerned and improved.
Recently, the physicochemical properties and therapeutic effects of ERMs have been widely studied. The results from the in-vitro and in-vivo studies have demonstrated that ERMs had a promising prospect in the target delivery of PSs, increase of photodynamic efficacy, and synergetic treatment of photodynamic therapy and chemotherapy/immunotherapy. However, the application of ERMs to deliver PSs in the management of malignacies is still in infancy. Their biosafety, biopharmaceutic and pharmacokinetic performance are rarely been concerned. And few clinical trials were conducted to investigate the precise delivery of PSs for PDT using ERMs. Additionally, tedious preparation, complicated characterization, and uncertainty of the in-vivo fate of ERMs are still challenges in the translation of ERMs to clinical applications. Therefore, overcoming the above-mentioned shortcomings and addressing these challenges should be important tasks for translating ERMs as a targeted delivery carrier of PSs for enhancing photodynamic therapy in the clinical settings.
Author Contributions
HL: Principal writer. CX: Article revision and review before submission. FY, WC, TG, YZ, XD, and WL: Writing–Original draft preparation.
Funding
This work was supported by the grants of High-level University Construction Fund of Guangdong Province (No. 06-410-2106154, 06-410-2106153, 06-410-2107229), the fund of Guangdong-Hongkong-Macao Joint Laboratory of Respiratory Infectious Disease for Dr Wenjie Chen (No. GHMJLRID-Z-202113).
Conflict of Interest
The authors declare that the research was conducted in the absence of any commercial or financial relationships that could be construed as a potential conflict of interest.
Publisher’s Note
All claims expressed in this article are solely those of the authors and do not necessarily represent those of their affiliated organizations, or those of the publisher, the editors and the reviewers. Any product that may be evaluated in this article, or claim that may be made by its manufacturer, is not guaranteed or endorsed by the publisher.
References
Ackroyd, R., Kelty, C., Brown, N., and Reed, M. (2001). The History of Photodetection and Photodynamic Therapy. Photochem. Photobiol. 74 (5), 656–669. doi:10.1562/0031-8655(2001)074<0656:thopap>2.0.co;2
Barthel, B. L., Zhang, Z., Rudnicki, D. L., Coldren, C. D., Polinkovsky, M., Sun, H., et al. (2009). Preclinical Efficacy of a Carboxylesterase 2-Activated Prodrug of Doxazolidine. J. Med. Chem. 52 (23), 7678–7688. doi:10.1021/jm900694z
Blake, L. C., Roy, A., Neul, D., Schoenen, F. J., Aubé, J., and Scott, E. E. (2013). Benzylmorpholine Analogs as Selective Inhibitors of Lung Cytochrome P450 2A13 for the Chemoprevention of Lung Cancer in Tobacco Users. Pharm. Res. 30 (9), 2290–2302. doi:10.1007/s11095-013-1054-z
Bowman, K., and Leong, K. W. (2006). Chitosan Nanoparticles for Oral Drug and Gene Delivery. Int. J. Nanomedicine 1 (2), 117–128. doi:10.2147/nano.2006.1.2.117
Bown, S. G. (20131995). Photodynamic Therapy for Photochemists. Phil. Trans. R. Soc. A. 371, 20120371. doi:10.1098/rsta.2012.0371
Cai, Y., Ni, D., Cheng, W., Ji, C., Wang, Y., Müllen, K., et al. (2020). Enzyme‐Triggered Disassembly of Perylene Monoimide‐based Nanoclusters for Activatable and Deep Photodynamic Therapy. Angew. Chem. Int. Ed. 59 (33), 14014–14018. doi:10.1002/anie.202001107
Cheng, D., Ji, Y., Wang, B., Jin, T., Xu, Y., Qian, X., et al. (2021). Enzyme/GSH Dual-Responsive Biodegradable Nanohybrid for Spatiotemporally Specific Photodynamic and Hypoxia-Augmented Therapy against Tumors. Int. J. pharmaceutics 603, 120730. doi:10.1016/j.ijpharm.2021.120730
Cheng, Y. J., Qin, S. Y., Liu, W. L., Ma, Y. H., Chen, X. S., Zhang, A. Q., et al. (2020). Dual‐Targeting Photosensitizer‐Peptide Amphiphile Conjugate for Enzyme‐Triggered Drug Delivery and Synergistic Chemo‐Photodynamic Tumor Therapy. Adv. Mater. Inter. 7 (19), 2000935. doi:10.1002/admi.202000935
Choi, K. Y., Yoon, H. Y., Kim, J.-H., Bae, S. M., Park, R.-W., Kang, Y. M., et al. (2011). Smart Nanocarrier Based on PEGylated Hyaluronic Acid for Cancer Therapy. ACS Nano 5 (11), 8591–8599. doi:10.1021/nn202070n
Coussens, L. M., Fingleton, B., and Matrisian, L. M. (2002). Matrix Metalloproteinase Inhibitors and Cancer-Trials and Tribulations. Science 295 (5564), 2387–2392. doi:10.1126/science.1067100
Dang, J., He, H., Chen, D., and Yin, L. (2017). Manipulating Tumor Hypoxia toward Enhanced Photodynamic Therapy (PDT). Biomater. Sci. 5 (8), 1500–1511. doi:10.1039/C7BM00392G
Debele, T., Peng, S., and Tsai, H.-C. (2015). Drug Carrier for Photodynamic Cancer Therapy. Int. J. Mol. Sci. 16 (9), 22094–22136. doi:10.3390/ijms160922094
Diogo, P., Gonçalves, T., Palma, P., and Santos, J. M. (2015). Photodynamic Antimicrobial Chemotherapy for Root Canal System Asepsis: A Narrative Literature Review. Int. J. Dentistry 2015, 1–26. doi:10.1155/2015/269205
Dolmans, D. E. J. G. J., Fukumura, D., and Jain, R. K. (2003). Photodynamic Therapy for Cancer. Nat. Rev. Cancer 3 (5), 380–387. doi:10.1038/nrc1071
Dosio, F., Arpicco, S., Stella, B., and Fattal, E. (2016). Hyaluronic Acid for Anticancer Drug and Nucleic Acid Delivery. Adv. Drug Deliv. Rev. 97, 204–236. doi:10.1016/j.addr.2015.11.011
Du, B., and Tung, C.-H. (2020). Enzyme-Assisted Photodynamic Therapy Based on Nanomaterials. ACS Biomater. Sci. Eng. 6 (5), 2506–2517. doi:10.1021/acsbiomaterials.9b00968
Dutkiewicz, Z., and Mikstacka, R. (2018). Structure-Based Drug Design for Cytochrome P450 Family 1 Inhibitors. Bioinorganic Chem. Appl. 2018, 1–21. doi:10.1155/2018/3924608
Fan, W., Huang, P., and Chen, X. (2016). Overcoming the Achilles' Heel of Photodynamic Therapy. Chem. Soc. Rev. 45 (23), 6488–6519. doi:10.1039/C6CS00616G
Gao, A., Chen, B., Gao, J., Zhou, F., Saeed, M., Hou, B., et al. (2019). Sheddable Prodrug Vesicles Combating Adaptive Immune Resistance for Improved Photodynamic Immunotherapy of Cancer. Nano Lett. 20 (1), 353–362. doi:10.1021/acs.nanolett.9b04012
Hayran, Y., Allı, N., Uysal, P. İ., and Çandar, T. (2020). Association of CYP2J2 Polymorphism with Susceptibility to Psoriasis in Turkish Population: a Case-Control Study. Anais Brasileiros de Dermatologia 95 (1), 25–31. doi:10.1016/j.abd.2019.04.006
Izumoto, A., Nishimura, T., Hazama, H., Ikeda, N., Kajimoto, Y., and Awazu, K. (2019). Singlet Oxygen Model Evaluation of Interstitial Photodynamic Therapy with 5-aminolevulinic Acid for Malignant Brain Tumor. J. Biomed. Opt. 25 (06), 1. doi:10.1117/1.JBO.25.6.063803
Jiang, H., Du, Y., Chen, L., Qian, M., Yang, Y., Huo, T., et al. (2020). Multimodal Theranostics Augmented by Transmembrane Polymer-Sealed Nano-Enzymatic Porous MoS2 Nanoflowers. Int. J. Pharmaceutics 586, 119606. doi:10.1016/j.ijpharm.2020.119606
Kazui, M., Nishiya, Y., Ishizuka, T., Hagihara, K., Farid, N. A., Okazaki, O., et al. (2010). Identification of the Human Cytochrome P450 Enzymes Involved in the Two Oxidative Steps in the Bioactivation of Clopidogrel to its Pharmacologically Active Metabolite. Drug Metab. Dispos 38 (1), 92–99. doi:10.1124/dmd.109.029132
Ke, C.-C., Chen, L.-C., Yu, C.-C., Cheng, W.-C., Huang, C.-Y., Lin, V. C., et al. (2020). Genetic Analysis Reveals a Significant Contribution of CES1 to Prostate Cancer Progression in Taiwanese Men. Cancers 12 (5), 1346. doi:10.3390/cancers12051346
Li, M., He, P., Li, S., Wang, X., Liu, L., Lv, F., et al. (2017). Oligo(p-phenylenevinylene) Derivative-Incorporated and Enzyme-Responsive Hybrid Hydrogel for Tumor Cell-specific Imaging and Activatable Photodynamic Therapy. ACS Biomater. Sci. Eng. 4 (6), 2037–2045. doi:10.1021/acsbiomaterials.7b00610
Li, M., Zhao, G., Su, W.-K., and Shuai, Q. (2020). Enzyme-Responsive Nanoparticles for Anti-tumor Drug Delivery. Front. Chem. 8. doi:10.3389/fchem.2020.00647
Li, Z., Ni, J., Liu, L., Gu, L., Wu, Z., Li, T., et al. (2021). Imaging-Guided Chemo-Photothermal Polydopamine Carbon Dots for EpCAM-Targeted Delivery toward Liver Tumor. ACS Appl. Mater. Inter. 13 (25), 29340–29348. doi:10.1021/acsami.1c05079
Lin, X., Wu, X., Chen, X., Wang, B., and Xu, W. (2021). Intellective and Stimuli-Responsive Drug Delivery Systems in Eyes. Int. J. Pharmaceutics 602, 120591. doi:10.1016/j.ijpharm.2021.120591
Liu, H., Quan, Y., Jiang, X., Zhao, X., Zhou, Y., Fu, J., et al. (2020). Using Polypeptide Bearing Furan Side Chains as a General Platform to Achieve Highly Effective Preparation of Smart Glycopolypeptide Analogue-Based Nano-Prodrugs for Cancer Treatment. Colloids Surf. B: Biointerfaces 194, 111165. doi:10.1016/j.colsurfb.2020.111165
Liu, H., Yao, J., Guo, H., Cai, X., Jiang, Y., Lin, M., et al. (2020). Tumor Microenvironment-Responsive Nanomaterials as Targeted Delivery Carriers for Photodynamic Anticancer Therapy. Front. Chem. 8. doi:10.3389/fchem.2020.00758
Löser, R., Fischer, S., Hiller, A., Köckerling, M., Funke, U., Maisonial, A., et al. (2013). Use of 3-[18F]fluoropropanesulfonyl Chloride as a Prosthetic Agent for the Radiolabelling of Amines: Investigation of Precursor Molecules, Labelling Conditions and Enzymatic Stability of the Corresponding Sulfonamides. Beilstein J. Org. Chem. 9, 1002–1011. doi:10.3762/bjoc.9.115
Lv, S. H., Rong, S. F., Cai, B. G., Guan, S. M., and Li, Q. Q. (2015). Property and Current Clinical Applications of Mammal Hyaluronidase. Eur. Rev. Med. Pharmacol. Sci. 19 (20), 3968–3976.
Mansoori, B., Mohammadi, A., Amin Doustvandi, M., Mohammadnejad, F., Kamari, F., Gjerstorff, M. F., et al. (2019). Photodynamic Therapy for Cancer: Role of Natural Products. Photodiagnosis Photodynamic Ther. 26, 395–404. doi:10.1016/j.pdpdt.2019.04.033
McAtee, C. O., Barycki, J. J., and Simpson, M. A. (2014). Emerging Roles for Hyaluronidase in Cancer Metastasis and Therapy. United States: Elsevier Science & Technology, 1–34. doi:10.1016/b978-0-12-800092-2.00001-0
Melancon, M. P., and Li, C. (2011). Multifunctional Synthetic poly(L-Glutamic Acid)-Based Cancer Therapeutic and Imaging Agents. Mol. Imaging 10 (1), 42. doi:10.2310/7290.2011.00007
Mi, P. (2020). Stimuli-responsive Nanocarriers for Drug Delivery, Tumor Imaging, Therapy and Theranostics. Theranostics 10 (10), 4557–4588. doi:10.7150/thno.38069
Mu, J., Lin, J., Huang, P., and Chen, X. (2018). Development of endogenous enzyme-responsive nanomaterials for theranostics. Chem Soc Rev 47 (15), 5554–5573. doi:10.1039/C7CS00663B
Muniyandi, K., George, B., Parimelazhagan, T., and Abrahamse, H. (2020). Role of Photoactive Phytocompounds in Photodynamic Therapy of Cancer. Molecules 25 (18), 4102. doi:10.3390/molecules25184102
Mura, S., Nicolas, J., and Couvreur, P. (2013). Stimuli-responsive Nanocarriers for Drug Delivery. Nat. Mater 12 (11), 991–1003. doi:10.1038/nmat3776
Natarajan, J., Berrar, D., Dubitzky, W., Hack, C., Zhang, Y., DeSesa, C., et al. (2006). Text Mining of Full-Text Journal Articles Combined with Gene Expression Analysis Reveals a Relationship between Sphingosine-1-Phosphate and Invasiveness of a Glioblastoma Cell Line. BMC Bioinformatics 7 (1), 373. doi:10.1186/1471-2105-7-373
Özel, T., White, S., Nguyen, E., Moy, A., Brenes, N., Choi, B., et al. (2015). Enzymatically Activated Near Infrared Nanoprobes Based on Amphiphilic Block Copolymers for Optical Detection of Cancer. Lasers Surg. Med. 47 (7), 579–594. doi:10.1002/lsm.22396
Pucelik, B., Sułek, A., Barzowska, A., and Dąbrowski, J. M. (2020). Recent Advances in Strategies for Overcoming Hypoxia in Photodynamic Therapy of Cancer. Cancer Lett. 492, 116–135. doi:10.1016/j.canlet.2020.07.007
Renaud, L., Silveira, W. A. d., Hazard, E. S., Simpson, J., Falcinelli, S., Chung, D., et al. (2017). The Plasticizer Bisphenol A Perturbs the Hepatic Epigenome: A Systems Level Analysis of the miRNome. Genes 8 (10), 269. doi:10.3390/genes8100269
Roy, R., Louis, G., Loughlin, K. R., Wiederschain, D., Kilroy, S. M., Lamb, C. C., et al. (2008). Tumor-Specific Urinary Matrix Metalloproteinase Fingerprinting: Identification of High Molecular Weight Urinary Matrix Metalloproteinase Species. Clin. Cancer Res. 14 (20), 6610–6617. doi:10.1158/1078-0432.CCR-08-1136
Sanghani, S. P., Quinney, S. K., Fredenburg, T. B., Sun, Z., Davis, W. I., Murry, D. J., et al. (2003). Carboxylesterases Expressed in Human Colon Tumor Tissue and Their Role in CPT-11 Hydrolysis. Clin. Cancer Res. 9 (13), 4983–4991.
Sato, H., Takino, T., Okada, Y., Cao, J., Shinagawa, A., Yamamoto, E., et al. (1994). A Matrix Metalloproteinase Expressed on the Surface of Invasive Tumour Cells. Nature 370 (6484), 61–65. doi:10.1038/370061a0
Schalch, T. O., Palmieri, M., Longo, P. L., Braz-Silva, P. H., Tortamano, I. P., Michel-Crosato, E., et al. (2019). Evaluation of Photodynamic Therapy in Pericoronitis. Medicine 98 (17), e15312. doi:10.1097/MD.0000000000015312
Sheng, R., Zhuang, X., Wang, Z., Cao, A., Lin, K., and Zhu, J. (2016). Cationic Nanoparticles Assembled from Natural-Based Steroid Lipid for Improved Intracellular Transport of siRNA and pDNA. Nanomaterials 6 (4), 69. doi:10.3390/nano6040069
Sivasubramanian, M., Chuang, Y., and Lo, L.-W. (2019). Evolution of Nanoparticle-Mediated Photodynamic Therapy: From Superficial to Deep-Seated Cancers. Molecules 24 (3), 520. doi:10.3390/molecules24030520
Stephens, D. C., Powell, T. W., Taraska, J. W., and Harris, D. A. (2020). Imaging the Rapid yet Transient Accumulation of Regulatory Lipids, Lipid Kinases, and Protein Kinases during Membrane Fusion, at Sites of Exocytosis of MMP-9 in MCF-7 Cells. Lipids Health Dis. 19 (1). doi:10.1186/s12944-020-01374-9
Torchilin, V. P. (2014). Multifunctional, Stimuli-Sensitive Nanoparticulate Systems for Drug Delivery. Nat. Rev. Drug Discov. 13 (11), 813–827. doi:10.1038/nrd4333
Wang, D., Wang, T., Yu, H., Feng, B., Zhou, L., Zhou, F., et al. (2019). Engineering Nanoparticles to Locally Activate T Cells in the Tumor Microenvironment. Sci. Immunol. 4 (37), 6584. doi:10.1126/sciimmunol.aau6584
Wang, Q., Sun, M., Li, D., Li, C., Luo, C., Wang, Z., et al. (2020). Cytochrome P450 Enzyme-Mediated Auto-Enhanced Photodynamic Cancer Therapy of Co-nanoassembly between Clopidogrel and Photosensitizer. Theranostics 10 (12), 5550–5564. doi:10.7150/thno.42633
Wang, R.-L., He, Y.-N., Staehelin, C., Liu, S.-W., Su, Y.-J., and Zhang, J.-E. (2017). Identification of Two Cytochrome Monooxygenase P450 Genes, CYP321A7 and CYP321A9, from the Tobacco Cutworm Moth (Spodoptera Litura) and Their Expression in Response to Plant Allelochemicals. Int. J. Mol. Sci. 18 (11), 2278. doi:10.3390/ijms18112278
Wang, S.-J., Chao, D., Wei, W., Nan, G., Li, J.-Y., Liu, F.-L., et al. (2020). CD147 Promotes Collective Invasion through Cathepsin B in Hepatocellular Carcinoma. J. Exp. Clin. Cancer Res. 39 (1). doi:10.1186/s13046-020-01647-2
Wells, C. M., Harris, M., Choi, L., Murali, V. P., Guerra, F. D., and Jennings, J. A. (2019). Stimuli-Responsive Drug Release from Smart Polymers. Jfb 10 (3), 34. doi:10.3390/jfb10030034
Winer, A., Adams, S., and Mignatti, P. (2018). Matrix Metalloproteinase Inhibitors in Cancer Therapy: Turning Past Failures into Future Successes. Mol. Cancer Ther. 17 (6), 1147–1155. doi:10.1158/1535-7163.MCT-17-0646
Wu, H., Minamide, T., and Yano, T. (2019). Role of Photodynamic Therapy in the Treatment of Esophageal Cancer. Dig. Endosc. 31 (5), 508–516. doi:10.1111/den.13353
Xing, Y., Ding, T., Wang, Z., Wang, L., Guan, H., Tang, J., et al. (2019). Temporally Controlled Photothermal/Photodynamic and Combined Therapy for Overcoming Multidrug Resistance of Cancer by Polydopamine Nanoclustered Micelles. ACS Appl. Mater. Inter. 11 (15), 13945–13953. doi:10.1021/acsami.9b00472
Yu, M., Jambhrunkar, S., Thorn, P., Chen, J., Gu, W., and Yu, C. (2013). Hyaluronic Acid Modified Mesoporous Silica Nanoparticles for Targeted Drug Delivery to CD44-Overexpressing Cancer Cells. Nanoscale 5 (1), 178–183. doi:10.1039/C2NR32145A
Zhang, C., Gao, F., Wu, W., Qiu, W.-X., Zhang, L., Li, R., et al. (2019). Enzyme-Driven Membrane-Targeted Chimeric Peptide for Enhanced Tumor Photodynamic Immunotherapy. ACS Nano 13 (10), 11249–11262. doi:10.1021/acsnano.9b04315
Zhang, J., Jiang, C., Figueiró Longo, J. P., Azevedo, R. B., Zhang, H., and Muehlmann, L. A. (2018). An Updated Overview on the Development of New Photosensitizers for Anticancer Photodynamic Therapy. Acta Pharmaceutica Sinica B 8 (2), 137–146. doi:10.1016/j.apsb.2017.09.003
Zhang, S., Han, F., Liang, Z., Tan, J., Cao, W., Gao, Y., et al. (2019). An Investigation of CNN Models for Differentiating Malignant from Benign Lesions Using Small Pathologically Proven Datasets. Comput. Med. Imaging Graphics 77, 101645. doi:10.1016/j.compmedimag.2019.101645
Zhao, B., and He, Y.-Y. (2014). Recent Advances in the Prevention and Treatment of Skin Cancer Using Photodynamic Therapy. Expert Rev. Anticancer Ther. 10 (11), 1797–1809. doi:10.1586/era.10.154
Zhou, J., Geng, S., Ye, W., Wang, Q., Lou, R., Yin, Q., et al. (2020). ROS-boosted Photodynamic Therapy against Metastatic Melanoma by Inhibiting the Activity of Antioxidase and Oxygen-Producing Nano-Dopants. Pharmacol. Res. 158, 104885. doi:10.1016/j.phrs.2020.104885
Keywords: photodynamic therapy, photosensitizer, smart materials, enzyme-responsive materials, carrier
Citation: Liu H, Yang F, Chen W, Gong T, Zhou Y, Dai X, Leung W and Xu C (2021) Enzyme-Responsive Materials as Carriers for Improving Photodynamic Therapy. Front. Chem. 9:763057. doi: 10.3389/fchem.2021.763057
Received: 23 August 2021; Accepted: 20 October 2021;
Published: 02 November 2021.
Edited by:
Lei Wang, Harbin Institute of Technology, ChinaReviewed by:
Xing Ma, Harbin Institute of Technology, ChinaTiedong Sun, Northeast Forestry University, China
Copyright © 2021 Liu, Yang, Chen, Gong, Zhou, Dai, Leung and Xu. This is an open-access article distributed under the terms of the Creative Commons Attribution License (CC BY). The use, distribution or reproduction in other forums is permitted, provided the original author(s) and the copyright owner(s) are credited and that the original publication in this journal is cited, in accordance with accepted academic practice. No use, distribution or reproduction is permitted which does not comply with these terms.
*Correspondence: Chuanshan Xu, eGNzaGFuQDE2My5jb20=