- 1Jiangsu Engineering and Technology Research Center of VOCs Treatment, Environmental Engineering College, Nanjing Polytechnic Institute, Nanjing, JS, China
- 2International Collaborative Laboratory of 2D Materials for Optoelectronics Science and Technology of Ministry of Education, Institute of Microscale Optoelectronics, Shenzhen University, Shenzhen, China
- 3Key Laboratory of Mesoscopic Chemistry of MOE, Jiangsu Provincial Key Laboratory of Vehicle Emissions Control, School of Chemistry and Chemical Engineering, Nanjing University, Nanjing, China
CO2 emission caused by fuel combustion and human activity has caused severe climate change and other subsequent pollutions around the world. Carbon neutralization via various novel technologies to alleviate the CO2 level in the atmosphere has thus become one of the major topics in modern research field. These advanced technologies cover CO2 capture, storage and conversion, etc., and electrocatalytic CO2 reduction reaction (CO2RR) by heterogeneous catalysts is among the most promising methods since it could utilize renewable energy and generate valuable fuels and chemicals. Covalent organic frameworks (COFs) represent crystalline organic polymers with highly rigid, conjugated structures and tunable porosity, which exhibit significant potential as heterogeneous electrocatalysts for CO2RR. This review briefly introduces related pioneering works in COF-based materials for electrocatalytic CO2RR in recent years and provides a basis for future design and synthesis of highly active and selective COF-based electrocatalysts in this direction.
Introduction
In modern societies, human activities and the development of industries primarily depend on fossil fuel combustion as energy source. One severe consequence of excessive consumption of fossil fuels is the considerable large amount of CO2 emission which is the major cause of greenhouse effect, climate change, and many other subsequent worldwide pollution issues (Bauer et al., 2016). It was reported that the global CO2 concentration in the atmosphere has increased from 353 to 409 ppm in the past 30 years and is predicted to reach over 600 ppm within this century (Mikkelsen et al., 2010; Yang et al., 2020).
To meet the demand of carbon neutralization and carbon recycling, a myriad of sustainable technologies have been developed to mitigate the level of atmospheric CO2, including CO2 capture, storage, and transferring CO2 into other useful carbonaceous molecules (Aresta et al., 2014; Yi et al., 2015; Liu et al., 2016; Abanades et al., 2017; Majumdar and deutch, 2018; Zheng et al., 2018). Photocatalysis, electrocatalysis, biological transformations, chemical fixation, and hydrogenation of CO2 have been extensively developed in the recent decades (Halmann, 1978; Tu et al., 2014; Duan et al., 2017; Kattel et al., 2017; Nguyen et al., 2018).
Among these, electrocatalytic CO2 reduction reaction (CO2RR) has been regarded as one of the most attractive methods to address the issue of renewable utilization of CO2 (Kuhl et al., 2012; Kondratenko et al., 2013; Al-Omari et al., 2018; Bushuyev et al., 2018; Chen et al., 2018; Tackett et al., 2019; Zhang M.-D. et al., 2020; Franco et al., 2020; Sun et al., 2020; Yang et al., 2020; Liang et al., 2021). This could be attributed to many reasons. First, given the advantages of electrocatalysis, the ease of controllability of the reaction rate and selectivity via applied potential current density makes CO2RR possible to be carried out under ambient conditions. Second, pure or a mixture of valuable C1/C2 carbon feedstocks such as CO, CH4, formic acid, or ethylene could be generated directly from electrochemical CO2RR as the main products via some multi-electron multi-proton pathways (Liu et al., 2015). Moreover, utilization of renewable energy (solar and windy) as the electricity source could fulfill the carbon cycle without emitting additional CO2 (Kumar et al., 2016). In addition, it is also feasible to scale up the electrochemical reaction system for mass productions (Qiao et al., 2014).
Despite these advantages, electrocatalytic CO2RR still suffers several technological obstacles, limiting the pace of its further development (Pletcher, 2018; Ross et al., 2019). The high C=O bond energy (805 kJ mol−1) makes the CO2 molecules chemically inert and thermodynamically stable (Glockler, 1958; Gottle and Koper, 2017). Thus, large activation energy is required to cleave the C=O bond with one electron and reorganize the linear molecule to its bent form in the electrocatalytic CO2RR process (Wang et al., 2018b). As a result, a highly negative reduction potential of −1.90 V (vs. SHE) is required for a single-electron reduction of CO2 on a non-catalytic electrode (Schwarz and Dodson, 1989). In addition, selectivity of products is another challenge of electrocatalytic CO2RR (Li et al., 2018; Zheng et al., 2019). Since it is usually carried out in aqueous protic media, a severe hydrogen evolution reaction (HER) at 0 V (vs. SHE) and some other side reactions will strongly interfere with the selected product formation and lower the Faraday efficiency (FE) (Zhou and Sun, 2017). To overcome these drawbacks, the creative design and synthesis of electrocatalysts with defined and stable structures along with excellent catalytic activity, product selectivity, and long-term durability are in urgent demand.
Covalent organic frameworks (COFs) have emerged as a novel type of porous crystalline polymer materials devised for various functions (Côté et al., 2005; Feng et al., 2012; Ding and Wang, 2013; Huang et al., 2016; Segura et al., 2016; Bisbey and Dichtel, 2017; Lohse and Bein, 2018; Chen et al., 2020; Geng et al., 2020). This type of reticular porous covalent frameworks is typically constructed by various organic building blocks consisting of only light nonmetal elements such as carbon, nitrogen, boron, and oxygen, which are uniformly linked by covalent bonds (Uribe-Romo et al., 2009). Compared to other porous polymer materials, COFs possess many unique features. Depending on the spatial geometric structures and functionalities of the building blocks and linkage molecules, the frameworks display rigid and highly ordered structures with 2D layer or 3D network topologies. COFs also have tunable porosity, which renders them extremely large accessible surface area and abundant active sites. Given these extraordinary characteristics, the COF-based materials have found wide applications in myriads of applications such as gas storage and separation, catalysis, photovoltaics, optoelectronics, drug delivery, and energy storage since the first report by Yaghi’s group in 2005.
In addition, since most of the COFs are synthesized via cross-coupling or condensation reactions between organic aromatic building blocks and linkers, the whole framework has large conjugation structures and shows semiconducting properties with tunable bandgaps, which renders them good platforms for electrocatalysis (Dogru and Bein, 2014; Lin and Chen, 2018; Lin et al., 2018; Song et al., 2019; Cui et al., 2020; Sharma et al., 2020; Guan et al., 2021; Zhao et al., 2021; Lee et al., 2022). To further improve the conductivity, COFs could be hybridized with other conductive materials such as graphene or carbon nanotubes, and the incorporation of transition metal active sites further enhances the catalytic activity and selectivity due to synergic effect between metals and COF supports. Indeed, recent years have witnessed much progress in the design and synthesis of COF-based materials for various electrocatalytic reactions including oxygen reduction and evolution reactions (ORR and OER), hydrogen evolution reaction (HER), and especially CO2 reduction (CO2RR) for the intention of energy conversion and fuel generation. Moreover, the large and tunable porous structures of COFs promote the diffusion and adsorption of CO2, and desorption of the resulting products, making them ideal electrocatalysts for CO2RR.
Despite the current progress, the development of COF-based electrocatalysts for CO2RR is still in its infancy stage. Herein, we will briefly review some of the seminal and representative work in this field. Although several previous reviews have come out dealing with the electrocatalytic CO2RR by COFs, focus in this work will be on the ingenuity of the design of electrocatalytic COF-based materials, and discussions will be divided by the types of high-value fuel products. In the end, we will also suggest the current challenges associated with and direction for future development of highly efficient COF-based electrocatalysts toward CO2 conversion.
CO2 reduction to CO
From a mechanistic perspective, the CO2RR theoretically involves one-, two-, four-, six-, or eight-electron reduction pathways. However, the most common product described in practical experiments in the mainstream studies of electrochemical CO2RR is CO, which is the simplest form of the reduction product via a two-electron transfer pathway.
In 2015, Yaghi et al. first reported the modular optimization of covalent organic frameworks for the electrochemical reduction of CO2 to CO (Lin et al., 2015). In this pioneering work, the authors synthesized two types of COFs (COF-366-Co and COF-367-Co) by reacting a molecular cobalt catalyst 5,10,15, and 20-tetrakis(4-aminophenyl)porphinato]cobalt Co(TAP) with two dicarboxaldehydes BDA and BPDA via imine condensation (Figure 1A). Characterizations demonstrated a stacking 2D sheet configuration consisting of 1D channels. In terms of electrocatalytic activities of CO2 reduction to CO, the catalysts exhibited high Faraday efficiency over 90% and turnover frequencies of 9,400 hour−1 at the overpotential of −0.55 V in the neutral aqueous condition. This activity was a 26-fold improvement compared to the sole molecular cobalt catalyst, and the COF catalysts could stand at least 24 h. The activity enhancement was attributed to the electronic structure of cobalt catalytic centers within the framework as revealed by X-ray absorption. In a later work by the same group, the electronic properties of 2D framework COF-366-Co were tuned by introducing electron-donating (-OMe) or electron-withdrawing (-F) groups on the BDA linker motif in the parent framework (Diercks et al., 2018). Electrocatalytic measurements showed that both catalytic activity and selectivity are further improved with the optimized 366-F-Co catalyst. X-ray absorption measurement revealed the synergetic effect between the inductive functionalities and the metal catalytic centers. Deng et al. further developed both 2D and 3D reticular COF-300-AR and COF-366-M-AR by directly reducing the corresponding parent COFs COF-300 (a previously reported COF synthesized with tetra(4-aminophenyl)methane (TAPM) and BDA via imine condensation) and COF-366-M with amine linkages (Liu et al., 2018). The amino groups turned out to improve both the crystallinity and chemical stability of COFs in acidic or basic environment, and FEs of CO conversion are 53% at −0.70 V and 80% at −0.85 V. Mechanistic studies showed the existence of carbamate intermediates facilitated by the amino groups during the activation of CO2. These consecutive works established the foundation of COF electrocatalysts for CO2RR.
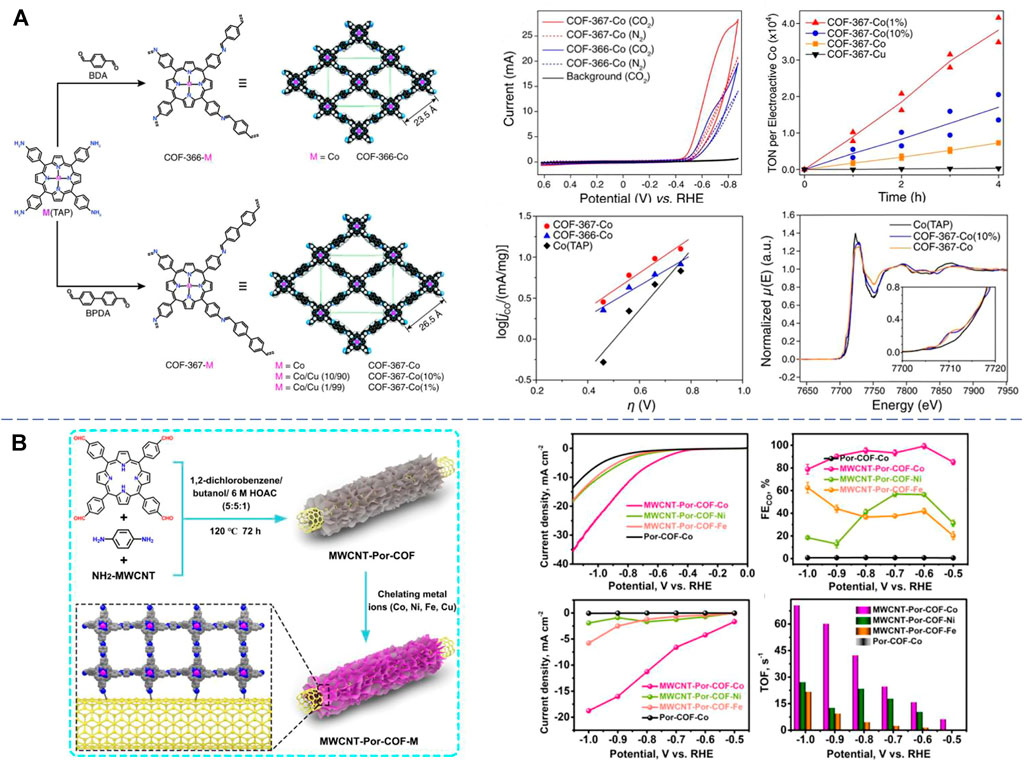
FIGURE 1. (A) Structures, electrochemical characterizations, and carbon dioxide reduction performance of the metalloporphyrin-derived 2D covalent organic frameworks COF-366-Co and COF-367-Co [reproduced from Lin et al. (2015) with the permission of AAAS]. (B) Design and synthesis of MWCNT-Por-COF-M and its electrochemical characterizations and carbon dioxide reduction performance [reproduced from Dong et al. (2022) with the permission of Elsevier].
COFs with phthalocyanine (Pc) centers often enable excellent electron transfer in the framework. Gao et al. first developed a series of cobalt-COFs constituted by molecular cobalt–phthalocyanine and boronic acid linkers via esterification reactions (Yao et al., 2018). Owing to the integration of storage function of the optimized Co-Pc-PBBA, the electrocatalytic reduction was carried out in the CO2 gas phase, which led to a nearly 100-fold CO2 concentration compared to the normal aqueous phase. The overpotential was considerably lowered, and this brought rate enhancement of CO production. Jiang et al. later synthesized a similar COF with cobalt phthalocyanine center and tetraone linkage via imine condensation (Huang et al., 2020). As a result, the whole 2D lattice exhibited full π conjugation along both x and y axes. The obtained Co-Pc-PDQ showed a Faraday efficiency of over 96% with an exceptional long-term turnover frequency of over 11,400 h−1, giving a 32-fold improvement over molecular catalysts. A similar Ni-Pc-PT conductive COF 2D nanosheet later developed by Cao et al. also provided over 93% high CO selectivity under a wide overpotential range of −0.60 to −1.10 V with 35 mA cm−1 current density in aqueous solution (Zhang M.-D. et al., 2020). Lan et al. designed and synthesized a series of COFs from cobalt and nickel phthalocyanine and tetrafluorophthalonitrile via dioxin linkers to improve stability (Lu et al., 2021). Outstanding Faraday efficiencies of over 96% were achieved in the electrocatalytic reduction of CO2 to CO, and when the system is coupled with photo irradiation, both the FE and current density were further improved. Two-dimensional polyimide-linked phthalocyanine COFs CoPc-PI-COF-1 and CoPc-PI-COF-2 developed by Jiang et al. showed great stability in strong acid aqueous solution and 87%–97% FE at the overpotentials between −0.60 and −0.90 V in the basic aqueous phase (Han et al., 2021).
The covalent triazine framework (CTF) represents a specific class of COF constitution. The building block molecules are connected by the cyano groups on their structures forming six-membered triazine rings, and in this case, no other reactants or linkers are necessary. Zhuang et al. developed a Ni porphyrin-based covalent triazine framework (NiPor-CTF) for electrocatalytic CO2 reduction (Lu et al., 2019). Still, remarkable results were obtained with an extremely high current density of 52.9 mA cm−1. Density function theory calculation suggested the key step of *CO2 transition to *COOH on the atomically dispersed NiN4 active centers. A rationally designed covalent triazine framework CTF-B featuring CuN2Cl2 active sites not only can convert CO2 to CO but also exhibits electrocatalytic selectivity for C2 products such as acidic acid and ethylene with a FE of 68.4% in total (Ma et al., 2020).
Sulfur-containing aldehyde linker molecules such as tetrathiafulvalene (TTF) and thieno[3,2-b]thiophene-2,5-dicarbaldehyde (TT) were also exploited to constitute COFs with porphyrins to further improve the conductivity. These sulfur-containing linkers could serve as electron carriers and form oriented electron transfer pathways with metalloporphyrin active sites during the electrocatalytic process. Related works by Lan et al. and Cao et al., respectively, demonstrate Co TT or TTF COFs giving exceptional results, paving a new way for constructing novel COFs for electrocatalysis (Zhu et al., 2020; Wu et al., 2021).
In addition to these, several other COFs for the highly efficient electrocatalytic CO2 reduction to CO in recent years were also worth mentioning. These unique structures include a single Mn atom loaded on bipyridyl-based COFs (COFbpyMn) via post-modification (Dubed Bandomo et al., 2021), a crown ether B18C6 embedded Co(TAPP)-COF (An et al., 2021), and a zwitterionic ultrathin cobalt tetraamino phthalocyanine-squaraine based COFs (COP-SA) (Song et al., 2021). All these ingenuous works guide the rational design of novel COF electrocatalysts for CO production from CO2.
CO2 reduction to hydrocarbon fuels
Although electrochemical CO2 reduction reaction catalyzed by a variety of conductive COF-based materials has been intensively investigated and remarkable results have been achieved during the past decade, an obvious bottleneck still exists in the type of reaction. As mentioned in the previous section, most of the COF-based catalysts direct the reaction to produce two-electron transferred product CO which is generated almost exclusively during the reaction. In these cases, the reduction of CO2 stays at the stage of CO at the active sites of COF catalysts, especially those with the metalloporphyrin active centers. However, hydrocarbon fuels with higher added value such as CH4, C2H4, and other deeply reduced multicarbon (C2+) products generated in CO2RR by COFs were barely reported. From a mechanistic viewpoint, the generation of these hydrocarbon fuels requires multiple electron (>2) transfer, and this process should be coupled with proton transfer. Thus, innovative COF catalysts for electrochemical CO2RR that are designed to meet these requirements remain a major challenge in this field.
A seminal work by Wen et al. first reported a metal-free perfluorinated covalent triazine framework (CTF)-based electrocatalysts FN-CTF-400 for highly selective CO2 conversion to CH4 with a 99.3% Faradaic efficiency in aqueous condition (Wang et al., 2018). The covalent triazine frameworks are featured in their ultralarge surface area and readily accessible of tuning surface functionalities by introducing heteroatom doping. Density functional theory calculations demonstrated that the high selectivity of CH4 production in the electrochemical CO2RR was probably attributed to the synergistic effect of doped F and N atoms in FN-CTF-400. Later, Huang and Cao et al. also devised copper nanoparticles (Cu NPs) immobilized on the imidazolium-functionalized covalent triazine framework (Cu/ICTF) to produce C2H4 in electrochemical CO2RR since Cu-based catalysts have been often utilized for generating multiple electron transfer C2+ products with relatively high selectivity (Mao et al., 2020). The imidazolium groups in ICTF could both improve the capture and activation of CO2 and thus lower the reaction energy barrier. Moreover, the Cu NPs are covalently linked and stabilized on the in situ formed N-heterocyclic carbenes (NHC) to form atomically dispersed active centers to prevent their deactivation. Cu/ICTF demonstrated a 35% high selectivity of C2H4 in CO2 electrochemical reduction along with a large current density of 4.14 mA cm−2 and an exceptional stability over 10 h.
In addition to the self-supported CTF, a series of well-defined nanostructures were also applied as supports of transition metal COF-based CO2RR electrocatalysts. Carbon nanotubes (CNTs) vertically connected with porphyrin-based covalent organic frameworks (Por-COF) nanosheets by covalent bonds have been developed for electrocatalytic CO2 reduction reaction to achieve CH4 (Dong et al., 2022). In this case, the CNT functions as both carriers to uniformly disperse Por-COF and controllers to facilitate the electron transfer pathway between porphyrin planes and metal active centers. As results, the MWCNT-Por-COF-Cu displayed high selectivity for CH4 with a FE of 71.2% under a flow-cell condition, which could be attributed to the in situ generated Cu-based nanoclusters during the electrocatalytic process. Meanwhile, the MWCNT-Por-COF-Co exhibited a remarkable catalytic activity with a FE high up to 99.3% at −0.60 V for CO production, a current density of 18.77 mA cm−2, and TOF up to 70.6 s−1 (Figure 1B). Lan et al. also designed tunable 1D nanofibers (NFs) and hollow tubes (HTs) as COF materials (Liu et al., 2021). These anthraquinone-based (AAn) COFs with superstructures could be post-modified with various transition metals for CH4 production, and the obtained AAn-COF-Cu (NF) and OH-AAn-COF-Cu (HT) exhibit exceptional Faraday efficiencies of 77% (128.1 mA cm−2, 0.90 V) and 61% (99.5 mA cm−2, 1.00 V), under flow-cell condition, respectively, the former of which is the highest among all the reported COF catalysts for CO2RR.
Carbon materials such as graphene oxide (GO) were also applied as linker molecules for building the 3D graphene framework (CGF) because of its easy accessibility, good conductivity, and functionalities. The CGF-based cobalt porphyrin catalysts developed by Bettelheim et al. exhibited a FE of about 20% for CH4 in CO2RR due to the strong irreversible adsorption of CO2 and stabilization of a key intermediate afforded by the free amine groups on the graphene (Bochlin et al., 2021).
Summary and perspectives
Electrocatalytic CO2 reduction reaction is currently regarded as one of the most efficient and straightforward technologies for alleviating the CO2 level in the atmosphere utilizing renewable energy and closing carbon cycle. Although various homogeneous and heterogeneous catalysts have been invented to improve the catalytic activity and product selectivity in electrocatalytic CO2RR, challenges associated with these catalysts also exist for the COF-based electrocatalysts. Therefore, this promising research field still awaits further development, and future devotion in this direction should continue to focus on improving the catalytic activities and product selectivities under various reaction conditions.
To be more specific, given the studies in recent years, it is obvious that the COF-based electrocatalysts for CO2RR still have problems such as poor electrical conductivity, few types of active centers, and the target product is mainly CO (Figure 2). Although the conductivity of electrocatalysts can be improved by compounding COFs with conductive materials such as graphene and CNT, we feel that the investigation of COF materials with inherent high conductivity for CO2RR can be more interesting. In addition, it is worth noting that most of the active centers of the COF-based electrocatalysts are in M-N4 structures (such as metalloporphyrin and metallophthalocyanine), which have been widely considered to exhibit excellent catalytic performance in electrocatalytic reactions. Exploring other types of active centers with different metal centers and building blocks would be another potential direction. Furthermore, in the electrocatalytic CO2RR by COF-based electrocatalysts, the obtained target product is primarily CO, while the high value-added hydrocarbons are more difficult to be obtained. Future studies looking deeper into the CO2RR mechanism may be conducive to the development of efficient catalysts for the production of hydrocarbon products. Last, since the COF-based electrocatalysts have demonstrated excellent performance toward CO2 adsorption and conversion, one step further could be taken by considering how to apply these COF electrocatalysts in organic synthesis exploiting CO2 via its reduction and insertion, and produce more high-value fine chemicals. We hope this review could offer new insights in the field of electrocatalytic CO2RR and pave ways for the design of more innovative COF-based electrocatalysts in the future.
Data availability statement
The original contributions presented in the study are included in the article/Supplementary Material. Further inquiries can be directed to the corresponding author.
Author contributions
All authors listed have made a substantial, direct, and intellectual contribution to the work and approved it for publication.
Funding
This work was supported by the National Natural Science Foundation of China (Grant No. 22002058) and Nanjing Polytechnic Institute (NJPI-RC-2022-02).
Conflict of interest
The authors declare that the research was conducted in the absence of any commercial or financial relationships that could be construed as a potential conflict of interest.
Publisher’s note
All claims expressed in this article are solely those of the authors and do not necessarily represent those of their affiliated organizations, or those of the publisher, the editors, and the reviewers. Any product that may be evaluated in this article, or claim that may be made by its manufacturer, is not guaranteed or endorsed by the publisher.
References
Abanades, J. C., Rubin, E. S., Mazzotti, M., and Herzog, H. J. (2017). On the climate change mitigation potential of CO2 conversion to fuels. Energy Environ. Sci. 10 (12), 2491–2499. doi:10.1039/c7ee02819a
Al-Omari, A. A., Yamani, Z. H., and Nguyen, H. L. (2018). Electrocatalytic CO2 reduction: From homogeneous catalysts to heterogeneous-based reticular Chemistry. Molecules 23 (11), 2835. doi:10.3390/molecules23112835
An, S., Lu, C., Xu, Q., Lian, C., Peng, C., Hu, J., et al. (2021). Constructing catalytic crown ether-based covalent organic frameworks for electroreduction of CO2. ACS Energy Lett. 6 (10), 3496–3502. doi:10.1021/acsenergylett.1c01681
Aresta, M., Dibenedetto, A., and Angelini, A. (2014). Catalysis for the valorization of exhaust carbon: From CO2 to chemicals, materials, and fuels. Technological use of CO2. Chem. Rev. 114 (3), 1709–1742. doi:10.1021/cr4002758
Bauer, N., Mouratiadou, I., Luderer, G., Baumstark, L., Brecha, R. J., Edenhofer, O., et al. (2016). Global fossil energy markets and climate change mitigation – an analysis with REMIND. Clim. Change 136 (1), 69–82. doi:10.1007/s10584-013-0901-6
Bisbey, R. P., and Dichtel, W. R. (2017). Covalent organic frameworks as a platform for multidimensional polymerization. ACS Cent. Sci. 3 (6), 533–543. doi:10.1021/acscentsci.7b00127
Bochlin, Y., Ezuz, L., Kadosh, Y., Benjamin, D., Mordekovitz, Y., Hayun, S., et al. (2021). Enhancement of electrocatalytic CO2 reduction to methane by CoTMPyP when hosted in a 3D covalent graphene framework. ACS Appl. Energy Mat. 4 (9), 10033–10041. doi:10.1021/acsaem.1c01978
Bushuyev, O. S., De Luna, P., Dinh, C. T., Tao, L., Saur, G., van de Lagemaat, J., et al. (2018). What should we make with CO2 and how can we make it? Joule 2 (5), 825–832. doi:10.1016/j.joule.2017.09.003
Chen, C., Kotyk, J. F. K., and Sheehan, S. W. (2018). Progress toward commercial application of electrochemical carbon dioxide reduction. Chem 4 (11), 2571–2586. doi:10.1016/j.chempr.2018.08.019
Chen, X., Geng, K., Liu, R., Tan, K. T., Gong, Y., Li, Z., et al. (2020). Covalent organic frameworks: Chemical approaches to designer structures and built-in functions. Angew. Chem. Int. Ed. 59 (13), 5050–5091. doi:10.1002/anie.201904291
Côté, A., Benin, A., Ockwig, N., O'Keeffe, M., Matzger, A., Yaghi, O., et al. (2005). Porous, crystalline, covalent organic frameworks. Science 310 (5751), 1166–1170. doi:10.1126/science.1120411
Cui, X., Lei, S., Wang, A. C., Gao, L., Zhang, Q., Yang, Y., et al. (2020). Emerging covalent organic frameworks tailored materials for electrocatalysis. Nano Energy 70, 104525. doi:10.1016/j.nanoen.2020.104525
Diercks, C. S., Lin, S., Kornienko, N., Kapustin, E. A., Nichols, E. M., Zhu, C., et al. (2018). Reticular electronic tuning of porphyrin active sites in covalent organic frameworks for electrocatalytic carbon dioxide reduction. J. Am. Chem. Soc. 140 (3), 1116–1122. doi:10.1021/jacs.7b11940
Ding, S.-Y., and Wang, W. (2013). Covalent organic frameworks (COFs): From design to applications. Chem. Soc. Rev. 42 (2), 548–568. doi:10.1039/C2CS35072F
Dogru, M., and Bein, T. (2014). On the road towards electroactive covalent organic frameworks. Chem. Commun. 50 (42), 5531–5546. doi:10.1039/C3CC46767H
Dong, H., Lu, M., Wang, Y., Tang, H.-L., Wu, D., Sun, X., et al. (2022). Covalently anchoring covalent organic framework on carbon nanotubes for highly efficient electrocatalytic CO2 reduction. Appl. Catal. B Environ. 303, 120897. doi:10.1016/j.apcatb.2021.120897
Duan, X. C., Xu, J. T., Wei, Z. X., Ma, J. M., Guo, S. J., Wang, S. Y., et al. (2017). Metal-free carbon materials for CO2 electrochemical reduction. Adv. Mat. 29 (41), 1701784. doi:10.1002/adma.201701784
Dubed Bandomo, G. C., Mondal, S. S., Franco, F., Bucci, A., Martin-Diaconescu, V., Ortuño, M. A., et al. (2021). Mechanically constrained catalytic Mn(CO)3Br single sites in a two-dimensional covalent organic framework for CO2 electroreduction in H2O. ACS Catal. 11 (12), 7210–7222. doi:10.1021/acscatal.1c00314
Feng, X., Ding, X., and Jiang, D. (2012). Covalent organic frameworks. Chem. Soc. Rev. 41 (18), 6010. doi:10.1039/C2CS35157A
Franco, F., Rettenmaier, C., Jeon, H. S., and Roldan Cuenya, B. (2020). Transition metal-based catalysts for the electrochemical CO2 reduction: From atoms and molecules to nanostructured materials. Chem. Soc. Rev. 49 (19), 6884–6946. doi:10.1039/D0CS00835D
Geng, K., He, T., Liu, R., Dalapati, S., Tan, K. T., Li, Z., et al. (2020). Covalent organic frameworks: Design, synthesis, and functions. Chem. Rev. 120 (16), 8814–8933. doi:10.1021/acs.chemrev.9b00550
Glockler, G. (1958). Carbon-oxygen bond energies and bond distances. J. Phys. Chem. 62 (9), 1049–1054. doi:10.1021/j150567a006
Gottle, A. J., and Koper, M. T. M. (2017). Proton-coupled electron transfer in the electrocatalysis of CO2 reduction: Prediction of sequential vs. Concerted pathways using DFT. Chem. Sci. 8 (1), 458–465. doi:10.1039/c6sc02984a
Guan, Y. R., Lai, J. P., and Xu, G. B. (2021). Recent advances on electrocatalysis using pristinely conductive metal-organic frameworks and covalent organic frameworks. ChemElectroChem 8 (15), 2764–2777. doi:10.1002/celc.202100492
Halmann, M. (1978). Photoelectrochemical reduction of aqueous carbon-dioxide on P-type gallium-phosphide in liquid junction solar-cells. Nature 275 (5676), 115–116. doi:10.1038/275115a0
Han, B., Ding, X., Yu, B., Wu, H., Zhou, W., Liu, W., et al. (2021). Two-dimensional covalent organic frameworks with cobalt(II)-Phthalocyanine sites for efficient electrocatalytic carbon dioxide reduction. J. Am. Chem. Soc. 143 (18), 7104–7113. doi:10.1021/jacs.1c02145
Huang, N., Lee, K. H., Yue, Y., Xu, X., Irle, S., Jiang, Q., et al. (2020). A stable and conductive metallophthalocyanine framework for electrocatalytic carbon dioxide reduction in water. Angew. Chem. Int. Ed. 59 (38), 16587–16593. doi:10.1002/anie.202005274
Huang, N., Wang, P., and Jiang, D. L. (2016). Covalent organic frameworks: A materials platform for structural and functional designs. Nat. Rev. Mat. 1 (10), 16068. doi:10.1038/natrevmats.2016.68
Kattel, S., Ramírez, P. J., Chen, J. G., Rodriguez, J. A., and Liu, P. (2017). CATALYSIS active sites for CO2 hydrogenation to methanol on Cu/ZnO catalysts. Science 355 (6331), 1296–1299. doi:10.1126/science.aal3573
Kondratenko, E. V., Mul, G., Baltrusaitis, J., Larrazabal, G. O., and Perez-Ramirez, J. (2013). Status and perspectives of CO2 conversion into fuels and chemicals by catalytic, photocatalytic and electrocatalytic processes. Energy Environ. Sci. 6 (11), 3112. doi:10.1039/c3ee41272e
Kuhl, K. P., Cave, E. R., Abram, D. N., and Jaramillo, T. F. (2012). New insights into the electrochemical reduction of carbon dioxide on metallic copper surfaces. Energy Environ. Sci. 5 (5), 7050. doi:10.1039/c2ee21234j
Kumar, B., Brian, J. P., Atla, V., Kumari, S., Bertram, K. A., White, R. T., et al. (2016). New trends in the development of heterogeneous catalysts for electrochemical CO2 reduction. Catal. Today 270, 19–30. doi:10.1016/j.cattod.2016.02.006
Lee, M. K., Shokouhimehr, M., Kim, S. Y., and Jang, H. W. (2022). Two-dimensional metal-organic frameworks and covalent–organic frameworks for electrocatalysis: Distinct merits by the reduced dimension. Adv. Energy Mat. 12 (4), 2003990. doi:10.1002/aenm.202003990
Li, F. W., MacFarlane, D. R., and Zhang, J. (2018). Recent advances in the nanoengineering of electrocatalysts for CO2 reduction. Nanoscale 10 (14), 6235–6260. doi:10.1039/c7nr09620h
Liang, F., Zhang, K., Zhang, L., Zhang, Y., Lei, Y., Sun, X., et al. (2021). Recent development of electrocatalytic CO2 reduction application to energy conversion. Small 17 (44), 2100323. doi:10.1002/smll.202100323
Lin, C. Y., Zhang, D. T., Zhao, Z. H., and Xia, Z. H. (2018). Covalent organic framework electrocatalysts for clean energy conversion. Adv. Mat. 30 (5), 1703646. doi:10.1002/adma.201703646
Lin, R.-B., and Chen, B. (2018). Reducing CO2 with stable covalent organic frameworks. Joule 2 (6), 1030–1032. doi:10.1016/j.joule.2018.05.017
Lin, S., Diercks, C. S., Zhang, Y. B., Kornienko, N., Nichols, E. M., Zhao, Y., et al. (2015). Covalent organic frameworks comprising cobalt porphyrins for catalytic CO2 reduction in water. Science 349 (6253), 1208–1213. doi:10.1126/science.aac8343
Liu, H., Chu, J., Yin, Z., Cai, X., Zhuang, L., Deng, H., et al. (2018). Covalent organic frameworks linked by amine bonding for concerted electrochemical reduction of CO2. Chem 4 (7), 1696–1709. doi:10.1016/j.chempr.2018.05.003
Liu, M., Pang, Y. J., Zhang, B., De Luna, P., Voznyy, O., Xu, J. X., et al. (2016). Enhanced electrocatalytic CO2 reduction via field-induced reagent concentration. Nature 537 (7620), 382–386. doi:10.1038/nature19060
Liu, M., Wang, Y.-R., Ding, H.-M., Lu, M., Gao, G.-K., Dong, L.-Z., et al. (2021). Self-assembly of anthraquinone covalent organic frameworks as 1D superstructures for highly efficient CO2 electroreduction to CH4. Sci. Bull. (Beijing). 66 (16), 1659–1668. doi:10.1016/j.scib.2021.05.001
Liu, Q., Wu, L. P., Jackstell, R., and Beller, M. (2015). Using carbon dioxide as a building block in organic synthesis. Nat. Commun. 6, 5933. doi:10.1038/ncomms6933
Lohse, M. S., and Bein, T. (2018). Covalent organic frameworks: Structures, synthesis, and applications. Adv. Funct. Mat. 28 (33), 1705553. doi:10.1002/adfm.201705553
Lu, C., Yang, J., Wei, S., Bi, S., Xia, Y., Chen, M., et al. (2019). Atomic Ni anchored covalent triazine framework as high efficient electrocatalyst for carbon dioxide conversion. Adv. Funct. Mat. 29 (10), 1806884. doi:10.1002/adfm.201806884
Lu, M., Zhang, M., Liu, C.-G., Liu, J., Shang, L.-J., Wang, M., et al. (2021). Stable dioxin-linked metallophthalocyanine covalent organic frameworks (COfs) as photo-coupled electrocatalysts for CO2 reduction. Angew. Chem. Int. Ed. 60 (9), 4864–4871. doi:10.1002/anie.202011722
Ma, L., Hu, W., Mei, B., Liu, H., Yuan, B., Zang, J., et al. (2020). Covalent triazine framework confined copper catalysts for selective electrochemical CO2 reduction: Operando diagnosis of active sites. ACS Catal. 10 (8), 4534–4542. doi:10.1021/acscatal.0c00243
Majumdar, A., and Deutch, J. (2018). Research opportunities for CO2 utilization and negative emissions at the gigatonne scale. Joule 2 (5), 805–809. doi:10.1016/j.joule.2018.04.018
Mao, M.-J., Zhang, M.-D., Meng, D.-L., Chen, J.-X., He, C., Huang, Y.-B., et al. (2020). Imidazolium-functionalized cationic covalent triazine frameworks stabilized copper nanoparticles for enhanced CO2 electroreduction. ChemCatChem 12 (13), 3530–3536. doi:10.1002/cctc.202000387
Mikkelsen, M., Jørgensen, M., and Krebs, F. C. (2010). The teraton challenge. A review of fixation and transformation of carbon dioxide. Energy Environ. Sci. 3 (1), 43–81. doi:10.1039/B912904A
Nguyen, P. T. K., Nguyen, H. T. D., Nguyen, H. N., Trickett, C. A., Ton, Q. T., Gutiérrez-Puebla, E., et al. (2018). New metal–organic frameworks for chemical fixation of CO2. ACS Appl. Mat. Interfaces 10 (1), 733–744. doi:10.1021/acsami.7b16163
Pletcher, D. (2018). Organic electrosynthesis – a road to greater application. A mini review. Electrochem. Commun. 88, 1–4. doi:10.1016/j.elecom.2018.01.006
Qiao, J. L., Liu, Y. Y., Hong, F., and Zhang, J. J. (2014). A review of catalysts for the electroreduction of carbon dioxide to produce low-carbon fuels. Chem. Soc. Rev. 43 (2), 631–675. doi:10.1039/c3cs60323g
Ross, M. B., De Luna, P., Li, Y. F., Dinh, C. T., Kim, D., Yang, P., et al. (2019). Designing materials for electrochemical carbon dioxide recycling. Nat. Catal. 2 (8), 648–658. doi:10.1038/s41929-019-0306-7
Schwarz, H. A., and Dodson, R. W. (1989). Reduction potentials of CO2 and the alcohol radicals. J. Phys. Chem. 93 (1), 409–414. doi:10.1021/j100338a079
Segura, J. L., Mancheño, M. J., and Zamora, F. (2016). Covalent organic frameworks based on schiff-base Chemistry: Synthesis, properties and potential applications. Chem. Soc. Rev. 45 (20), 5635–5671. doi:10.1039/C5CS00878F
Sharma, R. K., Yadav, P., Yadav, M., Gupta, R., Rana, P., Srivastava, A., et al. (2020). Recent development of covalent organic frameworks (COFs): Synthesis and catalytic (Organic-Electro-Photo) applications. Mat. Horiz. 7 (2), 411–454. doi:10.1039/C9MH00856J
Song, Y. P., Sun, Q., Aguila, B., and Ma, S. Q. (2019). Opportunities of covalent organic frameworks for advanced applications. Adv. Sci. 6 (2), 1801410. doi:10.1002/advs.201801410
Song, Y., Zhang, J.-J., Zhu, Z., Chen, X., Huang, L., Su, J., et al. (2021). Zwitterionic ultrathin covalent organic polymers for high-performance electrocatalytic carbon dioxide reduction. Appl. Catal. B Environ. 284, 119750. doi:10.1016/j.apcatb.2020.119750
Sun, L., Reddu, V., Fisher, A. C., and Wang, X. (2020). Electrocatalytic reduction of carbon dioxide: Opportunities with heterogeneous molecular catalysts. Energy Environ. Sci. 13 (2), 374–403. doi:10.1039/C9EE03660A
Tackett, B. M., Gomez, E., and Chen, J. G. (2019). Net reduction of CO2 via its thermocatalytic and electrocatalytic transformation reactions in standard and hybrid processes. Nat. Catal. 2 (5), 381–386. doi:10.1038/s41929-019-0266-y
Tu, W., Zhou, Y., and Zou, Z. (2014). Photocatalytic conversion of CO2 into renewable hydrocarbon fuels: State-of-the-Art accomplishment, challenges, and prospects. Adv. Mat. 26 (27), 4607–4626. doi:10.1002/adma.201400087
Uribe-Romo, F. J., Hunt, J. R., Furukawa, H., Klock, C., O'Keeffe, M., Yaghi, O. M., et al. (2009). A crystalline imine-linked 3-D porous covalent organic framework. J. Am. Chem. Soc. 131 (13), 4570–4571. doi:10.1021/ja8096256
Wang, Y., Chen, J., Wang, G., Li, Y., and Wen, Z. (2018). Perfluorinated covalent triazine framework derived hybrids for the highly selective electroconversion of carbon dioxide into methane. Angew. Chem. Int. Ed. 57 (40), 13120–13124. doi:10.1002/anie.201807173
Wang, Y. F., Han, P., Lv, X. M., Zhang, L. J., and Zheng, G. F. (2018). Defect and interface engineering for aqueous electrocatalytic CO2 reduction. Joule 2 (12), 2551–2582. doi:10.1016/j.joule.2018.09.021
Wu, Q., Mao, M.-J., Wu, Q.-J., Liang, J., Huang, Y.-B., Cao, R., et al. (2021). Construction of donor-acceptor heterojunctions in covalent organic framework for enhanced CO2 electroreduction. Small 17 (22), 2004933. doi:10.1002/smll.202004933
Yang, D., Ni, B., and Wang, X. (2020). Heterogeneous catalysts with well-defined active metal sites toward CO2 electrocatalytic reduction. Adv. Energy Mat. 10 (25), 2001142. doi:10.1002/aenm.202001142
Yao, C.-L., Li, J.-C., Gao, W., and Jiang, Q. (2018). An integrated design with new metal-functionalized covalent organic frameworks for the effective electroreduction of CO2. Chem. Eur. J. 24 (43), 11051–11058. doi:10.1002/chem.201800363
Yi, Q., Li, W., Feng, J., and Xie, K. (2015). Carbon cycle in advanced coal chemical engineering. Chem. Soc. Rev. 44 (15), 5409–5445. doi:10.1039/C4CS00453A
Zhang, M.-D., Si, D.-H., Yi, J.-D., Zhao, S.-S., Huang, Y.-B., Cao, R., et al. (2020). Conductive phthalocyanine-based covalent organic framework for highly efficient electroreduction of carbon dioxide. Small 16 (52), 2005254. doi:10.1002/smll.202005254
Zhao, X., Pachfule, P., and Thomas, A. (2021). Covalent organic frameworks (COFs) for electrochemical applications. Chem. Soc. Rev. 50 (12), 6871–6913. doi:10.1039/D0CS01569E
Zheng, T. T., Jiang, K., Ta, N., Hu, Y. F., Zeng, J., Liu, J. Y., et al. (2019). Large-scale and highly selective CO2 electrocatalytic reduction on nickel single-atom catalyst. Joule 3 (1), 265–278. doi:10.1016/j.joule.2018.10.015
Zheng, T. T., Jiang, K., and Wang, H. T. (2018). Recent advances in electrochemical CO2-to-CO conversion on heterogeneous catalysts. Adv. Mat. 30 (48), 1802066. doi:10.1002/adma.201802066
Zhou, Z. Y., and Sun, S. G. (2017). A breakthrough in electrocatalysis of CO2 conversion. Natl. Sci. Rev. 4 (2), 155–156. doi:10.1093/nsr/nww083
Keywords: CO2 reduction, electrocatalysts, carbon neutralization, sustainability, COFs
Citation: Fan Y, Chen M, Xu N, Wang K, Gao Q, Liang J and Liu Y (2022) Recent progress on covalent organic framework materials as CO2 reduction electrocatalysts. Front. Chem. 10:942492. doi: 10.3389/fchem.2022.942492
Received: 12 May 2022; Accepted: 30 June 2022;
Published: 22 July 2022.
Edited by:
Dong Liu, Jiangsu University, ChinaReviewed by:
Xun Cui, China University of Geosciences, ChinaCopyright © 2022 Fan, Chen, Xu, Wang, Gao, Liang and Liu. This is an open-access article distributed under the terms of the Creative Commons Attribution License (CC BY). The use, distribution or reproduction in other forums is permitted, provided the original author(s) and the copyright owner(s) are credited and that the original publication in this journal is cited, in accordance with accepted academic practice. No use, distribution or reproduction is permitted which does not comply with these terms.
*Correspondence: Yubing Liu, eWJsaXVAbmp1LmVkdS5jbg==