- 1Department of Biomolecular Sciences, Faculty of Pharmaceutical Sciences of Ribeirão Preto, University of São Paulo, Ribeirão Preto, Brazil
- 2Centro de Química Estrutural, Institute of Molecular Sciences & Departamento de Engenharia Química, Instituto Superior Técnico, Universidade de Lisboa, Lisboa, Portugal
- 3LAQV-REQUIMTE, Chemistry Department, University of Aveiro, Aveiro, Portugal
Phthalocyanine (Pc) dyes are photoactive molecules that can absorb and emit light in the visible spectrum, especially in the red region of the spectrum, with great potential for biological scopes. For this target, it is important to guarantee a high Pc solubility, and the use of suitable pyridinium units on their structure can be a good strategy to use effective photosensitizers (PSs) for photodynamic therapy (PDT) against cancer cells. Zn(II) phthalocyanines (ZnPcs) conjugated with thiopyridinium units (1–3) were evaluated as PS drugs against B16F10 melanoma cells, and their photophysical, photochemical, and in vitro photobiological properties were determined. The photodynamic efficiency of the tetra- and octa-cationic ZnPcs 1–3 was studied and compared at 1, 2, 5, 10, and 20 µM. The different number of charge units, and the presence/absence of a-F atoms on the Pc structure, contributes for their PDT efficacy. The 3-(4′,5′-dimethylthiazol-2′-yl)-2,5-diphenyl tetrazolium bromide (MTT) assays on B16F10 melanoma cells show a moderate to high capacity to be photoinactivated by ZnPcs 1–3 (ZnPc 1 > ZnPc 2 > ZnPc 3). The best PDT conditions were found at a Pc concentration of 20 μM, under red light (λ = 660 ± 20 nm) at an irradiance of 4.5 mW/cm2 for 667 s (light dose of 3 J/cm2). In these conditions, it is noteworthy that the cationic ZnPc 1 shows a promising photoinactivation ratio, reaching the detection limit of the MTT method. Moreover, these results are comparable to the better ones in the literature.
Introduction
Melanoma, a well-known malignant, aggressive, and invasive skin carcinoma, is formed by a decontrolled transformation of melanocytes, which is the main reason of cell death in skin cancer (Siegel et al., 2021). From the global scientific data of the International Agency for Research on Cancer (IARC) of the World Health Organization (WHO), the occurrence of melanoma health problems has been growing over time and represents a high ratio of skin cancer deaths (Globocan. Melanoma of ski, 2020). Recently, the Global Cancer Statistics reported the possible occurrence of 324,635 melanoma cases of skin cancer and estimated 57,043 cancer fatalities to have happened in 185 countries during 2020 (Sung et al., 2021). It is noteworthy that more than half of the melanomas evidence BRAF V600 mutations (Ascierto et al., 2012) with ∼25% of neuroblastoma RAS viral oncogene homolog (NRAS) gene mutations (Rajkumar and Watson, 2016). For the clinical treatment, surgery and chemotherapy processes are active and useful options for patients diagnosed with melanoma disease. For growing-phase melanoma cases, medicinal treatments have been remarkably developed with the incorporation of immune inhibitor types and selective therapy such as BRAF and mitogen-activated protein kinase (MEK) inhibitors and programmed cell death ligand-1 (PDL-1) blockage (Ribas et al., 2020). However, the use of these components has limitations due to the high rate of innate or developed resistance in progressive metastatic melanoma (Welsh et al., 2016; Kozar et al., 2019; Vasan et al., 2019; Savoia et al., 2020).
Immunotherapy and other cancer therapies have been explored with relative success, increasing the survival of patients with malignant melanoma disease. However, the response in some patients is not effective due to the development of melanoma resistance after treatment (Winder and Virós, 2018; Czarnecka et al., 2020). Indeed, to combat this intrinsic resistance to the existing methodologies of treatment, photodynamic therapy (PDT) is a therapeutic alternative that can be used as an encouraging clinical approach (Marciel et al., 2017; van Straten et al., 2017; Valli et al., 2019). The PDT process involves the biological administration of a photosensitizer (PS) drug that is activated by suitable visible or near-infrared lights, which in the presence of cellular molecular oxygen generates highly cytotoxic reactive oxygen species (ROS), including the singlet oxygen (1O2) (Karunakaran et al., 2013; Lourenço et al., 2014a; Saenz et al., 2017; Pereira et al., 2018; Zhang et al., 2020; Castro et al., 2021). These ROS are responsible for the reduction or extinction of the targeted cancer cells or tumor tissues (Lourenço et al., 2014b; Ferreira et al., 2020).
Phthalocyanine (Pc) derivatives are photoactive compounds (ideally absorb light in the UV–Vis spectrum), especially in the red to near-infrared regions, which allow a higher tissue penetration and, consequently, a better PDT response (Camerin et al., 2010; Vummidi et al., 2013; Lo et al., 2020; Lopes-Nunes et al., 2020; Galstyan, 2021; Janas et al., 2021; Ribeiro and Lourenço, 2021). However, Pc macrocycles have weak solubility in various organic solvents or in aqueous media, and therefore, to minimize this drawback, it is essential to incorporate “bio”motifs, such as biologics or charged groups, including cationic pyridinium units on their structure to improve their amphiphilicity (Lourenço et al., 2015; Lourenço et al., 2019; Gamelas et al., 2020; Lopes-Nunes et al., 2020; Pereira et al., 2020; Revuelta-Maza et al., 2020; Revuelta‐Maza et al., 2021). Different peripheral substituents (α- and/or ß-positions) and their number usually give distinct photophysical, photochemical, and PDT results (Halaskova et al., 2021).
Recently, Valli et al. (2020) described the oxidative stress-induced apoptotic and autophagic signaling pathways using ZnPc dyes for PDT of melanoma cells, the induction process of apoptotic response, and a triggering protective autophagy. The same authors also reported the oxidative stress caused by light irradiation of ZnPcs inducing a dual apoptotic and necrotic response in melanoma cells. Mantareva et al. (2005) reported long-wavelength-absorbing cationic ZnPcs as fluorescent contrast agents for B16 pigmented melanoma. In their work, the Pcs were found to selectively accumulate in the target tumor, providing a potential application for fluorescence detection in clinical practice. Zheng et al. (2020) developed non-aggregated ZnPcs with hexadeca cations for antitumor and antibacterial PDT, evidencing that the quaternized compounds are efficient and can be used as promising PS drugs.
The worldwide interest in cancer treatment, such as the very aggressive melanoma, led us to study a series of three thiopyridinium ZnPc dyes 1–3 (Figure 1), previously synthetized (Pereira et al., 2012) towards the photoinactivation of B16F10 melanoma cells.
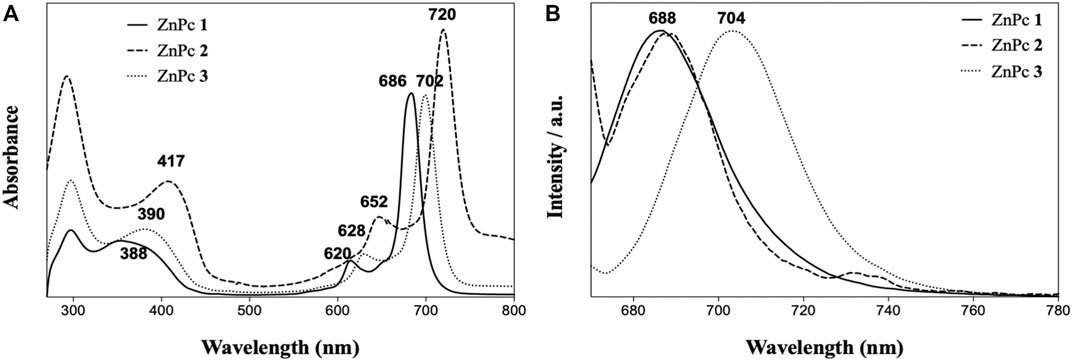
FIGURE 1. Spectra of (A) UV–Vis absorption and (B) normalized emission (λexc. = 660 nm) of ZnPcs 1–3 in DMF.
Experimental
All reagents were purchased from Sigma-Aldrich, Merck, or Gibco (without further purification). Analytical TLC was carried out on pre-coated silica gel sheets (Merck, 60, 0.2 mm). 1H, 19F, and 13C NMR spectra of Zn(II) phthalocyanine dyes (ZnPcs 1–3) (Pereira et al., 2012) were recorded on a Bruker Avance-300 spectrometer at 300.13, 282.38, and 75.47 MHz, respectively, or on a Bruker Avance-500 (13C NMR at 125.77 MHz). The characterization of the obtained compounds corresponded to their full characterization previously reported (Pereira et al., 2012). Absorption and fluorescence spectra were recorded using an Agilent 8,453 and F4500 Hitachi spectrofluorometer (λexc. at 660 nm, emission range 670–800 nm), respectively. The fluorescence emission spectra of Pc derivatives (C = 1 × 10−6 M) were recorded in DMF in 1 × 1 cm quartz optical cells under normal atmospheric conditions on a computer-controlled F4500 Hitachi spectrofluorometer. The fluorescence quantum yields were obtained using the commercial Zn(II) phthalocyanine (ZnPc) as standard (Gümrükçü et al., 2014) at optical density (O.D.) = 0.05 and excitation wavelength at 660 nm. The widths of both excitation and emission slits were set at 2.0 nm. The singlet oxygen quantum yield (ФΔ) generated by the ZnPc dyes (ZnPcs 1–3) were determined from the rate of decay of the 1O2 phosphorescence at 1,270 nm using an Edinburgh F900 instrument (Edinburgh, UK) consisting of a Rainbow OPO (Quantel Laser, France), 10 Hz, 2 mJ/pulse, which was pumped by a Brilliant NdYAG laser (Quantel Laser, France), and using ZnPc as the standard in DMF. The absorbance of the sample was determined in DMF and adjusted at O.D. = 0.1 with excitation wavelength at 660 nm. The 3-(4′,5′-dimethylthiazol-2′-yl)-2,5-diphenyl tetrazolium bromide (MTT) assays were performed to test the in vitro cytotoxicity of Zn(II) phthalocyanines (ZnPcs 1–3) against B16F10 melanoma cells.
Synthesis of Thiopyridinium Zn(II) Phthalocyanines
The cationic ZnPcs1–3 (Figure 1) were obtained from the experimental procedure reported in the literature (Pereira et al., 2012). Synthesis of the thiopyridyl ZnPcs was achieved either from a tetramerization process of phthalonitrile derivatives or via post-modification of the commercial Zn(II) hexadecafluorphthalocyanine (ZnPcF16). Then, the obtained thiopyridyl ZnPcs were cationized using methyl iodide to obtain the quaternized ZnPcs 1–3.
Cell Culture
B16F10 cells (murine melanoma cell line) were purchased from the American Type Culture Collection (ATCC®, N° CRL-6324TM) and grown in Roswell Park Memorial Institute (RPMI)-1,640 culture medium supplemented with 10% fetal bovine serum (FBS), 100 units/mL of penicillin, and 10 mg/ml of streptomycin.
Photobiological Studies In Vitro
An amount of 2 × 104 cells/well were seeded into 96-well plates and, subsequently, incubated for 24 h at 37°C in a 5% CO2 humidified atmosphere. After the incubation process, the cultures were incubated with different concentrations of ZnPcs 1–3 (1, 2, 5, 10, and 20 μM) for 4 h. The cultures were gently rinsed with phosphate buffered saline (PBS) solution, Roswell Park Memorial Institute (RPMI)-1,640 medium without phenol red was added, and then, PDT assays were performed. The cells were exposed to red light (emission peak maximum at 660 nm) emitted by an array of 96 light-emitting diodes (LEDs). The light irradiation dose was 3 J/cm2. After irradiation, the cultures were maintained at 37°C in a 5% CO2 humidified atmosphere. After 24 h of incubation, the cell viability was evaluated using MTT assay. Dark assays were performed at the same conditions used in the PDT studies. The irradiated control, cells without Pcs, was also evaluated. Three independent assays were performed with six replicates.
Photobleaching Studies
The photobleaching studies of ZnPcs 1–3 were evaluated by exposing a solution of each ZnPc in RPMI-1640 (10 μM) under the same conditions used in the PDT experiments. The stability of ZnPcs 1–3 was verified by analyzing the absorption spectra at regular intervals for up to 10 min.
Cellular Uptake
An amount of 5 × 104 cells/well were seeded into 24-well plates and, subsequently, incubated for 24 h under the same conditions used in the photosensitization experiments. After this time, the supernatant was removed, and the cells were incubated with each PS at 20 μM for 4 h. Subsequently, the cells were washed twice with PBS, and 500 μL of serum-free RPMI without phenol red was added. The cells were observed by fluorescence microscopy (Nikon Eclipse Ti Microscope model TI-FL). Filter Cy5 (λexc. = 620/660 nm and λem. = 662.5 to 737.5 nm) was used for ZnPc detection.
Statistical Analysis
The statistical analysis was performed by an unvaried ANOVA using GraphPrism 7. Similarity of variance was assumed with Bonferroni’s post hoc test for pairwise comparisons. Results with p ≤ 0.05 were considered statistically significant.
Results and Discussion
Synthesis of Thiopyridinium Zn(II) Phthalocyanine Dyes
The synthesis and characterization of phthalocyanines ZnPcs 1–3 containing thiopyridinium substituents (Scheme 6) was performed. The compounds were fully characterized by NMR, UV–Vis absorption, and emission spectroscopy, as well as mass spectrometry, as previously described in the literature (Pereira et al., 2012).
From the prepared compounds, it is important to highlight that the peripheral moieties not only influenced the photochemical and photophysical properties but also the photostability, production of singlet oxygen, lipophilicity, and cellular uptake. The presence of fluorine atoms (in ZnPc 3) decreases the potency of this compound against B16F10 cancer cells due to its low cellular uptake and ability to generate 1O2 when compared to ZnPc 1 and ZnPc 2 (vide infra) (Shah and Westwell, 2007; Goslinski and Piskorz, 2011).
Photophysical Properties of Thiopyridinium Zn(II) Phthalocyanines
The photophysical properties depend on the nature and number of the peripheral substituents and in this case, by the presence/absence of a-F atoms in the Pc structure. The UV–Vis spectra of ZnPcs 1–3 showed absorption Soret bands at 388, 390, and 417 nm, respectively, and Q-band maxima from 686 to 720 nm in DMF solutions (Figure 1; Table 1), which is attributed, respectively, to the S0 → S2 and S0 → S1 transitions that are characteristic for phthalocyanines in the monomeric form. For the emission spectra of ZnPcs 1–3, obtained after excitation at λ = 660 nm, it was observed that the typical bands centered at 688 nm for ZnPcs 1 and 2 and 704 nm for ZnPc 3 relative to the S0 → S1 transitions. For ZnPc 2, two additional bands at ca. 730 nm were observed, most probably associated to the vibrational state transitions.
It is noteworthy that the compound ZnPc 1 was the one that showed a higher fluorescence quantum yield (ΦF = 0.23), followed by ZnPc 2 and ZnPc 3 (Table 1). Comparing the ФF values of ZnPc 3 and ZnPc, the reduction of ФF value for ZnPc 3 can be explained partially by the electron-withdrawing effects of the F atoms on their structure that increase the radiative decay rates. The number of thiopyridinium groups also influenced the ФF values: ZnPc 1 > ZnPc 2 > ZnPc 3.
Additionally, the photobleaching studies under the same conditions of the biological experiments were carried out. Following the UV–Vis analysis, the decrease in the characteristic phthalocyanine absorption band as a function of time upon exposure to irradiation was relatively small (data not shown), suggesting remarkable photostability for all compounds under red light irradiation as previously reported by some of us (Pereira et al., 2012), making the compounds attractive for PDT studies. Moreover, the cationic compounds of ZnPcs 1 and 2 evidence high solubility in water media due to the presence of four or eight positive charges. However, the ZnPc 3 with a-F on their macrocycle showed less solubility.
Upon light irradiation at an excitation wavelength of 660 nm, the ZnPcs in their excited state interact with molecular oxygen to generate ROS, especially 1O2 species. As can be seen in Figure 2, the production of 1O2 species was performed by monitoring their fluorescence emission at 1,270 nm. Singlet oxygen quantum yields (ФΔ) of ZnPcs 1–3 were determined and compared to the ZnPc as a standard reference, where a significant increase for the 1O2 generation for ZnPc 1 was observed. Moreover, the ΦΔ values of ZnPcs 1–3 followed the descending order, ZnPc 1 > ZnPc 2 > ZnPc 3 (Table 1), which are correlated with the number of thiopyridinium groups and presence/absence of a-F atoms (heavy atom effect) on the phthalocyanine backbone. In fact, the photophysical properties of the studied phthalocyanines could be affected by various molecular aspects, including extended π conjugation, structural distortion, and internal heavy atom. As expected, the fluorescence is quenched as shown in Table 1 due to the heavy atom effect of F atoms. On the other hand, the lowest ФD observed for ZnPc 3 maybe due to the combination of other effects resulting in low spin orbital coupling with consequent decreases in the triplet quantum yield. Since the triplet state decays at a relatively faster rate, the efficiency of oxygen quenching of the triplet state decreases. In addition, due to its slightly lower solubility compared to ZnPc 1 and ZnPc 2 because of interactions, fluor–fluor and fluor–hydrogen cannot be ruled out (Murray et al., 2021).
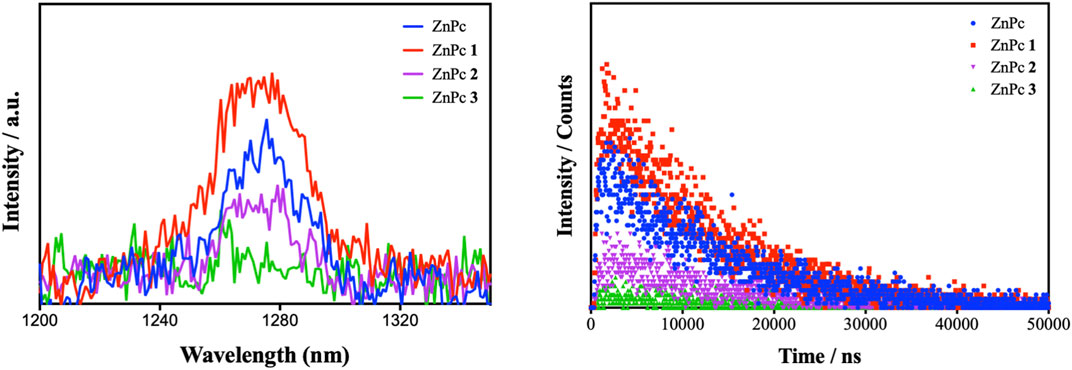
FIGURE 2. (A) Emission spectra resolved direct detection of 1O2 species and (B) time-resolved direct detection of 1O2 species generated by ZnPc reference and ZnPcs 1–3.
Photobiological Studies With B16F10 Melanoma Cells
Initially, the cytotoxicity studies of ZnPcs 1–3 were performed through cell viability of murine melanoma cells. The results are compiled in Figure 3 and show that the cellular viability is reduced ∼20–35% at higher concentrations (10 and 20 μM). These results suggest that ZnPcs 1–3 have a slight dark toxicity effect according to the concentration administered. However, the cytotoxic effect is slightly reduced at lower concentrations of 1 and 2 μM, and the cell viability is higher than 85%. Nevertheless, it was reported that the presence of 4-aminopyridine as the axial ligand in Ru(II) phthalocyanines induces a relative cytotoxicity effect against B16F10 cells due to their interaction with cell components (Martins et al., 2020). The cell viability decrease was significant at 1 μM (reduction in the cell viability = 62.6% compared to control); however, our results indicated an opposite effect because we observed a non-significant effect on this concentration.
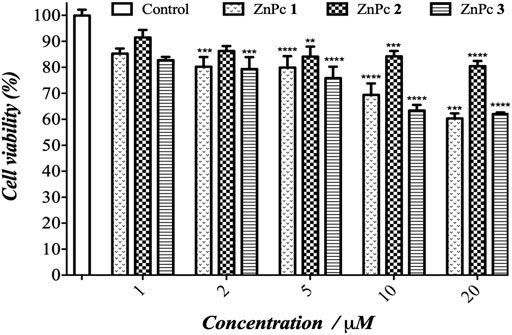
FIGURE 3. Dark cytotoxicity of ZnPcs 1–3 in B16F10 cells as a function of PS concentration. The results are presented as mean ± standard deviation. Statistical significance: **p < 0.01, ***p < 0.001, and ****p < 0.0001 vs. control.
The PDT effect of ZnPcs 1–3 is clearly evidenced in melanoma cells upon exposure to a light dose of 3 J/cm2 of red light (Figure 4). The cell viability is significantly reduced and indicates the occurrence of cell death. The PDT efficiency can be directly correlated to the singlet oxygen production, following the best performance order of ZnPc 1 > ZnPc 2 > ZnPc 3. Despite the presence of fluorine atoms increasing the lipophilicity of ZnPc 3, this PS showed the lowest ФΔ value. ZnPc 1 showed 3.5 to 10 times higher (photo)toxicity compared to ZnPc 3 under the same light conditions. The results of phototoxicity on B16F10 melanoma cells suggest that phthalocyanine phototoxic effects are also PS dose dependent. Notably, the ZnPc 1 caused a significant PDT response at lower concentrations (1–20 µM), showing a considerable viability reduction at 1 µM (79%), and reached the detection limit of the MTT method at the highest concentrations used (10–20 µM). The concentration dependence is evident for ZnPc 1 with the increase in cell death of 79.4–94.4% at a concentration of 1–20 μM at the light dose of 3 J/cm2, respectively. For ZnPc 3 (lowest ФΔ), the dependence on concentration is even more evident and the cell death was observed at 1 and 20 µM with a ratio of 26 and 53%, respectively.
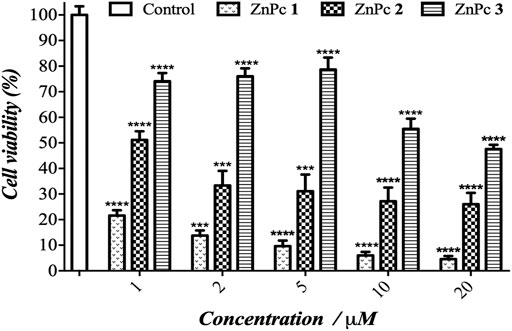
FIGURE 4. Photocytotoxicity of ZnPcs 1–3 against B16F10 cells as a function of PS concentration under red light and applying a light dose of 3 J/cm2. The results are presented as mean ± standard deviation. Statistical significance: ***p < 0.001 and ****p < 0.0001 vs. control. The control cells without PS were irradiated with the same conditions used in the PDT studies.
In fact, the obtained PDT results are surprisingly comparable to the ones with other photosensitizers reported in the literature (Woodburn et al., 1998; Sparsa et al., 2013; Ma et al., 2015; Aishwarya and Sanjay, 2018), especially with some of them clinically approved for various types of cancer by the Food and Drug Administration (e.g., Photofrin or 5-aminolevulinic acid (5-ALA)), so we conclude that the values are comparable with those reported (Woodburn et al., 1998; Sparsa et al., 2013; Ma et al., 2015; Aishwarya and Sanjay, 2018). In addition, it was observed that the remarkable cell viability reduction induced by ROS was as expected. Currently, some studies indicate that such kind of pigmented melanomas are unresponsive to PDT with Photofrin because of melanin interference. In turn, if we talk about how other therapeutic approaches are used with, e.g., cisplatin drugs, the viability reduction effect is higher compared to those described by us, however, with high secondary effects.
The photocytotoxicity of (5-ALA)-induced protoporphyrin IX (PpIX) accumulation against B16F10 cells varied as a function of PS concentration (light dose = 37 J/cm2, concentrations of 5-ALA of 0–20 mM) (Sparsa et al., 2013). Silva and co-workers showed that the complex cis-[Ru(H-dcbpy-)2(Cl) (NO)][Na4(Tb(TsPc) (acac)] (cis-[RuPc]) at 0.5 mM exhibited some dark toxicity (ca. 20%). However, when B16F10 cells were irradiated in the presence of the cis-[RuPc], the cell viability dropped significantly (ca. 80%). From our results in the B16F10 cells, it was observed that low doses of ZnPcs 1–3 are enough for PDT efficacy (Cicillini et al., 2009).
Recently, Silva and co-workers improved the PDT effectiveness through a combination with photobiomodulation (PBM) using ruthenium phthalocyanine (RuPc) as a PS drug. The use of PBM followed by the PDT approach has been previously described. The reactive oxygen and nitrogen species (RONS) and ROS production and cellular uptake justify the increased PDT efficiency. In these studies, low concentrations of RuPc were used (up 1.0 μM) against A375 melanoma cells at different light doses (1, 3, or 6 J/cm2) (Negri et al., 2019a). PDT assays were also performed for the B16F10 cells. The cell line was incubated for 24 h with 10 μM of RuPc, irradiated at 660 nm with a light dose of 8.9 J/cm2, and subsequently, resulted in 50% of cell death (Negri et al., 2019b). Nevertheless, it is worth mentioning that the in vitro results depend on the PS or cell line, incubation time, and other experimental conditions, such as the light dose in the case of PDT.
Tedesco and co-workers (Goto et al., 2017) reported the encapsulation of aluminium chloride phthalocyanine (ClAlPc) in solid lipid nanoparticles using the direct emulsification for PDT studies. Briefly, the authors noted a light dose dependency resulting in a cell viability decrease of 85% for the hybrid ClAlPc/SLN and 51% for the non-immobilized ClAlPc, both at 0.75 μg/ml and a light dose of 2 J/cm2.
Knowing that, the cytotoxic effect of a PS drug is also affected by the cellular internalization, where the fluorescence microscopy images of B16F10 cells treated with all the compounds were obtained (Figure 5). The cellular internalization of ZnPcs 1–3 was confirmed by fluorescence microscopy, and as shown in Figure 5, the characteristic red fluorescence of our compounds was observed. The overlay of fluorescence images with bright field showed that all compounds are distributed in the cells. In addition, the cellular uptake apparently increased according to the MTT assays: ZnPc 1 > ZnPc 3 > ZnPc 2. In fact, the PDT performance of PS increases with its cellular uptake, and the PS internalization varies according to its chemical and physical properties.
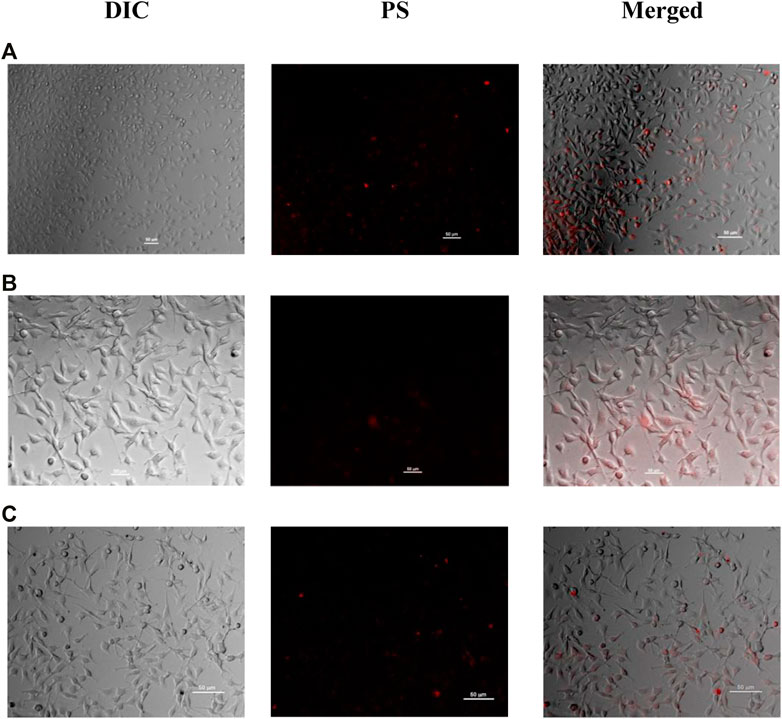
FIGURE 5. From left to right: differential interference contrast (DIC) and fluorescence microscopy images of B16F10 cells treated with (A) ZnPc 1, (B) ZnPc 2, and (C) ZnPc 3. The images show the distribution of the ZnPc dyes (10 μM) in B16F10 cells after incubation for 4 h.
Conclusion
The tetra- and octa-thiopyridinium ZnPcs 1–3 were evaluated as PS drugs against B16F10 melanoma cells. ZnPcs 1–3 were administered at different concentrations (1–20 μM) under dark and light conditions. The ZnPc 1 was able to photoinactivate B16F10 melanoma cells reaching the detection limit of the MTT method under red-light irradiation. In an attempt to justify the obtained results, the photophysical, photochemical, and in vitro photobiological properties were evaluated and correlated. The better performance observed for ZnPc 1 was directly correlated with its highest singlet oxygen production compared to the others: ZnPcs 2 and 3. The PDT results showed that the choice of the adequate phthalocyanine backbone modulates the photophysical and photochemical properties and, consequently, the performance of PDT. Moreover, the best PDT conditions were found for ZnPc 1 at a concentration of 20 µM, under red light (λ = 660 ± 20 nm) at an irradiance of 4.5 mW/cm2 for 667 s (light dose of 3 J/cm2). From the aforementioned data, the cationic ZnPc 1 can be considered an interesting PS agent to treat melanoma cancer cells, especially B16F10 ones.
Data Availability Statement
The raw data supporting the conclusion of this article will be made available by the authors, without undue reservation.
Author Contributions
KC: characterization, biological studies, and writing—original draft preparation, review, and editing. JP: characterization and biological studies. JB: characterization and biological studies. RS: validation, writing, review, and supervision. JT: writing, review, editing, and supervision. LL: phthalocyanine synthesis and characterization and writing original draft—review and editing. All authors contributed to writing the manuscript and approved the final version of the manuscript.
Conflict of Interest
The authors declare that the research was conducted in the absence of any commercial or financial relationships that could be construed as a potential conflict of interest.
Publisher’s Note
All claims expressed in this article are solely those of the authors and do not necessarily represent those of their affiliated organizations, or those of the publisher, the editors, and the reviewers. Any product that may be evaluated in this article, or claim that may be made by its manufacturer, is not guaranteed or endorsed by the publisher.
Acknowledgments
Thanks are due to Coordenação de Aperfeiçoamento de Pessoal de Nível Superior (CAPES), Conselho Nacional de Desenvolvimento Científico e Tecnológico (CNPq), and Fundação de Amparo à Pesquisa do Estado de São Paulo (FAPESP 2016/12707-0 and 2019/19448-8), Universidade de São Paulo. The authors thank Aveiro and Lisbon Universities, FCT/MCTES, for the financial support to LAQV-REQUIMTE (UIDB/50006/2020) and CQE (UIDB/00100/2020) research unities and to the FCT projects P2020-PTDC/QUI-QOR/31770/2017 and P2020-PTDC/QEQ-SUP/5355/2014, through national fonds (PIDDAC) and where applicable co-financed by the FEDER-Operational Thematic Program for Competitiveness and Internationalization-COMPETE 2020, within the PT2020 Partnership Agreement. K.A.D.F. Castro thanks CAPES for the post-doctoral scholarship granted (PNPD/CAPES). The authors would also like to thank Dr. Maurício da Silva Baptista (Cepid Redoxoma 2013/07937-8) from the Institute of Chemistry, University of São Paulo, for allowing them to use the laboratory and equipment, as well as for providing them the technical assistance of Dra. Helena Couto Junqueira.
References
Aishwarya, S., and Sanjay, K. R. (2018). Conjugation Study of 5-aminolevulinic Acid with Microbial Synthesized Gold Nanoparticles to Evaluate its Effect on Skin Melanoma and Epidermoid Carcinoma Cell Lines Using Photodynamic Cancer Therapy. Gold Bull. 51 (1-2), 11–19. doi:10.1007/s13404-017-0224-x
Ascierto, P. A., Kirkwood, J. M., Grob, J. J., Simeone, E., Grimaldi, A. M., Maio, M., et al. (2012). The Role of BRAF V600 Mutation in Melanoma. J. Transl Med. 10, 85. doi:10.1186/1479-5876-10-85
Camerin, M., Magaraggia, M., Soncin, M., Jori, G., Moreno, M., Chambrier, I., et al. (2010). The In Vivo Efficacy of Phthalocyanine-Nanoparticle Conjugates for the Photodynamic Therapy of Amelanotic Melanoma. Eur. J. Cancer 46 (10), 1910–1918. doi:10.1016/j.ejca.2010.02.037
Castro, K. A. D. F., Ramos, L., Mesquita, M., Biazzotto, J. C., Moura, N. M. M., Mendes, R. F., et al. (2021). Comparison of the Photodynamic Action of Porphyrin, Chlorin, and Isobacteriochlorin Derivatives toward a Melanotic Cell Line. ACS Appl. Bio Mater. 4, 4925–4935. doi:10.1021/acsabm.1c00218
Cicillini, S. A., Prazias, A. C. L., Tedesco, A. C., Serra, O. A., and da Silva, R. S. (2009). Nitric Oxide and Singlet Oxygen Photo-Generation by Light Irradiation in the Phototherapeutic Window of a Nitrosyl Ruthenium Conjugated with a Phthalocyanine Rare Earth Complex. Polyhedron 28 (13), 2766–2770. doi:10.1016/j.poly.2009.05.051
Czarnecka, A. M., Bartnik, E., Fiedorowicz, M., and Rutkowski, P. (2020). Targeted Therapy in Melanoma and Mechanisms of Resistance. Ijms 21 (13), 4576. doi:10.3390/ijms21134576
Ferreira, J. T., Pina, J., Ribeiro, C. A. F., Fernandes, R., Tomé, J. P. C., Rodríguez-Morgade, M. S., et al. (2020). Highly Efficient Singlet Oxygen Generators Based on Ruthenium Phthalocyanines: Synthesis, Characterization and In Vitro Evaluation for Photodynamic Therapy. Chemistry 26 (8), 1789–1799. doi:10.1002/chem.201903546
Galstyan, A. (2021). Turning Photons into Drugs: Phthalocyanine‐Based Photosensitizers as Efficient Photoantimicrobials. Chem. Eur. J. 27 (6), 1903–1920. doi:10.1002/chem.202002703
Gamelas, S. R. D., Gomes, A. T. P. C., Faustino, M. A. F., Tomé, A. C., Tomé, J. P. C., Almeida, A., et al. (2020). Photoinactivation of Escherichia coli with Water-Soluble Ammonium-Substituted Phthalocyanines. ACS Appl. Bio Mater. 3 (7), 4044–4051. doi:10.1021/acsabm.0c00128
Globocan. Melanoma of skin (2020). Available from https://gco.iarc.fr/today/data/factsheets/cancers/16-Melanoma-of-skin-fact-sheet.
Goslinski, T., and Piskorz, J. (2011). Fluorinated Porphyrinoids and Their Biomedical Applications. J. Photochem. Photobiol. C: Photochem. Rev. 12 (4), 304–321. doi:10.1016/j.jphotochemrev.2011.09.005
Goto, P. L., Siqueira-Moura, M. P., and Tedesco, A. C. (2017). Application of Aluminum Chloride Phthalocyanine-Loaded Solid Lipid Nanoparticles for Photodynamic Inactivation of Melanoma Cells. Int. J. Pharm. 518 (1), 228–241. doi:10.1016/j.ijpharm.2017.01.004
Gümrükçü, G., Karaoğlan, G. K., Erdoğmuş, A., Gül, A., and Avcıata, U. (2014). Photophysical, Photochemical, and BQ Quenching Properties of Zinc Phthalocyanines with Fused or Interrupted Extended Conjugation. J. Chem. 2014, 1–11. doi:10.1155/2014/435834
Halaskova, M., Rahali, A., Almeida-Marrero, V., Machacek, M., Kucera, R., Jamoussi, B., et al. (2021). Peripherally Crowded Cationic Phthalocyanines as Efficient Photosensitizers for Photodynamic Therapy. ACS Med. Chem. Lett. 12 (3), 502–507. doi:10.1021/acsmedchemlett.1c00045
Janas, K., Boniewska-Bernacka, E., Dyrda, G., and Słota, R. (2021). Porphyrin and Phthalocyanine Photosensitizers Designed for Targeted Photodynamic Therapy of Colorectal Cancer. Bioorg. Med. Chem. 30, 115926. doi:10.1016/j.bmc.2020.115926
Karunakaran, S. C., Babu, P. S. S., Madhuri, B., Marydasan, B., Paul, A. K., Nair, A. S., et al. (2013). In Vitro Demonstration of Apoptosis Mediated Photodynamic Activity and NIR Nucleus Imaging through a Novel Porphyrin. ACS Chem. Biol. 8 (1), 127–132. doi:10.1021/cb3004622
Kozar, I., Margue, C., Rothengatter, S., Haan, C., and Kreis, S. (2019). Many Ways to Resistance: How Melanoma Cells Evade Targeted Therapies. Biochim. Biophys. Acta (Bba) - Rev. Cancer 1871 (2), 313–322. doi:10.1016/j.bbcan.2019.02.002
Lo, P.-C., Rodríguez-Morgade, M. S., Pandey, R. K., Ng, D. K. P., Torres, T., and Dumoulin, F. (2020). The Unique Features and Promises of Phthalocyanines as Advanced Photosensitisers for Photodynamic Therapy of Cancer. Chem. Soc. Rev. 49 (4), 1041–1056. doi:10.1039/C9CS00129H
Lopes-Nunes, J., Carvalho, J., Figueiredo, J., Ramos, C. I. V., Lourenço, L. M. O., Tomé, J. P. C., et al. (2020). Phthalocyanines for G-Quadruplex Aptamers Binding. Bioorg. Chem. 100, 103920. doi:10.1016/j.bioorg.2020.103920
Lourenço, L. M. O., Neves, M. G. P. M. S., Cavaleiro, J. A. S., and Tomé, J. P. C. (2014). Synthetic Approaches to Glycophthalocyanines. Tetrahedron 70 (17), 2681–2698. doi:10.1016/j.tet.2014.01.058
Lourenço, L. M. O., Pereira, P. M. R., Maciel, E., Válega, M., Domingues, F. M. J., Domingues, M. R. M., et al. (2014). Amphiphilic Phthalocyanine-Cyclodextrin Conjugates for Cancer Photodynamic Therapy. Chem. Commun. 50 (61), 8363–8366. doi:10.1039/C4CC02226B
Lourenço, L. M. O., Rocha, D. M. G. C., Ramos, C. I. V., Gomes, M. C., Almeida, A., Faustino, M. A. F., et al. (2019). Photoinactivation of Planktonic and Biofilm Forms of Escherichia coli through the Action of Cationic Zinc(II) Phthalocyanines. ChemPhotoChem 3 (5), 251–260. doi:10.1002/cptc.201900020
Lourenço, L. M. O., Sousa, A., Gomes, M. C., Faustino, M. A. F., Almeida, A., Silva, A. M. S., et al. (2015). Inverted Methoxypyridinium Phthalocyanines for PDI of Pathogenic Bacteria. Photochem. Photobiol. Sci. 14 (10), 1853–1863. doi:10.1039/C5PP00145E
Ma, X., Qu, Q., and Zhao, Y. (2015). Targeted Delivery of 5-Aminolevulinic Acid by Multifunctional Hollow Mesoporous Silica Nanoparticles for Photodynamic Skin Cancer Therapy. ACS Appl. Mater. Inter. 7 (20), 10671–10676. doi:10.1021/acsami.5b03087
Mantareva, V., Petrova, D., Avramov, L., Angelov, I., Borisova, E., Peeva, M., et al. (2005). Long Wavelength Absorbing Cationic Zn(II)-phthalocyanines as Fluorescent Contrast Agents for B16 Pigmented Melanoma. J. Porphyrins Phthalocyanines 09 (01), 47–53. doi:10.1142/s1088424605000095
Marciel, L., Teles, L., Moreira, B., Pacheco, M., Lourenço, L. M., Neves, M. G., et al. (2017). An Effective and Potentially Safe Blood Disinfection Protocol Using Tetrapyrrolic Photosensitizers. Future Med. Chem. 9 (4), 365–379. doi:10.4155/fmc-2016-0217
Martins, T. J., Negri, L. B., Pernomian, L., Faial, K. D. C. F., Xue, C., Akhimie, R. N., et al. (2020). The Influence of Some Axial Ligands on Ruthenium-Phthalocyanine Complexes: Chemical, Photochemical, and Photobiological Properties. Front. Mol. Biosci. 7, 595830. doi:10.3389/fmolb.2020.595830
Murray, J. S., Seybold, P. G., and Politzer, P. (2021). The many Faces of Fluorine: Some Noncovalent Interactions of Fluorine Compounds. The J. Chem. Thermodynamics 156, 106382. doi:10.1016/j.jct.2020.106382
Negri, L. B., Martins, T. J., da Silva Gobo, N. R., de Oliveira, K. T., Hamblin, M. R., and da Silva, R. S. (2019). Design, Synthesis and Photobiological Activity of Novel Ruthenium Phthalocyanine Complexes. Inorg. Chem. Commun. 99, 60–63. doi:10.1016/j.inoche.2018.11.004
Negri, L. B., Martins, T. J., da Silva, R. S., and Hamblin, M. R. (2019). Photobiomodulation Combined with Photodynamic Therapy Using Ruthenium Phthalocyanine Complexes in A375 Melanoma Cells: Effects of Nitric Oxide Generation and ATP Production. J. Photochem. Photobiol. B: Biol. 198, 111564. doi:10.1016/j.jphotobiol.2019.111564
Pereira, J. B., Carvalho, E. F. A., Faustino, M. A. F., Fernandes, R., Neves, M. G. P. M. S., Cavaleiro, J. A. S., et al. (2012). Phthalocyanine Thio-Pyridinium Derivatives as Antibacterial Photosensitizers†. Photochem. Photobiol. 88 (3), 537–547. doi:10.1111/j.1751-1097.2012.01113.x
Pereira, N. A. M., Laranjo, M., Pina, J., Oliveira, A. S. R., Ferreira, J. D., Sánchez-Sánchez, C., et al. (2018). Advances on Photodynamic Therapy of Melanoma through Novel Ring-Fused 5,15-diphenylchlorins. Eur. J. Med. Chem. 146, 395–408. doi:10.1016/j.ejmech.2017.12.093
Pereira, P. M. R., Parada, B., Ribeiro-Rodrigues, T. M., Fontes-Ribeiro, C. A., Girão, H., Tomé, J. P. C., et al. (2020). Caveolin-1 Modulation Increases Efficacy of a Galacto-Conjugated Phthalocyanine in Bladder Cancer Cells Resistant to Photodynamic Therapy. Mol. Pharmaceutics 17 (6), 2145–2154. doi:10.1021/acs.molpharmaceut.0c00298
Rajkumar, S., and Watson, I. R. (2016). Molecular Characterisation of Cutaneous Melanoma: Creating a Framework for Targeted and Immune Therapies. Br. J. Cancer 115 (2), 145–155. doi:10.1038/bjc.2016.195
Revuelta‐Maza, M. Á., Heras, E., Agut, M., Nonell, S., Torres, T., and Torre, G. (2021). Self‐Assembled Binaphthyl‐Bridged Amphiphilic AABB Phthalocyanines: Nanostructures for Efficient Antimicrobial Photodynamic Therapy. Chem. Eur. J. 27 (15), 4955–4963. doi:10.1002/chem.202005060
Revuelta-Maza, M. Á., González-Jiménez, P., Hally, C., Agut, M., Nonell, S., de la Torre, G., et al. (2020). Fluorine-substituted Tetracationic ABAB-Phthalocyanines for Efficient Photodynamic Inactivation of Gram-Positive and Gram-Negative Bacteria. Eur. J. Med. Chem. 187, 111957. doi:10.1016/j.ejmech.2019.111957
Ribas, A., Algazi, A., Ascierto, P. A., Butler, M. O., Chandra, S., Gordon, M., et al. (2020). PD-L1 Blockade in Combination with Inhibition of MAPK Oncogenic Signaling in Patients with Advanced Melanoma. Nat. Commun. 11 (1), 6262. doi:10.1038/s41467-020-19810-w
Ribeiro, C. P. S., and Lourenço, L. M. O. (2021). Overview of Cationic Phthalocyanines for Effective Photoinactivation of Pathogenic Microorganisms. J. Photochem. Photobiol. C: Photochem. Rev. 48, 100422. doi:10.1016/j.jphotochemrev.2021.100422
Saenz, C., Cheruku, R. R., Ohulchanskyy, T. Y., Joshi, P., Tabaczynski, W. A., Missert, J. R., et al. (2017). Structural and Epimeric Isomers of HPPH [3-Devinyl 3-{1-(1-hexyloxy) Ethyl}pyropheophorbide-A]: Effects on Uptake and Photodynamic Therapy of Cancer. ACS Chem. Biol. 12 (4), 933–946. doi:10.1021/acschembio.7b00023
Savoia, P., Zavattaro, E., and Cremona, O. (2020). Clinical Implications of Acquired BRAF Inhibitors Resistance in Melanoma. Ijms 21 (24), 9730. doi:10.3390/ijms21249730
Shah, P., and Westwell, A. D. (2007). The Role of Fluorine in Medicinal Chemistry. J. Enzyme Inhib. Med. Chem. 22 (5), 527–540. doi:10.1080/14756360701425014
Siegel, R. L., Miller, K. D., Fuchs, H. E., and Jemal, A. (2021). Cancer Statistics, 2021. CA A. Cancer J. Clin. 71 (1), 7–33. doi:10.3322/caac.21654
Sparsa, A., Bellaton, S., Naves, T., Jauberteau, M.-O., Bonnetblanc, J.-M., Sol, V., et al. (2013). Photodynamic Treatment Induces Cell Death by Apoptosis or Autophagy Depending on the Melanin Content in Two B16 Melanoma Cell Lines. Oncol. Rep. 29 (3), 1196–1200. doi:10.3892/or.2012.2190
Sung, H., Ferlay, J., Siegel, R. L., Laversanne, M., Soerjomataram, I., Jemal, A., et al. (2021). Global Cancer Statistics 2020: GLOBOCAN Estimates of Incidence and Mortality Worldwide for 36 Cancers in 185 Countries. CA A. Cancer J. Clin. 71 (3), 209–249. doi:10.3322/caac.21660
Valli, F., García Vior, M. C., Roguin, L. P., and Marino, J. (2019). Oxidative Stress Generated by Irradiation of a Zinc(II) Phthalocyanine Induces a Dual Apoptotic and Necrotic Response in Melanoma Cells. Apoptosis 24 (1-2), 119–134. doi:10.1007/s10495-018-01512-w
Valli, F., García Vior, M. C., Roguin, L. P., and Marino, J. (2020). Crosstalk between Oxidative Stress-Induced Apoptotic and Autophagic Signaling Pathways in Zn(II) Phthalocyanine Photodynamic Therapy of Melanoma. Free Radic. Biol. Med. 152, 743–754. doi:10.1016/j.freeradbiomed.2020.01.018
van Straten, D., Mashayekhi, V., de Bruijn, H. S., Oliveira, S., and Robinson, D. J. (2017). Oncologic Photodynamic Therapy: Basic Principles, Current Clinical Status and Future Directions. Cancers (Basel) 9 (2), 19. doi:10.3390/cancers9020019
Vasan, N., Baselga, J., and Hyman, D. M. (2019). A View on Drug Resistance in Cancer. Nature 575 (7782), 299–309. doi:10.1038/s41586-019-1730-1
Vummidi, B. R., Noreen, F., Alzeer, J., Moelling, K., and Luedtke, N. W. (2013). Photodynamic Agents with Anti-metastatic Activities. ACS Chem. Biol. 8 (8), 1737–1746. doi:10.1021/cb400008t
Welsh, S. J., Rizos, H., Scolyer, R. A., and Long, G. V. (2016). Resistance to Combination BRAF and MEK Inhibition in Metastatic Melanoma: Where to Next? Eur. J. Cancer 62, 76–85. doi:10.1016/j.ejca.2016.04.005
Wibmer, L., Lourenço, L. M. O., Roth, A., Katsukis, G., Neves, M. G. P. M. S., Cavaleiro, J. A. S., et al. (2015). Decorating Graphene Nanosheets with Electron Accepting Pyridyl-Phthalocyanines. Nanoscale 7 (13), 5674–5682. doi:10.1039/C4NR05719H
Winder, M., and Virós, A. (2018). Mechanisms of Drug Resistance in Melanoma. Handb Exp. Pharmacol. 249, 91–108. doi:10.1007/164_2017_17
Woodburn, K. W., Fan, Q., Kessel, D., Luo, Y., and Young, S. W. (1998). Photodynamic Therapy of B16F10 Murine Melanoma with Lutetium Texaphyrin. J. Invest. Dermatol. 110 (5), 746–751. doi:10.1046/j.1523-1747.1998.00182.x
Zhang, X.-J., Han, G.-Y., Guo, C.-Y., Ma, Z.-Q., Lin, M.-Y., Wang, Y., et al. (2020). Design, synthesis and biological evaluation of novel 31-hexyloxy chlorin e6-based 152- or 131-amino acid derivatives as potent photosensitizers for photodynamic therapy. Eur. J. Med. Chem. 207, 112715. doi:10.1016/j.ejmech.2020.112715
Keywords: photodynamic therapy (PDT), photosensitizer, cationic phthalocyanine, melanoma, cancer cells, in vitro assay
Citation: Castro KADF, Prandini JA, Biazzotto JC, Tomé JPC, da Silva RS and Lourenço LMO (2022) The Surprisingly Positive Effect of Zinc-Phthalocyanines With High Photodynamic Therapy Efficacy of Melanoma Cancer. Front. Chem. 10:825716. doi: 10.3389/fchem.2022.825716
Received: 30 November 2021; Accepted: 15 February 2022;
Published: 14 March 2022.
Edited by:
Dinorah Gambino, Universidad de la República, UruguayReviewed by:
Muthumuni Managa, University of South Africa, South AfricaEdith Amuhaya, United States International University-Africa, Kenya
Copyright © 2022 Castro, Prandini, Biazzotto, Tomé, da Silva and Lourenço. This is an open-access article distributed under the terms of the Creative Commons Attribution License (CC BY). The use, distribution or reproduction in other forums is permitted, provided the original author(s) and the copyright owner(s) are credited and that the original publication in this journal is cited, in accordance with accepted academic practice. No use, distribution or reproduction is permitted which does not comply with these terms.
*Correspondence: Roberto S. da Silva, c2lsdmFAdXNwLmJy; Leandro M. O. Lourenço, bGVhbmRyb2xvdXJlbmNvQHVhLnB0