- 1Department of Pharmaceutical Engineering and Technology, IIT-BHU, Varanasi, Uttar Pradesh, India
- 2Dr. B.C. Roy College of Pharmacy and Allied Health Sciences, Durgapur, West Bengal, India
- 3Department of Pharmaceutical Chemistry, Narayan Institute of Pharmacy, Gopal Narayan Singh University, Sasaram, Bihar, India
- 4Department of Pharmaceutical Biology, Faculty of Pharmaceutical Sciences, UCSI Education SDN.BHD., Kuala Lumpur, Malaysia
Histone deacetylases (HDACs) are enzymes that play a role in chromatin remodeling and epigenetics. They belong to a specific category of enzymes that eliminate the acetyl part of the histones’ -N-acetyl lysine, causing the histones to be wrapped compactly around DNA. Numerous biological processes rely on HDACs, including cell proliferation and differentiation, angiogenesis, metastasis, gene regulation, and transcription. Epigenetic changes, specifically increased expression and activity of HDACs, are commonly detected in cancer. As a result, HDACi could be used to develop anticancer drugs. Although preclinical outcomes with HDACs as monotherapy have been promising clinical trials have had mixed results and limited success. In both preclinical and clinical trials, however, combination therapy with different anticancer medicines has proved to have synergistic effects. Furthermore, these combinations improved efficacy, decreased tumor resistance to therapy, and decreased toxicity. In the present review, the detailed modes of action, classification of HDACs, and their correlation with different cancers like prostate, breast, and ovarian cancer were discussed. Further, the different cell signaling pathways and the structure-activity relationship and pharmaco-toxicological properties of the HDACi, and their synergistic effects with other anticancer drugs observed in recent preclinical and clinical studies used in combination therapy were discussed for prostate, breast, and ovarian cancer treatment.
Introduction
Cancer is a significant public health problem worldwide and the second leading cause of death in the United States. An estimated 609,360 people in the United States will die from cancer in 2022, corresponding to almost 1700 deaths per day. The greatest number of deaths are from the cancers of lung, prostate, and colorectum in men and of the lung, breast, and colorectum in women (Siegel et al., 2022). According to the International Agency Global Cancer Burden (Ferlay et al., 2020), the number of new cases of cancer is 19.3 million whereas the death of cancer patients is 10 million in 2020. The most commonly diagnosed cancer, with an estimated 2.3 million new cases of female breast cancer (11.7%) has surpassed lung cancer (11.4%), colon and rectal (10%), prostate (7.3%), and stomach (5.6%) cancer. Lung cancer continues to exist as the most fatal type of cancer-causing millions of deaths (18%). HDACs are enzymes that play a role in chromatin remodeling and epigenetics. In cancers, HDACs are very often upregulated and can silence apoptosis-induced tumor suppressor genes to enhance the cancer progression (West and Johnstone, 2014). The active focus of several groups in the 1970s was the search for HDACs and proteins which have the potential of removing acetyl groups from histones. 18 types of mammalian HDACs were discovered so far and categorized into 4 major classes/groups. The first category or the class I of HDACs includes HDAC 1, 2, 3, and 8 while 4, 5, 7, and 9 are classified as class IIa type of HDACs. HDACs 6 and 10 are classified as class IIb while sirtuins (SIRT1-7) make up class III. HDAC 11 is included in the class IV type of HDAC (Seto and Yoshida, 2014). HDAC expressions that are abnormal are implicated in several phases of cancer and have become one of the symbols of hematological malignancy and tumors (Darwiche, 2020; Pant et al., 2020). HDACs are important players in cancer because they regulate a variety of cellular and molecular activities. HDAC inhibitors (HDACi) cause cell death by arresting the G1 phase of the cell cycle by reducing the cyclins and cyclin-dependent kinases (CDK) (Kim et al., 2000; Chun, 2015). Inhibition of HDACs also affects cancer cell apoptosis by controlling the expression of pro-and anti-apoptotic proteins (Zhang and Zhong, 2014; Gong et al., 2019). The discovery of particular HDACi has provided a valuable way to study HDACs biology. Hence, these inhibitors may act as a budding healing option for various chronic diseases like cancer, immunological and heart diseases (Losson et al., 2016).
The histone protein core is being wrapped throughout with the DNA in primitive eukaryotic cells, packaging, protecting, and regulating it. This structure, known as chromatin, is compressed and “closed,” which is linked to suppress the transcript process. On the other hand, it may be “open,” allowing transcription-control proteins to wrap DNA. Post-translational modifications (PTM) like phosphorylation, acetylation, methylation, and ubiquitination may play important roles in regulating chromatin in its many active states (Cui et al., 2014; Wang Y. C. et al., 2014). The well-understood mechanism is the reversible phosphorylation, acetylation, or methylation within histone tails to regulate chromatin. In many cells, the regulation of chromatin structure by histone PTMs has emerged as a significant driver of transcriptional responses. So, histone controls the protein machinery by the addition or deletion of the PTMs, which have proven pivotal in the understanding of physiological responses in a variety of cell types (Audia and Campbell, 2016).
Genetically, cancer is linked to the alteration in the genome and modifications, such as DNA methylation and histone alterations that can affect chromatin architecture. The nucleosome (the active part of chromatin) is wrapped around a histone core, which is made up of two core histones (H2A, H2B, H3, and H4) (Rhodes, 1997; Shaytan et al., 2015). Histone acetylation, phosphorylation, and methylation are the most prevalent epigenetic processes deregulated in cancer, where histone acetylation is having the most prominent role in cancer (Cheng et al., 2019; Rajan et al., 2020). Although several HDACi have been licensed for cancer treatment, clinical applications have been limited due to the HDACi’s poor pharmacokinetics, bioavailability, and selectivity, and most of them require the use of other medications to produce better outcomes. In the present review, efforts were made to evaluate the role of HDACi in the management of three common cancers: prostate, breast, and ovarian. Furthermore, a detailed discussion on the HDACi as the current medicines and clinical trials being conducted for the treatment of these cancers.
HDACs: Classification, enzymatic activities, and biological function
Eighteen human HDACs have been found and classified into four groups based on their sequence similarity to yeast HDACs. HDACs 1, 2, 3, and 8 are all classified as class I HDACs. They are the most common type of HDACs found in the nucleus, and they are related to the yeast Rpd3 in several ways (Emiliani et al., 1998; Buggy et al., 2000; Hu et al., 2000). Class II HDACs are homologous to yeast Hda1 and greater in size than the other two classes. They are classified into two subgroups based on domain organization and sequence: class IIa and class IIb. The class IIa HDACs are inactive on acetylated substrates, thus differing from class I and IIb enzymes (Lahm et al., 2007). HDACs 6 and 10 belong to Class IIb, and they have an extra deacetylase domain (Miska et al., 1999; Kao et al., 2000; Zhou et al., 2001; Fischer et al., 2002; Guardiola and Yao, 2002; Kao et al., 2002). Class III, also known as sirtuins (SIRTs), is a group of proteins that includes SIRT1-7 and has a wide range of activity and location. SIRT1, 6, and 7 are mostly found in the nucleus, SIRT2 is mostly located in the cytoplasm, SIRT3, 4 and 5 mostly located in mitochondria (Lin et al., 2000; Carafa et al., 2012; Chen B. et al., 2015). Sirtuins are NAD+ -dependent class III protein deacetylases that can be found in anything from bacteria to humans (Kida and Goligorsky, 2016). Sirtuins can sense changes in cellular energy by utilizing NAD+ as a cofactor for their enzymatic activity. Cell survival during stress, metabolic balance, chromatin control, and cell differentiation are the activities that Sirtuins can accomplish (Pant et al., 2017a; Wang Y. et al., 2019). Only HDAC 11 are found in Class IV (Gao et al., 2002). SIRTs are NAD+ dependent enzymes, whereas the other three types are Zinc cation (or Zn2+ ion)-dependent HDACs (Imai and Guarente, 2014). The classification, locations, and diverse enzymatic activities of HDACs are shown in Table 1. The IC50 values of each HDACIs have been shown in Table 2.
Histone deacetylation and cancer initiation
Through their effects on chromatin compaction and the stability of other cellular target proteins, HDACs play a critical role in the epigenetic regulation of gene transcription and expression (Ropero and Esteller, 2007). Dysregulation of DNA methylation and post-translational histone modifications, particularly histone acetylation, are common features of human cancer, with the disastrous result of gene transcription deregulation. Loss of acetylated Lys16 (K16-H4) and trimethylated Lys20 (K20-H4) of histone H4 is linked to hypomethylation of repetitive sequences and is a common event in human cancer (Fraga et al., 2005). Another research on gastrointestinal malignancies found that the decreased histone acetylation is linked to tumor invasion and metastasis as well as tumorigenesis (Yasui et al., 2003). HDACs overexpression has been shown in a variety of solid and hematological malignancies (Shankar and Srivastava, 2008), influencing a variety of cellular functions including proliferation, cell death, metastasis, autophagy, metabolism, and ciliary expression.
HDACs have been shown to regulate apoptosis in cancer cells by changing the expression of apoptotic proteins. HDAC2 expression was found to be abnormal in cancer cells, and HDAC2 inhibitors suppressed cell motility, invasion, and proliferation, as well as causing cell death, in gastric cancer cells (Kim et al., 2013). In human lung cancer cells, inhibiting HDAC2 inhibition leads to the activation of p53 and Bax that inhibits tumor cell proliferation causing cell death (Jung et al., 2012). HDACs are involved in the deacetylation of non-histone proteins as well as histone deacetylation, hence their role in cancer is multifaceted. In vivo and in vitro, HDAC1 interacts with the tumor suppressor p53 and deacetylates it (Juan et al., 2000; Luo et al., 2000). Under stressful situations, p53 is phosphorylated and acetylated. Since acetylated lysine residues in p53 overlap with ubiquitinated lysine residues, p53 acetylation promotes protein stability and activation, prompting cell-division checkpoints, persistent cell-division arrest, and cell death.
HDAC2 was also discovered to favorably control Aurora A kinase, which promotes pancreatic cancer cell proliferation while inhibiting cell death by inducing ciliary loss (Kobayashi et al., 2017). p53 is a tumor suppressor gene that can cause altered cells to die. The effect of p53 acetylation in tumor suppression has been extensively studied (Dai and Gu, 2010). MDM2 ubiquitinates p53 and leads to its destruction when HDACs such as SIRT1 and SIRT2 remove acetyl groups from p53’s C-terminal lysines, reducing p53 levels in cells (Lee and Gu, 2013). Lower p53 levels in cells may promote cell proliferation while inhibiting apoptosis.
SIRT1 controls histone acetylation (mostly at the K16-H4 and K9-H3 locations) and the acetylation of transcription factors such as p53 (Vaziri et al., 2001), p300 histone acetyltransferase (Bouras et al., 2005), E2F1 (Wang et al., 2006), DNA repair ku70 (Cohen et al., 2004; Fu et al., 2006). When all of these factors are considered, it is obvious that sirtuin dysregulation plays a role in cancer development. SIRT1 is upregulated in lung cancer (Yeung et al., 2004), prostate cancer (Kuzmichev et al., 2005), and leukemia (Bradbury et al., 2005), but downregulated in colon cancer (Özdağ et al., 2006).
When it comes to the role of HDACs in cancer, there are several mechanisms through which HDACs contribute to cancer development. The majority of investigations to date have focused on the impact of abnormal HDACs recruitment to certain promoters via interactions with fusion proteins resulting from chromosomal translocations common in hematological malignancies. Acute promyelocytic leukemia (APL) is an archetypal case that serves as a paradigm for various other hematological cancers. The chromosomal translocation that results in fusion proteins containing RAR-PML and RARPLZF is a hereditary feature of this disease. These fusion proteins bind to retinoic acid-responsive elements (RAREs) and recruit the HDACs repressor complex with high affinity, blocking retinoic acid-binding and suppressing the expression of genes that control myeloid cell differentiation and proliferation (Lin et al., 2001).
HDACs are known to deacetylate a wide range of proteins, including those involved in cell cycle regulation. The S phase and M phase transitions are critical for genomic integrity (Telles and Seto, 2012). The E2F members interact with retinoblastoma protein (pRb) to cause cell cycle advancement and apoptosis. By attracting HDAC 1 to E2F-responsive promoters, pRb can inhibit the E2F-mediated transcription of cell cycle proteins (Brehm et al., 1998).
CDH1, or epithelial-cadherin, is a cell marker that is decreased during metastasis. HDAC1 is recruited to the CDH1 promoter in pancreatic cancer cells, resulting in deacetylation of histone 3 and 4 proteins in the nucleus and E-cadherin depletion, which aids in the epithelial-mesenchymal transition (EMT) (Von Burstin et al., 2009). To suppress E-cadherin expression, the transcription factor Snail binds HDAC1, HDAC2, and the corepressor complex mSin3A to the promoter (Peinado et al., 2004). Downregulation or loss of function of E-cadherin has been linked to carcinomas gaining invasive capacity (Christofori and Semb, 1999; Hajra and Fearon, 2002), suggesting that abnormal recruitment of HDACs to this promoter could play a role in tumor invasion and metastasis.
HDACs activity may be controlled directly by several metabolites produced by various intracellular metabolic processes. The addition of NADPH and Coenzyme A to recombinant HDAC1 and HDAC2 complexes boosted their cellular activity (Vogelauer et al., 2012). A bioactive lipid sphingosine-1 phosphate, generated during nuclear sphingolipid metabolism involved in the oxidation of fatty acids, was discovered to suppress HDAC activity by binding to its active site, leading to more research into the function of metabolic control in HDAC activity (Hait et al., 2009; Huang et al., 2012). HDAC3 has oncogenic effects in cholangiocarcinoma (CCA) cells, according to Yin et al. (2017), by suppressing apoptosis and promoting cell growth. Furthermore, elevated HDAC3 and HDAC6 expressions were found in CCA patients’ tissues, which were associated with a poor prognosis (Hubbert et al., 2002; Gradilone et al., 2013; Yin et al., 2017).
The role of autophagy in the genesis, maintenance, and advancement of cancer cells has been intensively researched. HDACs like HDAC6 (Gradilone et al., 2013) and HDAC10 deacetylate cytoplasmic proteins in CCA cells, and they’ve been linked to the autophagy process through modulating critical autophagy proteins including LC3-II and Beclin1 (Koeneke et al., 2015). The increased autophagic flow was seen in cells lacking class I HDACs, as evidenced by increased autophagosomal proteins such as LC3-II, Beclin1, and ATG5 (Schipper et al., 2014). Higher expression of autophagy regulators involved in various cell functions is linked to the depletion of HDACs such as class I and IIa isozymes as autophagy can promote cancer cell survival, simultaneously targeting autophagy might improve the therapeutic effects of HDACi against cancer.
HDACi in cancer therapy
HDACi have shown potent therapeutic effects in different types of cancers, in both preclinical research and clinical trials. HDACi can considerably reduce cancer burden by attenuating tumor growth and regulating aberrantly proliferating vasculature (Guerriero et al., 2017). HDACi can suppress HDAC activity, promote histone acetylation aggregation in autosomes, and induce gene expression (Sanaei and Kavoosi, 2019). HDAC is have been found to have anticancer effects in cancer cells by a variety of pathways, including cell cycle arrest, apoptosis, and autophagy induction (Lam et al., 2013; Luchenko et al., 2014). Sodium butyrate was discovered in the 1970s to be able to transform red leukemia cells into normal cells and resynthesize haemoglobin. The first HDACi was discovered as a result of this process, which was accompanied by severe histone hyperacetylation (Ginsburg et al., 1973; Riggs et al., 1977).
Following FDA approval, HDACi are currently divided into four categories: (i) hydroxamic acids or hydroxamates, such as e.g., trichostatin A (TSA) and vorinostat, panobinostat and belinostat; (ii) cyclic peptides, including depsipeptides, tetrapeptides [e.g., romidepsin (FK228)]; (iii) benzamides, such as chidamide, entinostat (MS-275); and (iv) short-chain fatty acids, including valproic acid (VPA) and phenylbutyrate (Li and Zhu, 2014; Li and Seto, 2016). Tsuji et al. (1976) isolated the first natural HDACi, TSA, which was derived from Streptomyces hygroscopicus. Following the discovery of TSA, trapoxin was isolated from fungi and also found to act as an HDACi (Itazaki et al., 1990).
HDACi can also increase cancer cell death by inducing DNA damage, cell cycle arrest, apoptosis, and autophagy, as discussed previously. Some new SIRT inhibitors, such as MC2494, MHY2245, MHY2256, tenovin-6, and YC8-02, mediate apoptosis or autophagy, and so have anti-tumor properties (De et al., 2018; Tae et al., 2018; Li et al., 2019; Igase et al., 2020). The hydroxamic acid class includes vorinostat, the first FDA approved HDACi to treat patients with cutaneous T-cell lymphoma (CTCL). In 2009, the FDA approved the cyclic peptide romidepsin for the treatment of CTCL. In 2014, the FDA approved panobinostat and belinostat for the treatment of peripheral T-cell lymphoma (PTCL), with belinostat receiving additional clearance from the European Medicines Agency (EMA) (Andreu Vieyra and Berenson, 2014). The majority of SIRT inhibitors are still in the preclinical stage. Only nicotinamide (vitamin B3) has been used in clinical trials to treat cancer thus far (e.g., NCT02416739 and NCT00033436). Nicotinamide has been found to have a function in the prevention of nonmelanoma skin cancers, which are caused mostly by UV exposure (Chen et al., 2015).
Natural substances offer a wide spectrum of medications that are both powerful and pleiotropic. Various HDACi have so far been discovered to be of natural origin. HDACi like FK322, a cyclic peptide derived from Chromobacterium violaceum, and TSA from S. hygroscopicus inhibit HDAC 1 and 2 activities selectively. HDACi derived from a fungus, trapoxin A and depudecin, are also naturally occurring HDACi. Natural HDACi, such as largazole and azumamides, are also found in some marine organisms (Bassett and Barnett, 2014; Singh et al., 2018). Butyrate is a short-chain fatty acid produced by the gut microbiota during the fermentation of dietary fibers, and it has been found to inhibit HDACs class III, which affects post-translational modifications of histones (Delage and Dashwood, 2008; Morrison and Preston, 2016). Butyrate has been shown to reduce the growth of hepatocellular carcinoma (Wang et al., 2013; Pant et al., 2017b), lung cancer (Amoêdo et al., 2011), breast cancer (Chopin et al., 2004), pancreatic cancer (Farrow et al., 2003; Natoni et al., 2005), and colon cancer cells by increasing histone acetylation (Donohoe et al., 2012, 2014).
HDACi are currently useful for treating a variety of cancers, although adverse effects such as diarrhea, myelosuppression, and cardiovascular toxicity limit their use. Because HDACs affect various cellular pathways and current HDACi are mainly non-isoform-selective, several adverse effects are possible (pan-HDACi). As a result, more potent and isoform-selective HDACi are still needed like SB-429201, PCI-34051, SB-379278A, tubacin (Bieliauskas and Pflum, 2008).
Prostate cancer
Prostate cancer is the most commonly diagnosed cancer in men and the second greatest cause of death from cancer. Prostate cancer is the most frequent cancer among males in the United States, with over 34,000 men died from it in 2021. (Siegel et al., 2022). It is also worth noting that it is the second-leading cause of cancer death among men (Kaushik et al., 2015). Even while localized prostate cancer has a 100%, 5-year survival rate, it reduces to 29.3 percent when cancer spreads to other organs (Damodaran et al., 2017). Furthermore, between 2005 and 2018, the number of new cases of prostate cancer increased by 31%, from 9,74,000 to 1.3 million (Fitzmaurice et al., 2017; Rawla, 2019). After the initial diagnosis, most patients are treated with localized radical prostatectomy, radiation therapy, proton beam therapy, and cryosurgery (Hayden et al., 2010; Ukimura, 2010). Patients with metastatic illness or recurring cancer with the localized region and distant metastases, should consider androgen deprivation therapy (ADT) or castration therapy as the first line of treatment (Perlmutter and Lepor, 2007). Unfortunately, despite an excellent initial therapeutic response, most patients treated with ADT eventually develop a very aggressive and therapy-resistant type of prostate cancer, resulting in poor clinical outcomes (Miller et al., 2017; Moreira et al., 2017).
The human prostate is a walnut-sized glandular structure that emerges from the urogenital sinus during embryonic development (Lee et al., 2011). Its main job is to make seminal fluid, which contains zinc, citric acid, and a variety of enzymes, including prostate-specific antigen protease (PSA). Because of the lack of well-characterized prostate epithelial lineage, the cellular origin of prostate cancer is unclear (Stoyanova et al., 2013; Wang et al., 2014). Because prostate cancer cells rely on androgens for survival in the early stages, androgen removal is the most common systemic treatment, which is hypothesized to act by inducing apoptosis (Isaacs, 1994; Montironi et al., 1998). Androgen deprivation therapy has been the standard of care for advanced prostate cancer, resulting in remission in 80%–90% of men with advanced disease and a median disease-free survival of 12–33 months. Unfortunately, despite a past response to androgen deprivation, neoplastic cells will continue to proliferate in the majority of individuals. Castration-resistant prostate cancer (CRPC) is a progressive form of prostate cancer with a median overall survival of 23–37 months after the onset of androgen deprivation (Hellerstedt and Pienta, 2002). Patients with CRPC who are unresponsive to androgen deprivation and/or chemotherapy require newer therapeutic agents. By improving the binding between histones and the DNA backbone, the HDACs family of enzymes limits the expression of genomic regions.
Development HDACi against prostate carcinoma
Jadhavar et al. (2016) designed some androgen receptor (AR)-HDAC6 inhibitors based on the structures of AR inhibitor enzalutamide and HDACi vorinostat and panobinostat. After synthesizing fourteen hybrid compounds, a few compounds were selected based on inhibitory potential against a range of HDACs (HDAC-1, 2, 3, and 6) and AR as well as on selectivity towards HDAC6 as compared to other HDACs. For example, one compound, the structure of which is presented in Figure 1 as (I), has an IC50 value of 36 nM against HDAC6 whereas its inhibitory potency against other HDACs (e.g., HDAC1, HDAC2, and HDAC3) is found in the micromolar range. HDAC6 has been reported to be involved in the acetylation of heat shock protein 90 (Hsp90) to regulate the nuclear localization and activation of the AR. Therefore, dual HDAC6/AR inhibitors are likely to produce synergistic effects that may conveniently be utilized as a new approach for the treatment of prostate cancer. The cytotoxicity of some dual HDAC6/AR inhibitors was therefore tested by these investigators with breast carcinoma cell line MDA-kb2 to establish that the designed compounds have similar cytotoxic potential as enzalutamide. When tested on prostate cancer cell line LNCaP, hyperacetylation of tubulin was obtained from two compounds.
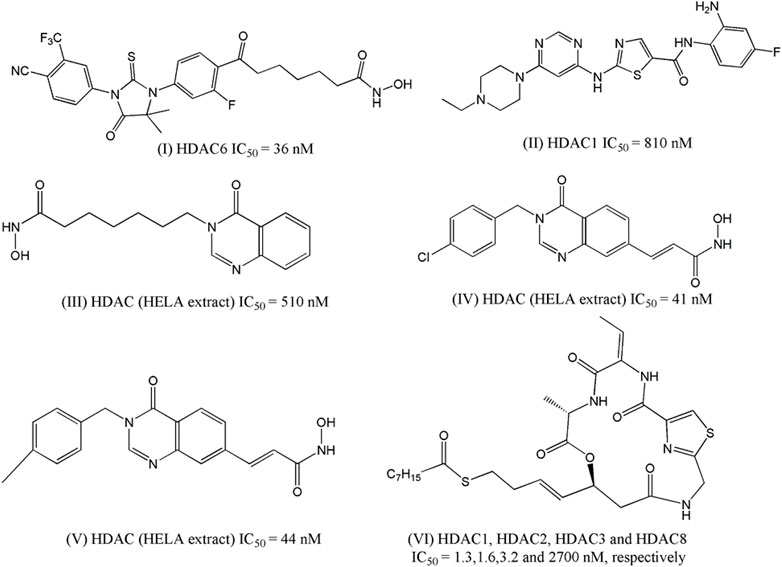
FIGURE 1. Structures of HDACi designed in recent years depicted anti-proliferative potential against the prostate carcinoma cell lines.
Chen and co-workers (Chen et al., 2016) attempted to develop compounds with dual Bcr-Abl and HDACi by combining the fragment of dasatinib (one well-known clinically used Bcr-Abl inhibitor) with the zinc-binding domain of HDACi named MS-275. The designed structure lacked hydroxylamine moiety and instead consisted of o-Phenylenediamine residue that served as a zinc-binding domain in the HDACs. The synthesized compounds were first tested against Bcr-Abl and HDAC-1. The most potent compounds were then subjected to enzymatic assay against multiple HDACs enzymes and their antiproliferative potential was measured against three cell lines including prostate cancer cell line DU145. The most potent compound (II, Figure 1) was found to have an IC50 of 0.60 µM against this cell line and at the same time, it also showed micromolar potencies against the HDAC-1 enzyme (IC50: 0.81 µM).
Hieu et al. (2018) developed quinazolin-4(3H)- one based HDACi on the basis of the fact that this moiety is frequently found in various therapeutic agents. In their designed compounds, this moiety was assumed as a cap group whereas either N-hydroxybenzamides or N-hydroxypropenamides was used as zinc binding group. Sixteen synthesized compounds were tested against HDACs as well as against three different cancer cell lines including prostate cancer cell line PC-3. The most active HDACi was reported with IC50 value of 90 nM whereas the same compound depicted an IC50 of 810 nM against PC-3 cell. Considering that both these activities are higher than standard HDACi vorinostat, it may be inferred that the design was indeed successful, as far as the efficacy is concerned. By modifying the linker moiety of these structures, the same group reported another investigation (Huan et al. (2019), and this time too, the biological activities of some synthesized compounds were found to be highly satisfactory as these both HDACi as well as cytotoxic agent. For example, one compound, structure of which is presented in Figure 1 as III, depicted IC50 of 520 nM against HDACs (HELA extract) whereas the IC50 value of 210 nM was obtained in the cytotoxicity assay against PC-3 cell line. Noticeably, the cytotoxic potency of this compound was 15-times higher than that of vorinostat. More recently, another library of compounds was designed and synthesized by this group keeping the quinazolin-4(3H)-one moiety intact but generating various derivatives by changing substituents at the N-3 position of this aromatic moiety as well as by varying the zinc binder groups (Anh et al., 2021). Similar to earlier investigations, these compounds were tested against multiple cancer cell lines and one of these was PC-3. Two compounds (IV and V of Figure 1) were found to be the most potent derivatives. Their HDAC inhibitory activity (IC50 values, 0.041–0.044 μM) and cytotoxicity (IC50 values, 0.671–1.211 μM) were even higher than those of vorinostat. Furthermore, some compounds showed up to 10-fold more potent HDAC6 inhibition compared to their inhibitory activity in total HDACs extract assay. Further analyses of IV and V revealed that these compounds strongly induced both early and late apoptosis and arrested SW620 cells at the G2/M phase.
Zhang et al. (2020) took a different approach to successfully design HDACi by modifying the structure of romidepsin, which is a clinically approved HDACi used for the treatment of lymphomas. The investigators initially identified a novel cyclic depsipeptide through structural modification of romidepsin and this novel compound was found to selectively block class I HDACs. A series of novel cyclic depsipeptides was then prepared and screened against HDACs to select the most potent derivatives. On one hand, these compounds selectively inhibited class I HDACs and at the same time displayed nanomolar antiproliferative potencies against a range of cancer cell lines, and one compound (VI, Figure 1) was reported with GI50 of 7 nM against prostate cancer cell PC-3 and more importantly it showed 200 times more selectivity against human normal cell line.
HDACs and prostate cancer
The molecular processes governing prostate cancer cell proliferation in an androgen-deficient environment are now being investigated. Covalent acetylation and deacetylation of histone proteins are one of these methods. The transcription of proto-oncogenes and tumor suppressor genes is regulated by these covalent changes. In prostate cells, HDACs regulate the expression of various functional genes, including the androgen receptor (AR). As a result, HDACi are currently being studied in CRPC patients. It has recently been discovered that the majority of recurrent prostate tumors are dependent on the AR signaling axis rather than being hormone-refractory or androgen-independent (Montgomery et al., 2008). The AR is a cytoplasmic protein that binds to testosterone or dihydrotestosterone in the nucleus, causing gene transcription to change. The synthesis of intracrine androgens may be important in sustaining tumoral androgen levels and the progression of CRPC (Stanbrough et al., 2006). HDACs are widely produced and elevated in prostate cancer, according to several lines of evidence (Waltregny et al., 2004; Weichert et al., 2008). Using a patient cohort of 192 patients who underwent radical prostatectomy, Weichert and colleagues investigated the expression patterns of HDACs 1, 2, and 3 in prostate cancer. HDAC 1 and 2 were found to have a positive correlation with Gleason scores, with high-grade tumors expressing both isoforms at higher rates. Furthermore, the Ki-67-positive proliferative fraction of prostate cancer cells is linked substantially with HDACs 1, 2, and 3, indicating increased cellular proliferation (Weichert et al., 2008).
During the malignant transformation of prostate epithelial cells, hypermethylation of CpG islands and chromatin remodeling play essential roles in the suppression of several tumor suppressor genes. It has been shown that DNMT1 and HDAC1 levels are higher in prostate cancer than in BPH, implying that they play a role in DNA-methylation-induced chromatin remodeling-induced inactivation of different essential genes (Patra et al., 2001). Dihydrotestosterone causes acetylation of the androgen receptor (AR), and HDACi increase p300 binding while reducing N-CoR/HDAC/Smad3 co-repressor binding, improving cell survival and growth in prostate cancer cells both in vivo and in vitro (Fu et al., 2003). NAD-dependent SIRT1 is necessary for androgen antagonist-mediated transcriptional repression and growth suppression, according to new research. SIRT1 and nuclear receptor co-repressor are recruited to AR-responsive promoters by androgen antagonist-bound androgen receptor, which deacetylates histone H3 locally at the PSA promoter (Dai et al., 2007). ARR19 is a new AR co-repressor that engages HDAC4, resulting in AR transactivation suppression (Jeong et al., 2004). Androgen has also been shown to play a role in the nuclear localization of HDAC4 and to be more prevalent in the nucleus in more aggressive prostate tumors (Halkidou et al., 2004). These data show that androgen, HDAC4, and ARR19 all play key roles in the progression of prostate cancer. Maspin, a tumor suppressor, has been linked to malignancies that are better differentiated, more responsive to pharmacological therapy, and have a better prognosis. In human prostate cancer cell lines and tissues, Maspin interacts with HDAC1 (Li et al., 2006). HDAC1 is inhibited by this direct molecular interaction in both the nucleus and the cytoplasm via glutathione S-transferase (GST), according to studies (Li et al., 2006). Through DNA methylation and chromatin changes, the hDAB2IP gene is epigenetically repressed in prostate cancer (Chen et al., 2003). Ezh2, a histone lysine methyltransferase, and HDAC I are involved in the downregulation of hDAB2IP (Chen et al., 2005). HDACIs with anti-prostate cancer activities have been shown in Table 3.
Preclinical studies of HDACi in prostate cancer
Inhibition of Class I HDAC1, −2, and −3 by the adamantyl-capped HDACI CN133 was superior to vorinostat (IC50 = 0.6, 2, and 0.3 nM for CN133; 4, 11, and 3 nM for vorinostat), whereas vorinostat was more potent against HDAC6 (IC50 = 2 nM) than CN133 (IC50 = 4.1 nM). In 22Rv1 CRPC cells, CN133 was 100 times more antiproliferative (IC50 = 10 nM) than vorinostat (IC50 = 1 M). CN133 reduced AR signaling and decreased CRPC cell migration and invasion. Mice with 22Rv1 CRPC were given CN133 (1 mg/kg), which reduced tumor volume and weight by 50% when compared to placebo-treated mice (Chen et al., 2021).
Compound 2–75 is an enzalutamide hybrid with HDAC inhibitory activity that promoted p21, increased acetyl-tubulin levels (due to enhanced HDAC6 inhibition), and lowered Hsp90 and AR protein levels in C4-2 prostate cancer cells (Rosati et al., 2016). Deeper studies of 2–75 in CRPC were conducted based on these findings. DHT-induced AR transcriptional activity and AR translocation to the nucleus were reduced by compound 2–75 more effectively than enzalutamide. In addition to AR, 2–75 downregulated the mutant AR-V7 in prostate cancer cells in a proteasome-dependent manner, implying that 2–75-treated cells had better AR breakdown. In vivo tests with LNCaP tumor models demonstrated that 2–75 treatment (10 mg/kg, intratumoral injection twice weekly) exhibited tumor growth inhibitory effect comparable to enzalutamide, but that 2–75 had better tumor growth suppression in the long run (after Day 24) when compared to enzalutamide. In the tumor bodies of treated animals, 2–75 activity was associated with enhanced apoptotic induction and decreased AR nuclear accumulation (Hu et al., 2019).
Compound CUDC-101 suppressed HDACs, EGFR, and HER2 by combining an HDAC inhibitory fragment with an EGFR inhibitory scaffold derived from the authorized anticancer active EGFR inhibitor erlotinib (Lai et al., 2010). CUDC-101 inhibited full-length AR as well as the mutant AR-V7 form in CRPC cells, increased p21, and decreased HER2/NEU. CUDC-101 (50 g/kg/day for 14 days) effectively suppressed tumor growth in castrated mice with aggressive 22Rv1 CRPC tumors, with no discernible weight loss in the treated mice (Sun et al., 2016). Erlotinib and CUDC-101 both have limitations that necessitate more research. Cytochrome P450 enzymes can activate the ethinylphenyl residue of erlotinib, resulting in oxidized phenol and quinone molecules with toxicity potential (Li et al., 2010). Indeed, in cancer patients receiving corticosteroids or ciprofloxacin the erlotinib has been shown to increase the incidence of deadly gastrointestinal tract perforations (Gass-Jégu et al., 2016).
In DU145 CRPC cells, the chimeric compounds 3ClQuin-vorinostat and 3BrQuin-vorinostat showed 3–4 times stronger growth inhibitory action (IC50 = 3.23 M for 3ClQuin-vorinostat and 3.53 M for 3BrQuin-vorinostat) than gefitinib (IC50 = 11.9 M); however, vorinostat (IC50 = 0.68 M) was still more antiproliferative. Nonetheless, 3ClQuin-vorinostat and 3BrQuin-vorinostat combined EGFR inhibitory efficacy with HDAC inhibition, decreased EGFR expression in DU145 cells to a level comparable to vorinostat, showed only minor unspecific toxicity, triggered death, and prevented angiogenesis (Goehringer et al., 2021). As a result of the reduced erlotinib (and vorinostat) toxicity and resistance generation, these chimeric compounds may be suitable anticancer therapeutic candidates in prostate cancer therapy.
Another promising HDAC/kinase inhibitor, CUDC-907 (fimepinostat), was developed to target HDAC enzymes as well as the kinase PI3K (Qian et al., 2012). CUDC-907 decreased HDACs and PI3K signaling in a panel of eight prostate cancer cell lines, promoted apoptosis in a dose-dependent manner linked with enhanced pro-apoptotic Bim, and lowered antiapoptotic Mcl-1 and Bcl-xL expression in 22Rv1 CRPC cells, and inhibited HDACs and PI3K signaling. Furthermore, CUDC-907 treatment increased DNA damage because DNA damage response proteins were downregulated (Wee1, CHK1, RRM1, and RRM2). Finally, in castration-resistant LuCaP 35CR mice xenografts, CUDC-907 (100 mg/kg/day, p.o.) suppressed in vivo tumor growth by around 60% while causing little weight loss (Hu et al., 2020).
Registered HDACi in clinical trials of prostate cancer
Several clinical trials with HDACi have been conducted to assess the benefits and drawbacks of their use in clinics. Only the most important findings from clinical trials with HDACi for the treatment of castration-resistant prostate cancer conditions are reported in Table 4.
Entinostat (SNDX-275, MS-275) is a class I and IV HDACi that is taken orally. In vitro and in vivo, entinostat decreases prostate cancer (PCa) growth and suppresses Treg cell function. In the small phase I investigation, Entinostat at the indicated dose levels in conjunction with the standard dose of enzalutamide demonstrated a promising safety profile (NCT03829930). Prostate cancer cells can develop in response to androgen. Enzalutamide, for example, may reduce the quantity of androgen produced by the body. Decitabine may inhibit tumor cell growth by inhibiting some of the enzymes required for cell proliferation. Decitabine with enzalutamide may be more effective in treating castration-resistant prostate cancer patients (NCT04471974).
Vorinostat inhibits class I and II HDACs. It has been demonstrated to reduce PC-3 xenograft tumors and decrease the growth of PC-3, DU-145, and LNCaP human prostate cancer cell lines. It causes Akt dephosphorylation by disrupting HDAC complexes bound to PP1, which results in more PP1–Akt association complexes and enhanced PP1-Akt association (Kulp et al., 2006). A phase II clinical trial (NCT00330161) was just completed on the medication (Bradley et al., 2009). Oral vorinostat is being tested in two other clinical trials at the same time. The Roswell Park Cancer Institute is conducting a phase I trial (NCT01174199) to compare vorinostat to intravenous temsirolimus in individuals with metastatic disease. The “Total Androgen-Receptor Gene Expression Targeted Therapy (TARGET) trial,” a National Cancer Institute phase II study (NCT00589472), evaluates neoadjuvant vorinostat with oral bicalutamide and intramuscular leuprolide acetate or subcutaneous goserelin acetate 4–8 weeks prior to radical prostatectomy. FT-7051 is an orally available, effective, and selective CBP/p300 inhibitor that has shown promise in preclinical models of prostate cancer, particularly those resistant to AR inhibitors like enzalutamide. The Courage Study (NCT04575766) is a multicenter, phase 1 open-label study evaluating the safety, pharmacokinetics (PK), preliminary anti-tumor activity, and pharmacodynamics (PD) of FT-7051 in men with metastatic castration-resistant prostate cancer (mCRPC) who have progressed amidst prior treatment and have been treated with at least one approved androgen receptor pathway inhibitor (NCT04575766).
In mCRPC patients whose disease has progressed on prior abiraterone or enzalutamide, a phase Ib open-label, dose-escalation study was conducted to assess the safety and efficacy of oral administration of GSK525762 in combination with either abiraterone plus prednisone (Arm A) or enzalutamide (Arm B) (Vaishampayan et al., 2018). In metastatic CRPC, a phase Ib/IIa clinical trial combining the BETi PLX2853 with abiraterone or olaparib has recently been begun (NCT04556617). Finally, a new phase II trial in men with CRPC (NCT04471974) combines enzalutamide (ZEN003694) and the immune checkpoint inhibitor pembrolizumab (NCT04471974). This trial comprises a group of patients with NEPC tumors. These findings show that BETi is a viable strategy worthy of further investigation. The possibility of combining BETi with immunotherapy or AR targeting medicines to treat advanced prostate cancer will be determined by the results of ongoing clinical trials.
Panobinostat (LBH589), an HDACi, resensitized CRPC models that had undergone EMT to ADT (Ruscetti et al., 2016). Despite these encouraging preclinical findings, clinical trials of single-agent HDACi have failed to show meaningful effectiveness (Eigl et al., 2015). Furthermore, a recent clinical trial with panobinostatin conjunction with the AR inhibitor bicalutamide revealed that the combination could be used to resensitize tumors to AR inhibition (Ferrari et al., 2019). In another phase Ib/IIa research, patients with metastatic CRPC who were resistant to enzalutamide and/or abiraterone were given enzalutamide and the BETi ZEN003694 (NCT02711956). In a subgroup of patients, the combination of enzalutamide and ZEN003694 was well tolerated and resulted in a prolonged PFS (Aggarwal et al., 2020).
Pracinostat, which has been designated as an orphan medication by the FDA for the treatment of AML, was also tested in a clinical phase 2 trial with 32 patients with CRPC. Even though just two patients had PSA reductions of more than 50%, the medicine (60 mg, 3 times per week, p. o.) was well tolerated, led to stable disease in 22% of patients, and reduced the amount of circulating tumor cells in nine others (Eigl et al., 2015). A phase 2 clinical trial with 35 CRPC patients tested the depsipeptide romidepsin. Two patients had a radiological partial response, 11 had stable disease, and 22 had progressive disease after receiving intravenous romidepsin (13 mg/m2). Despite the absence of grade 4 complications, 11 patients had to drop out of the study early, and romidepsin was not advised for additional phase 3 trials in CRPC (Molife et al., 2010). Vorinostat and romidepsin, on the other hand, caused drug-induced toxicities in a significant proportion of patients, forcing them to discontinue treatment. The relatively poor responses induced by the aforesaid first-generation HDACi when administered as a monotherapy in CRPC patients is the reason why no phase 3 studies in CRPC patients have been performed yet.
Breast cancer
Breast cancer, a heterogeneous illness, is the most commonly diagnosed cancer in women globally and the second greatest cause of cancer-related death (Siegel et al., 2019). Breast cancer affects roughly 12% of American women during their lifetime, according to the American Cancer Society. Furthermore, approximately 2,300 men were diagnosed with breast cancer in 2015, with 440 dying as a result of the disease (DeSantis et al., 2015; Nyante et al., 2017). Males are not immune to breast cancer. Males usually have worse outcomes than females because to delays in diagnosis and estimates (Rizzolo et al., 2013; Kochan and Kovalchuk, 2015). The current classification of breast cancer is based on molecular subtypes, which reflect the tumor’s hormone response (Cava et al., 2015). Breast cancer can be divided into two primary classes and four groups based on particular molecular subtypes. Breast cancer can be divided into four intrinsic subtypes based on gene expression profiling: luminal A, luminal B (Luminal B1 and Luminal B2), HER2 loaded, and basal-like (Table 5). (Fragomeni et al., 2018). Estrogen receptor (ER), progesterone receptor (PR), or the human epidermal growth factor receptor2 (HER2/ERBB2) protooncogenic receptor are expressed in around 90% of breast cancer patients. In the luminal A subtype, mutations in GATA3, PIK3CA, and MAP3K1 were frequently found (Koboldt et al., 2012). The Luminal B2 subtype of HER2-positive breast cancer, which overexpressed GATA3, BCL2, and ESR1 genes, was found to account for roughly half of all HER2-positive breast cancer subtypes (Wu et al., 2015). The frequency of p53 mutations was higher in luminal B1 than in luminal A, although the prevalence of PIK3CA mutations was lower.
The overexpressed genes in the luminal subtype were down-regulated or deleted in the HER2 positive subtype. There is no clinically-proven type-specific therapeutic target for the 10% of breast cancer cases that are negative for ER, PR, and HER2, and hence are designated “triplenegative,” and only genotoxic chemotherapy is utilised (Sørlie et al., 2001). TNBC is a more aggressive subtype of breast cancer that, regrettably, remains a clinical challenge to treat due to its poor prognosis, aggressiveness, and lack of targeted medicines. TNBC is divided into six molecular subtypes: two basal like classes (BL1 and BL2), immunomodulatory (IM), mesenchymal (M), mesenchymal stem cell (MSL), and luminal androgen receptor (LAR) (Eckstein, 2011). Anti-estrogens (e.g., aromatase inhibitors, tamoxifen, fulvestrant) and HER2-targeted medicines have greatly increased their survival (Giuliano et al., 2011; Rexer and Arteaga, 2012). However, some tumors grow de novo or gain resistance to anti-estrogen and HER2-targeted medicines despite these treatments, and these tumors can recur (Tryfonidis et al., 2016). In order to treat breast cancer, new therapeutic targets must be developed, given the disease’s high prevalence and severity.
Development HDACi against breast carcinoma
In 2016, Peng et al. designed and synthesized eighteen N-phenylquinazolin-4- amine hybrids as dual inhibitors of vascular endothelial growth factor receptor 2 (VEGFR-2) and HDACs considering the roles of these receptors in cancer progression (Peng et al., 2016). Upon interacting with its endogenous substrate VEGF, the tyrosine kinase receptor VEGFR-2 initiates downstream signaling leading to tumor angiogenesis, proliferation and migration. Being a promising target of cancer therapy, several agents have been developed as VEGFR-2 though frequent emergence of drug resistance that restricted their therapeutic potential. Therefore, the development of multitarget inhibitors with activity against both VEGFR-2 and HDACs may be promising strategy. The designing strategy adopted by the investigators was simple and interesting as they attempted to combine the aromatic moiety of vandetanib (a VEGFR-2 inhibitor) with the side chain of HDACi vorinostat. Among 18 synthesized derivatives, one compound (VII, Figure 2) depicted IC50 values of 2.2 nM and 74 nM against HDACs (HELA cell nuclear extract containing the mixtures of HDACs) and VEGFR-2, respectively. At the same time the cell proliferation assay revealed that this compound is active against breast carcinoma cell line MCF-7 with IC50 of 0.85 µM. When this compound was tested against different HDAC isoforms such as HDAC-1, HDAC-2, HDAC-6 and HDAC-8, maximum potency was observed against HDAC-1 (i.e., IC50 = 1.8 nM) followed by HDAC-2 (i.e., IC50: 3.3 nM), HDAC-8 (i.e., IC50: 4.6 nM) and HDAC-6 (i.e., IC50: 16.4 nM). In the same year, Gromek and co-workers (Gromek et al., 2016) attempted to develop potent HDACi with a hybrid structure (SCA-vorinostat, Shown in Figure 2, VIII) formed with vorinostat and santacruzamate A (SCA), a natural HDACi (previously identified by the investigators) with micromolar potencies against various isoforms of HDACs. Similar to SCA, SCA-vorinostat was found to be active against multiple HDAC isoforms and forty derivatives of these compounds were prepared. When these hybrids compounds were tested against breast carcinoma cells, two silylated derivatives were found to have promising antiproliferative activity against MCF-7 cell line and interestingly the potency (GI50 of 13.3 and 23.7 nM) of these compounds against this breast carcinoma cell line was even higher than that of vorinostat (i.e., GI50 29.1 nM). Interestingly, these compounds were in fact tested against a panel of cell lines that also included triple negative breast carcinoma cell MDA-MB-231, colon carcinoma cell HCT-116, lymphoma cells (Hut-78 and Molt-4) as well as against non-cancerous peripheral blood mononuclear cell PBMC. However, no significant potency was noted against any of these cell lines including MDA-MB-231 indicating that these compounds may selectively inhibit normal breast carcinoma cells following unique mechanisms. In 2016, Saha et al. (2016) reported one investigation where twelve compounds containing triazole and hydroxyacetamide moieties that were tested against HDACs and MCF-7 cells. The most potent HDACi in this series depicted IC50 of 90 nM which is close to the potency of vorinostat (IC50: 56 nM) but the HDAC inhibitory activity did not match well with antiproliferative activity of these derivatives against MCF-7 that range from growth inhibitory (GI50) values of 20–60 µM. In 2018, one research investigation was reported by Yamashita et al. (2018) describing the design of dual inhibitors of HDACs and DNA topoisomerase II. The latter enzyme is a well-known target for inhibition of cancer progression due to its role in the regulation of DNA topology. Some hybrid compounds were prepared by combining the side chain of HDACi Trichostatin and a lactone compound that was proved to be a potent inhibitor of DNA topoisomerase II. One of these hybrids was found to be dual inhibitors of both enzymes and further modification of this structures led to six of its derivatives that were found to be potent inhibitors of DNA topoisomerase II as well as HDACs and at the same time, these were also found to be antiproliferative against breast (MCF-7), colon (HCT-116) and prostate (DU-145) cancer cells. For example, the most potent compound of this series (IX, Figure 1) had growth inhibitory (GI50) values of 3.24 µM, 3.39 µM and 3.98 µM against MCF-7, HCT-116 and DU-145. Another important investigation was reported in the same year (Chen et al., 2019), where a series of methylquinazoline derivatives were rationally designed based on previously conducted in silico fragment-based analyses that had projected this fragment as a potential surface recognition cap group. The synthesized compounds were first tested for their antiproliferative activity against colon cancer cell line HCT116 and based on the results, eleven compounds were picked for enzymatic assays against HDAC1 and HDAC6. Significantly all these compounds depicted nanomolar potency against these enzyme isoforms and more importantly, these compounds also had sufficient selectivity towards these two specific HDAC isoforms. A series of solid and hematologic tumor cell lines were then chosen to estimate the overall antiproliferative potential of some of these inhibitors and two compounds depicted IC50 values of 2.65 and 7.41 nM against MCF-7 cell line. Based on MCF-7/ADR xenograft model, one compound (X, Figure 2) was finally projected as a potential lead molecule for cancer therapy. Focusing on the treatment of triple negative breast carcinoma, Yao et al. (2020) later selected the same quinazoline moiety to synthesize more than forty derivatives and each of these was tested against HDAC-6 by enzymatic assay and MDA-MB-231 by cell viability assay. Two compounds with high inhibitory potential against both these targets were then selected for pharmacological assays where these were proved to promote autophagy, apoptosis while suppressing migration of this breast carcinoma cells. More significantly, improved pharmacokinetic profiles were noted with these derivatives as compared to vorinostat. Two of the most potent compounds (XI and XII) in this series are shown in Figure 2.
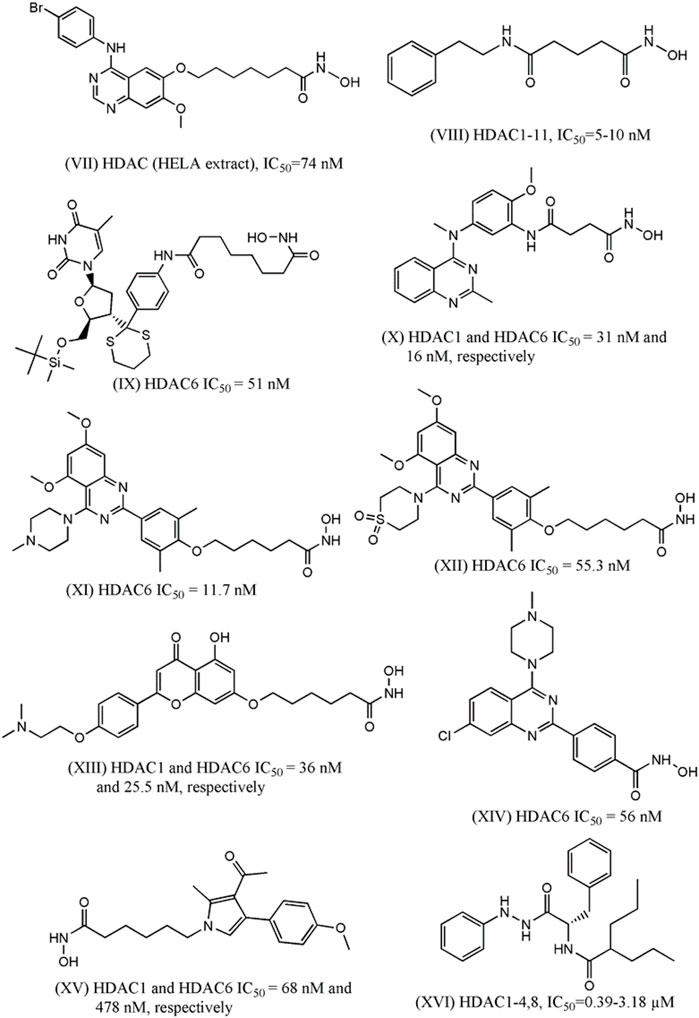
FIGURE 2. Structures of HDACi designed in recent years that depicted anti-proliferative potential against the breast carcinoma cell lines.
More recently, Wei et al. (2020) designed some flavone- and isoflavone-based HDACi considering pharmacological versatility of these moieties. Choosing these moieties as hydrophobic capping groups, more than 25 derivatives were synthesized and tested for inhibitory potential against HDACs. The most potent one (XIII, Figure 2) was then tested for isoform selectivity that led to the observation that its more selective towards HDAC-1,2,3, and 6. A number of biological assays were then conducted to establish strong antiproliferative activity of this lead molecule against multiple TNBC cells (i.e., MDA-MB-231, MDA-MB-468, BT-549, and Sum-159), HER-2 negative breast carcinoma cell (i.e., MCF-7, T47D), breast carcinoma cell (i.e., BCAP-37), pancreatic carcinoma (i.e., PANC-1, PC-3), melanoma, hepatocarcinoma, lung carcinoma as well as normal human cell lines. The same compound, with improved pharmacokinetic profiles, was found to downregulate HDAC-induced STAT3 in vivo in some breast cancer cells to enhance anti-tumor activity.
In another investigation (García et al., 2020), fluorescent coumarin-hydroxamic acid derivatives were designed as HDACi. The designed compounds, which were actually hybrids of vorinostat and coumarin, were initially tested against two breast carcinoma (i.e., BT-474 and MDA-MB-231) and one prostate cancer (i.e., PC-3) cell lines to choose the most potential hits and some of these were found to downregulate the expressions of cell-cycle regulatory genes such as p21, p53 and cyclin D1 (CD1) in both breast and prostate cancer cells. However, enzyme inhibition activity against any HDAC of these derivatives were not reported though molecular docking analyses hinted that some of these compounds may bind to the catalytic sites of class I HDACs.
The role of quinazoline moiety was further investigated by Yao et al. (2021) with a purpose to design dual mTOR/HDACi. The mTOR is one of the key members in pI3K-AKT-mTOR pathway that have been implicated in the progress of several carcinomas. The investigators correctly identified that quinazoline moiety, which is present in the very potent mTOR inhibitor KU-0063794, may be explored to develop hybrid structures with activity against mTOR and HDACs. A total 28 derivatives were synthesized and tested against mTOR and HDAC6 but maximum inhibitory potential was obtained with a compound (shown in Figure 2 as XIV) that depicted IC50 of 56 nM against HDACs and IC50 of 133.7 nM against mTOR. This compound depicted promising anti-proliferative activity against TNBC cell like MDA-MB-231 and at the same time also promoted autophagy and apoptosis in this cell in a dose-dependent manner. The anti-migratory action was also noted in the cells treated with this compound. Very recently Singh and co-workers (Singh et al., 2021) attempted to develop some pyrrole based HDACi keeping the hydroxylamine moiety intact as zinc binder domain. After synthesizing a series of 4-substituted-methyl 6-(3-acetyl-2-methyl-1H-pyrrol-1-yl)-hexanoate and 4-substituted-6-(3-acetyl-2-methyl-1H-pyrrol-1-yl)-N-hydroxyhexanamide derivatives, some most potent derivatives were tested against breast cancer cell line MCF-7 along with leukemia, lung and cervical cancer cell lines. One compound (XV, Figure 2) depicted total growth inhibition (TGI) at 53.7 µg/ml and this compound also showed IC50 of 68 nM and 478 nM against HDAC-1 and HDAC-6, respectively. While designing novel HDACi, hydroxamic acid remained the most frequent choice as zinc binding group even though this group may be replaced with other zinc binding groups as well. Recently, Ibrahim et al. (2020) designed structurally diverse HDACi that consisted of valproic acid as the cap group whereas these designed compounds contained a range of zinc binding groups (e.g., carboxy, hydrazine, etc) as zinc binding domain. These valproic acid conjugates were tested for their antiproliferative activity with multiple human solid cancer cell lines including breast cancer cell MCF-7. Based on the cytotoxicity results, a few compounds were then assayed against nine different HDAC isoforms (i.e., HDAC-1-9) to assure their potency and selectivity towards class I HDAC enzyme isoforms. One compound (XVI, Figure 2) was then selected for cell cycle analyses conducted with MCF-7 cells to infer that it is likely to lead towards Pre-G1 apoptosis and cell growth arrest at G2/M. Furthermore, the investigators also resorted to in vivo assay to confirm that this novel compound is capable of reducing the size of tumor as well as number of tumor cells in mice model. In recent reports, Bingul et al. (2021) helped identifying some indole derivatives to enhance the cytotoxic efficiency of vorinostat against breast cancer cells such as MCF-7 and MDA-MB-231.
Histone deacetylases and breast cancer
At the estrogen- and progesterone-mediated signaling pathways, HDACs play a crucial role in transcriptional control. Acetylation has been discovered to be a crucial mediator at numerous locations along this pathway, affecting both ER transcription and turnover (Thomas and Munster, 2009). The development of breast cancer has been linked to a disruption in the equilibrium between HATs and HDACs. Zhang et al. (2004) discovered that hormone-positive breast cancer patients with small tumors and low histological grade had increased expression of HDAC6 mRNA, indicating that they were more receptive to endocrine treatment and had a better prognosis. Krusche proposed HDAC 1 as an independent breast cancer prognostic marker in 2005. HDAC 1 expression profiling could be relevant in the clinic to help patients with breast cancer receive individualized, risk-directed adjuvant systemic therapy (Krusche et al., 2005). HDAC4 upregulation was discovered in breast cancer cells in 2006, compared to lung and colon cancer cells (Özdağ et al., 2006). Lee et al. (2008) approved the potential function of HDAC6 in anchorage independent breast cancer cell proliferation. Ververis and Karagiannis (2012) found higher expression of Class I HDACs in breast cancer tissue than Class II enzymes. HDACs inhibits the expression of GABARAPL1, an autophagy-related gene, and promotes breast cancer development (Hervouet et al., 2015). Furthermore, tumor differentiation and tumor cell proliferation are linked to the expression levels of the histone-modifying enzymes HDAC2, LSD1, and SIRT1 (Derr et al., 2014). The global reduction of monoacetylated lysine 16 of histone H4 (H4K16) is a common occurrence in cancer, and low levels of H4K16 acetylation have been indicated as an early event in breast cancer (Falahi et al., 2014). Suziki et al. discovered that ductal carcinoma in situ and invasive ductal carcinoma have lower levels of ac-H4 and ac-H4K12 acetylation than normal breast epithelium (Karsli-Ceppioglu et al., 2014). H3K4ac has been linked to both early and late phenotypes of breast cancer cells. H3K4ac enrichment is seen at promoters of genes linked to cancer-related phenotypic features like oestrogen response and epithelial-to-mesenchymal transition pathways (Messier et al., 2016). As a result, HDACs are critical for breast cancer pathogenesis and progression, giving new therapy options for the disease.
Preclinical studies of HDACi in breast cancer
HDACi have been tested in all breast cancer subtypes because preclinical research shows that this class of drug can target breast cancer in a variety of ways, including relief of transcriptional repression with an impact on the epithelial-mesenchymal transition (EMT), reactivation of silenced oestrogen receptor (ER) in hormone receptor-negative tumors, restoring the sensitivity of hormonal therapy in estrogen-positive tumors, and modulation of transcriptional repression with an impact on the epi (Connolly et al., 2017). It was previously reported that HDACi regulate 8%–20% of genes at the transcriptional level by inhibiting HDACs function on histone tails, and that they could also target gene transcription via an indirect mechanism by inhibiting HDACs interactions with non-histone proteins, as HDACs act on a variety of proteins other than histones, including transcription factors, enzymes, and HDACs themselves (Glaser et al., 2003). HDACi inhibits HDACs activity, resulting in hyper-acetylation of histone lysine residues. HDACs plays a critical role in breast cancer treatment (Contreras-Leal et al., 2016). HDACs block HDAC’s catalytic activity by chelating the zinc co-enzyme factor. HDACi can inhibit breast cancer in a variety of ways, and they can also help with the therapy of breast cancer. HDACi has the potential to disrupt cell mitosis. HDACi CG-1521 inhibits the production of mitotic spindles and prevents abscission during cytokinesis, which leads to death in inflammatory breast cancer cells (Chatterjee et al., 2013). HDACi are also known to induce apoptosis by regulating anti- and/or pro-apoptotic molecules such as the Bcl-2 family of molecules (Johnstone and Licht, 2003), and the mechanism underlying the suppression of Bcl-XL protein by HDACi has been reported to be regulated at the transcriptional or translational level in several carcinomas, including TNBC (Johnstone and Licht, 2003). Although HDACi appear to be potential anti-cancer medications in theory, their usage as monotherapy for solid tumors is still limited, and most trials examining them combine them with another anti-cancer drug (Wang et al., 2016). Preclinical evidence suggests that HDACi, as a single drug candidate or in combination with other anticancer medicines, have a wide range of anticancer effects in several cancer cell lines and cancer xenograft models by targeting many cancer pathways.
Vorinostat, TSA, belinostat, panobinostat, givinostat, resminostat, abexinostat, and quisinostat are examples of pan-HDACi, while ricolinostat, pracinostat, and CHR-3996 are instances of selective hydroxamates. Vorinostat, TSA, panobinostat, and belinostat have all been studied extensively in different BC cell models. The FDA has approved vorinostat and panobinostat for the treatment of cutaneous T-cell lymphoma and multiple myeloma, respectively (Andreu Vieyra and Berenson, 2014). Three HDACi, vorinostat, and panobinostat, have been approved by the FDA and are currently being tested in clinical trials for breast cancer patients as a single agent or in combination with other standard therapies such as chemotherapies, aromatase inhibitors (exemestane), or SERM (tamoxifen) (Munster et al., 2011). Vorinostat is a hydroxamic acid-based HDACi that has been proven to block the majority of HDACs (class I, II, and IV), making it a pan inhibitor. Vorinostat inhibited the proliferation of TAMR/MCF-7 BC cells (tamoxifen-resistant) via inducing apoptosis and autophagic cell death, according to Lee et al. In mice with TAMR/MCF-7 tumors, vorinostat also inhibited tumor cell proliferation (Lee et al., 2012). In a mouse model of triple-negative BC, Palmieri et al. (2009) found that vorinostat can generate dsDNA breaks and can prevent brain metastasis (62% large metastases compared to untreated groups). Vorinostat can activate heat shock protein (hsp)90 hyperacetylation, diminish hsp90 binding to ERα, increase polyubiquitylation, and decrease ERα expression in ERα-positive BC cells, according to Fiskus et al. (Fiskus et al., 2007). Vorinostat was also discovered to limit the capacity of 4T1 BC cells to migrate and invade. Vorinostat inhibited 4T1-luc cell metastasis in vivo, according to the results of the same study’s in vivo trials (Chiu et al., 2013). Terranova-Barberio et al. found that vorinostat therapy resulted in an 18-fold increase in PD-L1 and HLA-DR expression in triple-negative BC cells (Terranova-Barberio et al., 2017).
TSA is an HDACi that comes from nature (Damaskos et al., 2017). TSA was discovered to make hormone-receptor-negative BC cells receptive to tamoxifen by altering the transcriptional activity of ER in ER-negative BC cells (Jang et al., 2004). Chen et al. (2017) have revealed that co-treatment of TSA with BEZ235 (a PI3K/mTOR/AKT pathway inhibitor) can cause apoptosis and mediate strong anticancer effects in MCF-7, T47D, and MDA-MB-231 BC cells. The same study also found that co-treatment of TSA with BEZ235 inhibited the growth of MDA-MB-231 tumors in mouse xenograft models. TSA and CG-1521 (hydroxamate-based HDACi) can induce apoptosis and cause cell cycle arrest in SUM149PT and SUM190PT inflammatory BC cell lines, according to Chatterjee et al. (2013). Panobinostat can greatly increase histone acetylation, cell cycle arrest, and trigger apoptosis in BC cells, as per preclinical studies. Tate et al. (2012) found that panobinostat can reduce proliferation and induce histone acetylation in triple-negative BC cell lines MDA MB157, MDA-MB-231, MDA-MB-468, and BT-549. Panobinostat can also re-express the silenced ER gene in triple-negative BC cells via restoring heterochromatin-associated proteins without promoter hypermethylation, according to Zhou et al. (2007). Furthermore, a study found that treating hormone-responsive BC cells with panobinostat and letrozole together suppressed aromatase expression synergistically, implying that panobinostat and letrozole combined therapy is an appropriate way to target hormone receptor-positive/aromatase-positive BC cells (Chen et al., 2010).
Another FDA-approved medication for the treatment of PTCL is belinostat. Hsu et al. recently found that belinostat inhibited the proliferation of MDA-MB-231, SKBR-3, and MCF-7 BC cell lines and triggered apoptosis in a caspase-dependent manner (Hsu et al., 2018). Belinostat or vorinostat were also reported to reduce the proliferation of a triple-negative BC cell line panel (8 cell lines) and tumor growth in triple-negative BC xenografts when used in combination with olaparib (an FDA-approved anticancer medication) (Marijon et al., 2018). In MCF-7 BC cells treated with belinostat, Androutsopoulos and Spandidos observed reduction of cell growth, strong HDACs inhibition, and elevation of acetylated tubulin levels (Androutsopoulos and Spandidos 2017). Valproic acid (VPA) is a valeric acid-derived short-chain fatty acid (Damaskos et al., 2017). Tian et al. (2017) recently discovered that VPA and hydroxyurea (a ribonucleotide reductase inhibitor) can limit the proliferation of MCF-7 BC cells synergistically by inhibiting RPA2 and increasing the Rad51-mediated homologous recombination DNA repair pathway. By suppressing the activity of HDAC1 and modifying the methylation state of H19 by induction of the enzyme DNA methyltransferase 1 (DNMT1) expression, Hao et al. (2017) found that VPA may induce death in A549 BC cells and reduce the production of the H19 oncogene.
The sodium salt of butyric acid is designated as NAB (Smith et al., 1998). NAB can trigger apoptosis in MCF-7 and MDA-MB-468 BC cell lines via oxidative stress, according to a recent study (Salimi et al., 2017). The similar effects of NAB have been demonstrated in MCF-7 BC cells by Louis et al. (Louis et al., 2004). Furthermore, via regulating Fas signaling, NAB has been shown to cause apoptosis in MCF-BC cells in a p53-independent way (Chopin et al., 2002). Entinostat is a class I HDACi that is synthesised (Knipstein and Gore, 2011). Schech et al. (2015) found that entinostat significantly reduced TICs from triple-negative BC cells in a study. Furthermore, entinostat treatment decreased the number of CD44 (high)/CD24 (low) cancer stem cells, aldehyde dehydrogenase 1 (ALDH1) levels, and TIC marker expression (Oct-4, Bmi-1 and Nanog). According to Shah et al. (2014), entinostat can reverse epithelial-mesenchymal transition (EMT) in triple-negative BC cells by decreasing the binding of transcription factors Snail and Twist to the E-cadherin promoter. Entinostat was discovered to sensitise HER2-positive BC cells to trastuzumab/lapatinib treatment, supporting the use of entinostat/lapatinib and trastuzumab in HER2-positive BC patients.
Cyclic tetrapeptides are natural chemicals found in fungi and bacteria from the sea (Li and Seto, 2016). Romidepsin is a selective class I HDACi that has been approved by the FDA for the treatment of CTCL patients. Primary and metastatic cancers were effectively suppressed by a combination of romidepsin and paclitaxel treatment. In another study, romidepsin and oncogenic H-Ras stimulated the ERK pathway in H-Ras-transfected MCF10A mammary cells, resulting in the activation of Nox-1 and reactive oxygen species (ROS) and apoptosis (Choudhary et al., 2010). Several cancer cell models have been used to test the preclinical efficacy of sirtuin inhibitors such sirtinol, cambinol, EX527, and suramin. By decreasing the expression of SIRT1/2 in MCF-7 BC cells, Wang et al. found that sirtinol can trigger apoptotic and autophagic cell death (Wang et al., 2012). In sirtinol-treated MCF-7 BC cells, researchers were able to induce a senescence-like growth arrest by inhibiting the Ras/MAPK signaling pathway (Ota et al., 2006). Sirtuins are located in a variety of subcellular sites in mammals, including the nucleus (SIRT1, 2, 6, and 7), mitochondria (SIRT3, 4 and 5), and cytoplasm (SIRT1 and 2), and are thought to play a role in cell survival, metabolism, ageing, and genetic integrity (Haigis and Sinclair, 2010).
Registered HDACi in clinical trials of breast cancer
HDACi have failed to exhibit highly effective anticancer efficacy in breast cancer clinical trials as single medicines. HDACi, on the other hand, have become a popular component of breast cancer treatment regimens (Trapani et al., 2017). Hormone therapy resistance is a problem in treating estrogen receptor (ER) positive breast tumors, hence researchers have looked into using HDACi in combination with hormone therapy. The first clinical trial combining vorinostat and tamoxifen for hormone therapy-resistant breast cancer was completed by researchers (NCT00365599) (Munster et al., 2011). This study looked at restoring hormone sensitivity to tamoxifen in advanced breast cancer patients who had progressed on prior hormone therapy. The 43 individuals who participated in this study had their H4 acetylation and HDAC2 expression induced by vorinostat in their peripheral blood mononuclear cells. The addition of the HDACi vorinostat to tamoxifen hormone receptor-positive breast tumors resulted in tumor regression or prolonged disease stability in 40% of patients who had progressed on earlier hormonal therapy and chemotherapy, as per the findings.
Entinostat decreased MDSCs and the regulation of MDSC CD40 expression in breast cancer patients, while also increasing HLA-DR expression on CD14+ monocytes. These findings support the use of entinostat in conjunction with immune checkpoint inhibitors as a treatment option (Tomita et al., 2016). In addition, a phase III trial in hormone receptor-positive breast cancer patients will soon begin assessing endocrine therapy with the HDACi, entinostat, or placebo (NCT02115282). Vorinostat was studied as a single drug in early BC clinical investigations. However, HDACi’s overall clinical effectiveness as single treatments in solid tumors has not always been favourable. Rubin et al. (2006) conducted a Phase I research in patients with advanced cancer, including BC, to assess the safety, tolerability, and pharmacokinetics of single and multiple doses of vorinostat, as well as the effects of a high-fat diet on vorinostat pharmacokinetics. The results of research showed that one (stage IV BC) of four BC patients who took vorinostat [400 mg on days 1–20 (fasted) and 5 (fed)] and were fed a high fat diet for >15 months maintained stable disease (SD).
In addition, patients with advanced HR-positive, HER2-negative breast cancer whose disease progressed after nonsteroidal aromatase inhibitors were used were enrolled in a multicenter, randomised, double-blind, placebo-controlled phase III research (E2112). This phase III clinical trial was based on the results of a prior ENCORE301 phase II investigation, which found that combining entinostat and exemestane therapy improved PFS and OS (Yardley et al., 2013). Patients were given either oral 25 mg exemestane once daily and 5 mg entinostat or placebo 5 mg once weekly in this trial. According to the findings, the median PFS of exemestane plus entinostat was 3.3 months and 3.1 months, respectively, with no significant difference.
In metastatic advanced triple-negative breast cancer (TNBC) patients, a phase II single-arm clinical trial was done to examine the efficacy and safety of a combination treatment of tucidinostat/chidamide with cisplatin. Tucidinostat/chidamide and cisplatin, 20 mg twice weekly for 2 weeks and 75 mg/m2 on a 21-days cycle, were given to women with TNBC (Meng et al., 2021). Table 6 lists some of the most recent clinical trials for breast cancer treatment.
Ovarian cancer
Most ovarian cancers are either ovarian epithelial cancers (cancer that begins in the cells on the surface of the ovary) or malignant germ cell tumors (cancer that begins in egg cells). With a survival rate of 47%, malignant ovarian cancer has the highest mortality rate compared to other cancers of gynecological origin (Moufarrij et al., 2019). The most characteristic trait of which is a papillary serous in its histology. The ovarian cancers are thus subdivided into high grade and low grade serous ovarian cancers. The incidence of about 14%–20% of all ovarian cancers are attributed to hereditary predisposition, mutations of specific genes (BRCA 1 & 2) and the loss of their function or genes encoding proteins that complex with BRCA proteins, such as BRIP1, RAD51C, RAD51D, and FANCM, which are responsible for mechanisms of DNA repair, along with the loss of mismatch repair function is what causes the genetic instability. This instability along with mutations in the tumor suppressor gene (Tumor protein-TP53) leads to high grade EOC (Moufarrij et al., 2019).
The major issue in treating ovarian cancer is its late diagnosis, causing the treatment to begin in advanced stages (stage III or IV). This is due to absence of distinct symptoms specific to early stage EOC and the lack of biomarkers for screening of EOC (Yang et al., 2018). The usual treatment protocol consists of surgery and cytoreduction for reduction of tumor volume followed by chemotherapy with a platinum agent and a taxane. Even though the initial response shows great promise, resistance to chemotherapy poses problems in treating ovarian cancers, caused by recurrent tumors. Many other targeted therapies have been used, namely PARP inhibitors, which targets angiogenesis, however the response was unsatisfactory (Yang et al., 2018). The heterogeneous nature of ovarian cancer makes selection of the right drug very difficult, suggesting that a multi-targeted treatment approach would be more effective in treating ovarian cancer. The limitations of the aforementioned mentioned therapies led the way for the use of novel epigenetic therapies to treat ovarian cancer (Khabele, 2014).
Development HDACi against ovarian carcinoma
Only a few investigations were reported in recent years where ovarian carcinoma cells were tested for HDACi. In 2017, an investigation (Stenzel et al., 2017) was reported by Stenzel and co-workers (2017) that involved syntheses of aloxyurea-based HDACi to improve the potency of cisplatin in various chemo-resistant cancer cells. These aloxyurea derivatives, which contained structural similarity with ricolinostat, depicted selective inhibition of HDAC-1 and HDAC-6 over other HDACs and also displayed satisfactory inhibitory potential against ovarian cancer cells A2780 and A2780CisR. Further studies conducted with chemo-resistant cancer cells (i.e., A2780CisR and Cal27CisR) revealed that some of these potent derivatives actually increase the potency of cisplatin in a synergistic manner. Further, Andrade et al. (2018) explored the moiety of natural HDACi santacruzamate A to produce seven synthetic compounds that were tested against a panel of human cancer lines that included breast cancer cells MCF-7 and MDA-MB-231 as well as ovarian cancer cell TOV-21G to have moderate micromolar potencies. Even though in vitro potencies of these compounds against HDACs were not reported, further investigation revealed that the most potent compound of this series may indeed enhance DNA damage and may promote apoptotic cell death through intrinsic pathway.
HDACs and ovarian cancer
HDACs 1, 2, and 3 i.e., belonging to class I are over-expressed in ovarian cancer cells, namely high grade serous, mucinous, endometrioid as well as clear cell type of EOC and promote carcinogenesis (Yano et al., 2018). The role of HDACs in ovarian cancer is depicted in Figure 3. It has been observed that HDACs belonging to class I are over-expressed in tumor cells with high proliferative activity and this is also the underlying cause of poor prognosis of malignant ovarian tumor (Eckschlager et al., 2017). Regulator of G-protein Signaling 2 (RGS2) inhibits G-protein coupled receptors (GPCRs) by causing the deactivation of heterotrimeric G-proteins. It has been observed that RGS2 levels dropped sharply in chemo-resistant ovarian epithelial cells as compared to chemo-sensitive cells. HDAC1 are shown to down-regulate this RGS2 along with the promotion of cyclin A, thereby enhancing cellular proliferation (Smith et al., 2017). Resistance to platinum-based chemical therapies in EOCs is achieved by chromatin remodeling via HDAC2 (Yang et al., 2018). Suppression of E-cadherin is facilitated by HDAC3 expression that enhances cellular migration which is essential in metastasis of malignant ovarian cancer (Smith et al., 2017). OX-40 L and 4-1BBL are responsible for regulating the activity of effector cytotoxic T-cells whereas immunosuppressive effects are exhibited by PD-L1(Programmed death ligand 1), protecting the tumor cells to form immune destruction (Takai et al., 2007). HDAC 1 and 3 enhance suppression of OX-40 L and 4-1BBL in chemotherapy-resistant ovarian cancer cells. Additionally, HDAC3 has a role in inflammation whereas HDAC4 facilitates proliferation, invasion potential and migration of ovarian cancer cells by suppressing p21, while HDAC6 is over-expressed in ARID1A-mutated EOCs. Intriguingly, HDAC1 and 7 are over-expressed in cancer stem cells, whereas, HDAC9 and 10 are both essential for homologous recombination in malignant ovarian tumors (Yang et al., 2018). The role HDACi in ovarian cancer is depicted in Figure 4.
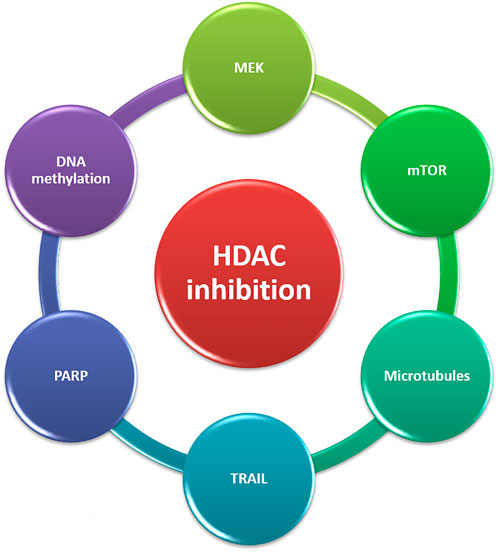
FIGURE 3. Targets for combination therapies with HDACi in CRPC. CRPC, castration-resistant prostate cancer; HDACi, Histone deacetylase inhibitors; TRAIL, TNF-related apoptosis-inducing ligand; MEK, MAPK/ERK kinase.
Pre-clinical studies on HDACi in ovarian cancer
Trichostatin A (TSA) is under preclinical stage for ovarian cancer treatment. It has been investigated as a potent anti-tumor agent in a variety of cancers, including ovarian cancer, inhibits both HDACs 1 and 2, induces gene expression of P73 as well as induces Bax-dependent apoptosis (Yano et al., 2018). Additionally, TSA is also involved in the inhibition of VEGF-induced expression of VEGF receptors Nrp1, VEGFR1 and VEGFR2. Moreover, TSA and vorinostat regulate the SEMA3 (VEGF protein competitor) expression, at both mRNA and protein levels (Takai and Narahara, 2010). Whereas, valproic acid (VPA), owing to its anti-angiogenic property, induces down regulation of endothelial nitric oxide synthase (eNOS) in endothelial cells (Eckschlager et al., 2017). The combination of VPA, 5- Azacytidine and carboplatin has been studied by Falchook et al. (2013), but it showed high toxicity. Various cell culture models elucidated that the exposure to VPA results in dose-dependent cell cycle arrest as well as apoptosis in ovarian cancer cell lines (Takai and Narahara, 2010). The ability of VPA to inhibit the growth of human SK-OV-3 ovarian cancer tumors was tested in immunodeficient mice and it was observed that VPA suppresses the tumor growth remarkably along with no observable adverse effects. The studies demonstrated by Qian et al. (2012) revealed that PXD101 in combination with carboplatin cause enhancement in the anti-tumor activity tested on human A2780 ovarian xenografts whereas, paclitaxel in combination vorinostat enhance the anticancer effect as compared to vorinostat as a single agent (Hontecillas-Prieto et al., 2020).
Vorinostatis an HDACi, which has been successfully tested against EOCs (Smith et al., 2017). In clinical trials, vorinostat appeared to be one of the most promising HDACi in the treatment of EOC, as compared to all other HDACi. It proves to be effective in treating ovarian cancer, either individually or in combination with anti-cancer drugs like cisplatin (Moufarrij et al., 2019). About 6 ovarian cancer cell lines were tested against TSA, vorinostat, VPA and NaB, it was found that all the cell lines were sensitive to the drugs, it was observed that vorinostat arrests the cell cycle as well as induces apoptosis in the ovarian cancer cell lines (Takai and Narahara, 2010). Further, vorinostat was found to induce apoptosis (caspase- 3 activity) in about half the ovarian cancer cell lines along with few of the primary cancer cells, isolated from stage III EOC patients. However, vorinostat failed to show anti- tumor activity in platinum- resistant EOC cells. The fact that vorinostat enhanced apoptosis as well as reduced viability in a similar fashion as that of paclitaxel in EOC cell lines, was reported by Cooper et al. (2007), however the combination was not significant statistically. CBHA, TSA, scriptaid, vorinostat, sodium butyrate, VPA, PDX101, MS-275, M344 and apicidin, are the HDACi drugs that exhibited anticancer activity as single agents. An approach involving the combination of HDACi with other agents, namely carboplatin, paclitaxel, cisplatin, docetaxel, etc., are being studied for efficacy in ovarian cancer (Khabele, 2014). This multi- targeted approach is to treat ovarian cancer is highly beneficial as it exploits the varied mechanisms of action of HDACi, thereby producing a synergistic effect with the other agents which finally leads to an enhanced anti-tumor activity in ovarian cancer (Hontecillas-Prieto et al., 2020).
On the other hand, it has been stated that scriptaid, apicidin and CBHA enhanced the amount of cells in various phases of the cell cycle (namely the G0/G1 and/or G2/M phases) and reduced the amount of cells in S phase of the cell cycle (Tan et al., 2010). It has been indicated by various studies that apoptosis induced by HDACi is linked to the loss of mitochondrial transmembrane potential along with the altered expression of E-cadherin, p21WAF1, cyclin A, p27KIP1, and p16. It was reported that treatments with scriptaid, apicidin and CBHA enhanced the acetylation of H3 and H4 histone tails (Eckschlager et al., 2017). From these results, it has been observed that the HDACi exert anti-proliferative activity through the induction of selective genes inducing cell growth, malignant phenotype and apoptosis (Takai et al., 2007). Role of HDACi in treatment ovarian cancer is given in Figure 5.
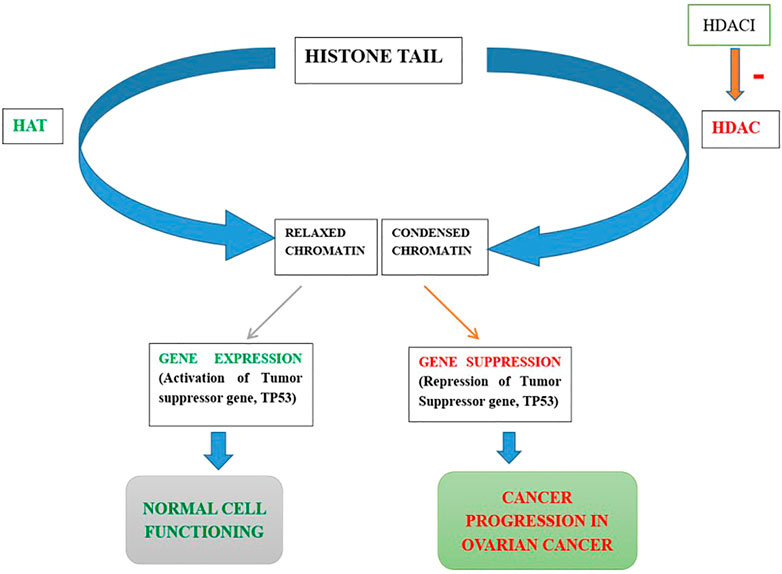
FIGURE 5. Role of HDACi in treatment ovarian cancer. HDAC, Histone deacetylase; HAT, Histone acetyltransferase; HDACi, Histone deacetylase inhibitor.
Registered HDACi in clinical trials of ovarian cancer
Romidepsin, panobinostat as well as vorinostat, are the HDACi that have been successfully tested against ovarian cancer, both individually as well as in combination with cisplatin-like drugs, along with being approved by FDA (Singh et al., 2011). Apart from these three agents, several HDACi are going through rapid development as well as being under investigation in preclinical and clinical trials for having anti-ovarian cancer potential. The impact of belinostat was evaluated in platinum-resistant ovarian cancer population in a study conducted by the Gynecologic Oncology Group (GOG) (Singh et al., 2011). This study was terminated in initial stages, due to high toxicity along with the lack of activity. However, this study was initiated again and phase Ib/II study was performed by Dizon et al. (2012), with an investigative expansion of phase 2 scheduled for women having recurrent EOC for the clinical activity evaluation of BelCaP (Belinostat, Carboplatin and Paclitaxel). It was revealed by the results that 46% of the tested population had primary platinum-resistant disease. On the other hand about 54% of the patients showed recurrence within 6 months of the treatment. BelCaP was administered for 6 (range, 1–23) median no. cycles with an overall response rate of 43% (95% confidence interval, 26%–61%). However, it was observed that median overall survival rate was not attained in the duration of the follow-up study (4 months-median follow-up). It was further revealed from the results that BelCaP was tolerated reasonably well and its clinical benefits were verified in heavily-pretreated EOC patients. The addition of belinostat to this platinum-based regimen represents a novel approach for the therapy of epithelial ovarian cancer (EOC) and required further investigation (Singh et al., 2011).
HDACi like trapoxin have poor bioavailability in-vivo along with having adverse effects at high doses and thereby have limited therapeutic use. Phase I clinical trial of phenylbutyrate sodium for dose escalation to test its efficacy in advanced-stage tumor patients was by Camacho et al. (2016) at Memorial Sloan-Kettering Cancer Center. It was observed that the administration of phenylbutyrate sodium twice a day as an infusion schedule was deemed safe, with a maximum tolerated dose of 300 mg/kg/day (Singh et al., 2011). NaB, on the other hand, had a noteworthy suppressive effect on growth in human ovarian cancer cells, regardless of their p53 gene status, as indicated by the study conducted by Terao et al. (2001), it has been studied at length for its antitumor activity, it has been found that NaB can induce cancer cell differentiation, however, its therapeutic potential has been limited by a plasma half-life of only 5 min (short t1/2). Vorinostat was subjected to a phase II clinical trial to evaluate its efficacy and toxicity in recurrent or persistent EOC patients. An oral daily dose of 400 mg of vorinostat was continued for a duration of about 3 weeks and toxic side effects barred further therapy with vorinostat (Hontecillas-Prieto et al., 2020). Another drug romidepsin, obtained from a bacterial source C. violaceum, is an HDACi and brings about apoptosis in tumor cells. It was approved by FDA in 2009, along with the clinical trials that have been executed in a variety of tumors including ovarian cancer, prostate cancer, breast cancer, etc. (Smith et al., 2017). Many of the other compounds namely abexinostat (PCI24781), givinostat (ITF2357), quisinostat (JNJ-26481585), and resminostat (4SC201) have been investigated recently as the pan-HDACi in clinical trails. However, vorinostat and romidepsin have not been exhibited in studies with various solid tumors including ovarian, cervical tumors, etc. Thus, a combination of various HDACi with other agents are under investigation in clinical trials in order to boost their anticancer potential (Khabele, 2014).
Vorinostat, in combination with gemcitabine and carboplatin along with the continuation of vorinostat, is used in the treatment of platinum-sensitive, recurrent epithelial ovarian, fallopian tube, or peritoneal cancer (Eckschlager et al., 2017). This study was terminated in the IB/II phase of clinical trials due to unacceptable toxicity. Similarly, vorinostat is used to treat primary advanced stage ovarian cancer in combination with paclitaxel and carboplatin. This study was also terminated in phase I/II of the clinical trials, due to unavoidable toxicity like GI perforation with results indicating a complete response of 39%, partial response of 11.2%, and an overall response rate of 50% in the duration of the study (Khabele, 2014).
On the other hand, belinostat (PXD101) combined with carboplatin is used to treat recurrent or persistent platinum-resistant ovarian, fallopian tube, or primary peritoneal cancer. This study was terminated in phase II due to minimal activity and the results show that there was a partial response of 3.7%, 44.4% of stable disease and 29.6% of progressive diseases (Khabele 2014). Similarly, belinostat in combination with carboplatin and paclitaxel is used to treat ovarian cancer, the study was completed in phase I/II with no observed grade 4 toxicities, the results indicated a complete response of 8.6%, 34.2% of partial response, and an overall response rate of 43% on completion of the study. A minor response was obtained in phase I of clinical trial of valproic acid, in combination with 5-azacytidine and carboplatin, in treatment of platinum resistant epithelial ovarian cancer (Smith et al., 2017). Recent HDACi in clinical trials of ovarian cancer have been shown in Table 7. Also the role of HDACi in combination with other drugs in clinical research and the status of clinical research on HDACIs have been shown in Table 8 and Table 9, respectively.
Conclusion and future direction
HDACs participates in a variety of physiological processes of cells through histone and non-histone substrates, and is closely related to the occurrence and development of cancer. HDACi can inhibit the proliferation of cancer cells and promote apoptosis, and have become an effective means of cancer treatment. Over the last decade, numerous HDACi have been discovered with five of them (Vorinostat, FK-288, PXD-101, panobinostat and CS-055) approved for clinical use as anticancer drugs. However, there are two major problems that limit the clinical uses of HDACi, which include toxicity and drug resistance. The toxicity of current pan-HDACi is largely attributed to the lack of HDACs isoforms selectivity; while the causes of drug resistance to HDACi are multi-fold, including but not limiting to the (re) activation of other signaling pathways such as CDK and AKT.
Author contributions
YD contributed in the generation of concept, literature search and revision of the manuscript. SP, AK, and UM contributed significantly in the preparation of the manuscript and the literature review. DK and MR contributed significantly in the preparation of the manuscript. All authors of this manuscript have read and approved the manuscript for submission.
Acknowledgments
The authors are thankful to the Science and Engineering Board (SERB), the Department of Science and Technology, New Delhi, India for providing necessary facilities for the completion of the work (Project File number SRG/2021/001631). The authors are also thankful to UCSI University Research Excellence and Innovation Grant (REIG) with project code REIG-FPS-2022/005 for their support in this work.
Conflict of interest
The authors declare that the research was conducted in the absence of any commercial or financial relationships that could be construed as a potential conflict of interest.
Publisher’s note
All claims expressed in this article are solely those of the authors and do not necessarily represent those of their affiliated organizations, or those of the publisher, the editors and the reviewers. Any product that may be evaluated in this article, or claim that may be made by its manufacturer, is not guaranteed or endorsed by the publisher.
References
Aggarwal, R. R., Schweizer, M. T., Nanus, D. M., Pantuck, A. J., Heath, E. I., Campeau, E., et al. (2020). A phase Ib/IIa study of the pan-BET inhibitor ZEN-3694 in combination with enzalutamide in patients with metastatic castration-resistant prostate cancer. Clin. Cancer Res. 26 (20), 5338–5347. doi:10.1158/1078-0432.ccr-20-1707
Amengual, J. E., Johannet, P., Lombardo, M., Zullo, K., Hoehn, D., Bhagat, G., et al. (2015). Dual targeting of protein degradation pathways with the selective HDAC6 inhibitor ACY-1215 and bortezomib is synergistic in lymphoma. Clin. Cancer Res. 21, 4663–4675. doi:10.1158/1078-0432.ccr-14-3068
Amoêdo, N. D., Rodrigues, M. F., Pezzuto, P., Galina, A., da Costa, R. M., de Almeida, F. C., et al. (2011). Energy metabolism in H460 lung cancer cells: Effects of histone deacetylase inhibitors. PLoS One 6 (7), e22264. doi:10.1371/journal.pone.0022264
Andrade, S. N., Evangelista, F. C. G., Seckler, D., Marques, D. R., Freitas, T. R., Nunes, R. R., et al. (2018). Synthesis, cytotoxic activity, and mode of action of new Santacruzamate A analogs. Med. Chem. Res. 27 (11-12), 2397–2413. doi:10.1007/s00044-018-2244-3
Andreu-Vieyra, C. V., and Berenson, J. R. (2014). The potential of panobinostat as a treatment option in patients with relapsed and refractory multiple myeloma. Ther. Adv. Hematol. 5 (6), 197–210. doi:10.1177/2040620714552614
Androutsopoulos, V. P., and Spandidos, D. A. (2017). Antiproliferative effects of TSA, PXD-101 and MS-275 in A2780 and MCF7 cells: Acetylated histone H4 and acetylated tubulin as markers for HDACi potency and selectivity. Oncol. Rep. 38 (6), 3412–3418. doi:10.3892/or.2017.6015
Anh, D. T., Hai, P.-T., Huy, L. D., Ngoc, H. B., Ngoc, T. T. M., Dung, D. T. M., et al. (2021). Novel 4-oxoquinazoline-based N-hydroxypropenamides as histone deacetylase inhibitors: Design, synthesis, and biological evaluation. ACS Omega 6 (7), 4907–4920. doi:10.1021/acsomega.0c05870
Audia, J. E., and Campbell, R. M. (2016). Histone modifications and cancer. Cold Spring Harb. Perspect. Biol. 8 (4), a019521. doi:10.1101/cshperspect.a019521
Bassett, S. A., and Barnett, M. P. (2014). The role of dietary histone deacetylases (HDACs) inhibitors in health and disease. Nutrients 6 (10), 4273–4301. doi:10.3390/nu6104273
Bieliauskas, A. V., and Pflum, M. K. (2008). Isoform-selective histone deacetylase inhibitors. Chem. Soc. Rev. 37 (7), 1402. doi:10.1039/b703830p
Bingul, M., Arndt, G. M., Marshall, G. M., Black, D. S., Cheung, B. B., Kumar, N., et al. (2021). Synthesis and characterisation of novel tricyclic and tetracyclic furoindoles: Biological evaluation as SAHA enhancer against neuroblastoma and breast cancer cells. Molecules 26 (19), 5745. doi:10.3390/molecules26195745
Bouras, T., Fu, M., Sauve, A. A., Wang, F., Quong, A. A., Perkins, N. D., et al. (2005). SIRT1 deacetylation and repression of p300 involves lysine residues 1020/1024 within the cell cycle regulatory domain 1. J. Biol. Chem. 280 (11), 10264–10276. doi:10.1074/jbc.m408748200
Bradbury, C. A., Khanim, F. L., Hayden, R., Bunce, C. M., White, D. A., Drayson, M. T., et al. (2005). Histone deacetylases in acute myeloid leukaemia show a distinctive pattern of expression that changes selectively in response to deacetylase inhibitors. Leukemia 19 (10), 1751–1759. doi:10.1038/sj.leu.2403910
Bradley, D., Rathkopf, D., Dunn, R., Stadler, W. M., Liu, G., Smith, D. C., et al. (2009). Vorinostat in advanced prostate cancer patients progressing on prior chemotherapy (national cancer Institute trial 6862) trial results and interleukin-6 analysis: A study by the department of defense prostate cancer clinical trial consortium and university of chicago phase 2 consortium. Cancer 115 (23), 5541–5549. doi:10.1002/cncr.24597
Brehm, A., Miska, E. A., McCance, D. J., Reid, J. L., Bannister, A. J., Kouzarides, T., et al. (1998). Retinoblastoma protein recruits histone deacetylase to repress transcription. nature 391 (6667), 597–601. doi:10.1038/35404
Buggy, J. J., Sideris, M. L., Mak, P., Lorimer, D. D., Mcintosh, B., Clark, J. M., et al. (2000). Cloning and characterization of a novel human histone deacetylase, HDAC8. Biochem. J. 350 (1), 199–205. doi:10.1042/bj3500199
Camacho, P. M., Petak, S. M., Binkley, N., Clarke, B. L., Harris, S. T., Hurley, D. L., et al. (2016). American Association Of Clinical Endocrinologists and American College of Endocrinology clinical practice guidelines for the diagnosis and treatment of postmenopausal osteoporosis-2016-executive summary. Endocr. Pract. 22 (9), 1111–1118.
Carafa, V., Nebbioso, A., and Altucci, L. (2012). Sirtuins and disease: The road ahead. Front. Pharmacol. 3, 4. doi:10.3389/fphar.2012.00004
Cava, C., Bertoli, G., and Castiglioni, I. (2015). Integrating genetics and epigenetics in breast cancer: Biological insights, experimental, computational methods and therapeutic potential. BMC Syst. Biol. 9 (1), 62. doi:10.1186/s12918-015-0211-x
Cha, E. K., and Eastham, J. A. (2015). Chemotherapy and novel therapeutics before radical prostatectomy for high-risk clinically localized prostate cancer. Urol. Oncol. 33 (No. 5), 217–225. Elsevier. https://clinicaltrials.gov/ct2/show/NCT00589472. doi:10.1016/j.urolonc.2014.11.020
Chatterjee, N., Wang, W. L., Conklin, T., Chittur, S., and Tenniswood, M. (2013). Histone deacetylase inhibitors modulate miRNA and mRNA expression, block metaphase, and induce apoptosis in inflammatory breast cancer cells. Cancer Biol. Ther. 14 (7), 658–671. doi:10.4161/cbt.25088
Chen, A. C., Martin, A. J., Choy, B., Fernández-Peñas, P., Dalziell, R. A., McKenzie, C. A., et al. (2015). A phase 3 randomized trial of nicotinamide for skin-cancer chemoprevention. N. Engl. J. Med. Overseas. Ed. 373 (17), 1618–1626. doi:10.1056/nejmoa1506197
Chen, B., Zang, W., Wang, J., Huang, Y., He, Y., Yan, L., et al. (2015). The chemical biology of sirtuins. Chem. Soc. Rev. 44 (15), 5246–5264. doi:10.1039/c4cs00373j
Chen, H., Toyooka, S., Gazdar, A. F., and Hsieh, J. T. (2003). Epigenetic regulation of a novel tumor suppressor gene (hDAB2IP) in prostate cancer cell lines. J. Biol. Chem. 278 (5), 3121–3130. doi:10.1074/jbc.m208230200
Chen, H., Tu, S. W., and Hsieh, J. T. (2005). Downregulation of human DAB2IP gene expression mediated by polycomb Ezh2 complex and histone deacetylase in prostate cancer. J. Biol. Chem. 280, 22437–22444. doi:10.1074/jbc.m501379200
Chen, J., Sang, Z., Jiang, Y., Yang, C., and He, L. (2019). Design, synthesis, and biological evaluation of quinazoline derivatives as dual HDAC1 and HDAC6 inhibitors for the treatment of cancer. Chem. Biol. Drug Des. 93 (3), 232–241. doi:10.1111/cbdd.13405
Chen, L., Jin, T., Zhu, K., Piao, Y., Quan, T., Quan, C., et al. (2017). PI3K/mTOR dual inhibitor BEZ235 and histone deacetylase inhibitor Trichostatin A synergistically exert anti-tumor activity in breast cancer. Oncotarget 8 (7), 11937–11949. doi:10.18632/oncotarget.14442
Chen, S., Ye, J., Kijima, I., and Evans, D. (2010). The HDAC inhibitor LBH589 (panobinostat) is an inhibitory modulator of aromatase gene expression. Proc. Natl. Acad. Sci. U. S. A. 107 (24), 11032–11037. doi:10.1073/pnas.1000917107
Chen, X., Zhao, S., Wu, Y., Chen, Y., Lu, T., Zhu, Y., et al. (2016). Design, synthesis and biological evaluation of 2-amino-N-(2-aminophenyl)thiazole-5-carboxamide derivatives as novel Bcr-Abl and histone deacetylase dual inhibitors. RSC Adv. 6 (105), 103178–103184. doi:10.1039/c6ra21271a
Chen, Z., Wang, X., Yang, X., Xu, Y., Yang, Y., Wang, H., et al. (2021). Imaging assisted evaluation of antitumor efficacy of a new histone deacetylase inhibitor in the castration-resistant prostate cancer. Eur. J. Nucl. Med. Mol. Imaging 48 (1), 53–66. doi:10.1007/s00259-020-04896-7
Cheng, Y., He, C., Wang, M., Ma, X., Mo, F., Yang, S., et al. (2019). Targeting epigenetic regulators for cancer therapy: Mechanisms and advances in clinical trials. Signal transduction and targeted therapy. Signal Transduct. Target Ther. 4 (1), 62. doi:10.1038/s41392-019-0095-0
Chiu, H. W., Yeh, Y. L., Wang, Y. C., Huang, W. J., Chen, Y. A., Chiou, Y. S., et al. (2013). Suberoylanilide hydroxamic acid, an inhibitor of histone deacetylase, enhances radiosensitivity and suppresses lung metastasis in breast cancer in vitro and in vivo. Plos one 8 (10), e76340. doi:10.1371/journal.pone.0076340
Chopin, V., Toillon, R. A., Jouy, N., and Bourhis, X. L. (2002). Sodium butyrate induces P53‐independent, Fas‐mediated apoptosis in MCF‐7 human breast cancer cells. Br. J. Pharmacol. 135 (1), 79–86. doi:10.1038/sj.bjp.0704456
Chopin, V., Toillon, R. A., Jouy, N., and Le Bourhis, X. (2004). P21 WAF1/CIP1 is dispensable for G1 arrest, but indispensable for apoptosis induced by sodium butyrate in MCF-7 breast cancer cells. Oncogene 23 (1), 21–29. doi:10.1038/sj.onc.1207020
Choudhary, S., Rathore, K., and Wang, H. C. (2010). FK228 and oncogenic H-Ras synergistically induce Mek1/2 and Nox-1 to generate reactive oxygen species for differential cell death. Anti-cancer drugs 21 (9), 831–840. doi:10.1097/cad.0b013e32833ddba6
Christofori, G., and Semb, H. (1999). The role of the cell-adhesion molecule E-cadherin as a tumour-suppressor gene. Trends Biochem. Sci. 24 (2), 73–76. doi:10.1016/s0968-0004(98)01343-7
Chun, P. (2015). Histone deacetylase inhibitors in hematological malignancies and solid tumors. Arch. Pharm. Res. 38 (6), 933–949. doi:10.1007/s12272-015-0571-1
Cogliati, V., Capici, S., Pepe, F. F., di Mauro, P., Riva, F., Cicchiello, F., et al. (2022). How to treat HR+/HER2-Metastatic breast cancer patients after CDK4/6 inhibitors: An unfinished story. Life 12 (3), 378. https://www.clinicaltrials.gov/ct2/show/NCT05400993. doi:10.3390/life12030378
Cohen, H. Y., Miller, C., Bitterman, K. J., Wall, N. R., Hekking, B., Kessler, B., et al. (2004). Calorie restriction promotes mammalian cell survival by inducing the SIRT1 deacetylase. science 305 (5682), 390–392. doi:10.1126/science.1099196
Connolly, R. M., Rudek, M. A., and Piekarz, R. (2017). Entinostat: A promising treatment option for patients with advanced breast cancer. Future Oncol. 13 (13), 1137–1148. doi:10.2217/fon-2016-0526
Connolly, R. M., Zhao, F., Miller, K. D., Lee, M. J., Piekarz, R. L., Smith, K. L., et al. (2021). E2112: Randomized phase III trial of endocrine therapy plus entinostat or placebo in hormone ReceptorPositive advanced breast cancer. A trial of the ECOG-ACRIN cancer research group. J. Clin. Oncol. 39, 3171–3181. doi:10.1200/jco.21.00944
Contreras-Leal, E., Hernández-Oliveras, A., Flores-Peredo, L., Zarain-Herzberg, Á., and Santiago-García, J. (2016). Histone deacetylase inhibitors promote the expression of ATP2A3 gene in breast cancer cell lines. Mol. Carcinog. 55 (10), 1477–1485. doi:10.1002/mc.22402
Cooper, A. L., Greenberg, V. L., Lancaster, P. S., van Nagell, J. R., Zimmer, S. G., Modesitt, S. C., et al. (2007). In vitro and in vivo histone deacetylase inhibitor therapy with suberoylanilide hydroxamic acid (SAHA) and paclitaxel in ovarian cancer. Gynecol. Oncol. 1043, 596–601. doi:10.1016/j.ygyno.2006.09.011
Cosenza, M., Civallero, M., Marcheselli, L., Sacchi, S., and Pozzi, S. (2017). Ricolinostat, a selective HDAC6 inhibitor, shows anti-lymphoma cell activity alone and in combination with bendamustine. Apoptosis 22, 827–840. doi:10.1007/s10495-017-1364-4
Cui, Z., Scruggs, S. B., Gilda, J. E., Ping, P., and Gomes, A. V. (2014). Regulation of cardiac proteasomes by ubiquitination, SUMOylation, and beyond. J. Mol. Cell. Cardiol. 71, 32–42. doi:10.1016/j.yjmcc.2013.10.008
Dai, C., and Gu, W. (2010). p53 post-translational modification: deregulated in tumorigenesis. Trends Mol. Med. 16 (11), 528–536. doi:10.1016/j.molmed.2010.09.002
Dai, Y., Ngo, D., Forman, L. W., Qin, D. C., Jacob, J., Faller, D. V., et al. (2007). Sirtuin 1 is required for antagonist-induced transcriptional repression of androgen-responsive genes by the androgen receptor. Mol. Endocrinol. 21 (8), 1807–1821. doi:10.1210/me.2006-0467
Damaskos, C., Valsami, S., Kontos, M., Spartalis, E., Kalampokas, T., Kalampokas, E., et al. (2017). Histone deacetylase inhibitors: An attractive therapeutic strategy against breast cancer. Anticancer Res. 37 (1), 35–46. doi:10.21873/anticanres.11286
Damodaran, S., Kyriakopoulos, C. E., and Jarrard, D. F. (2017). Newly diagnosed metastatic prostate cancer: Has the paradigm changed? Urol. Clin. North Am. 44 (4), 611–621. doi:10.1016/j.ucl.2017.07.008
Darwiche, N. (2020). Epigenetic mechanisms and the hallmarks of cancer: An intimate affair. Am. J. Cancer Res. 10 (7), 1954–1978.
De, U., Son, J. Y., Sachan, R., Park, Y. J., Kang, D., Yoon, K., et al. (2018). A new synthetic histone deacetylase inhibitor, MHY2256, induces apoptosis and autophagy cell death in endometrial cancer cells via p53 acetylation. Int. J. Mol. Sci. 19 (9), 2743. doi:10.3390/ijms19092743
Delage, B., and Dashwood, R. H. (2008). Dietary manipulation of histone structure and function. Annu. Rev. Nutr. 28, 347–366. doi:10.1146/annurev.nutr.28.061807.155354
Derr, R. S., van Hoesel, A. Q., Benard, A., Goossens-Beumer, I. J., Sajet, A., Dekker-Ensink, N. G., et al. (2014). High nuclear expression levels of histone-modifying enzymes LSD1, HDAC2 and SIRT1 in tumor cells correlate with decreased survival and increased relapse in breast cancer patients. BMC cancer 14 (1), 604. doi:10.1186/1471-2407-14-604
DeSantis, C. E., Bray, F., Ferlay, J., Lortet-Tieulent, J., Anderson, B. O., Jemal, A., et al. (2015). International variation in female breast cancer incidence and mortality rates. Cancer Epidemiol. Biomarkers Prev. 24 (10), 1495–1506. doi:10.1158/1055-9965.epi-15-0535
Dizon, D. S., Blessing, J. A., Penson, R. T., Drake, R. D., Walker, J. L., Johnston, C. M., et al. (2012). A phase II evaluation of belinostat and carboplatin in the treatment of recurrent or persistent platinum-resistant ovarian, fallopian tube, or primary peritoneal carcinoma: A gynecologic oncology group study. Gynecol. Oncol. 125 (2), 367–371. doi:10.1016/j.ygyno.2012.02.019
Donohoe, D. R., Collins, L. B., Wali, A., Bigler, R., Sun, W., Bultman, S. J., et al. (2012). The Warburg effect dictates the mechanism of butyrate-mediated histone acetylation and cell proliferation. Mol. Cell. 48 (4), 612–626. doi:10.1016/j.molcel.2012.08.033
Donohoe, D. R., Holley, D., Collins, L. B., Montgomery, S. A., Whitmore, A. C., Hillhouse, A., et al. (2014). A gnotobiotic mouse model demonstrates that dietary fiber protects against colorectal tumorigenesis in a microbiota-and butyrate-dependent manner. Cancer Discov. 4 (12), 1387–1397. doi:10.1158/2159-8290.cd-14-0501
Eckschlager, Tomas, Plch, Johana, Stiborova, Marie, and Jan, Hrabeta (2017). Histone deacetylase inhibitors as anticancer drugs. Int. J. Mol. Sci. 18 (7), 1414. doi:10.3390/ijms18071414
Eckstein, N. (2011). Platinum resistance in breast and ovarian cancer cell lines. J. Exp. Clin. Cancer Res. 30 (1), 91. doi:10.1186/1756-9966-30-91
Eigl, B. J., North, S., Winquist, E., Finch, D., Wood, L., Sridhar, S. S., et al. (2015). A phase II study of the HDAC inhibitor SB939 in patients with castration resistant prostate cancer: NCIC clinical trials group study IND195. Invest. New Drugs 33 (4), 969–976. https://clinicaltrials.gov/ct2/show/NCT01075308. doi:10.1007/s10637-015-0252-4
Emiliani, S., Fischle, W., Van Lint, C., Al-Abed, Y., and Verdin, E. (1998). Characterization of a human RPD3 ortholog, HDAC3. Proc. Natl. Acad. Sci. U. S. A. 95 (6), 2795–2800. doi:10.1073/pnas.95.6.2795
Falahi, F., van Kruchten, M., Martinet, N., Hospers, G., and Rots, M. G. (2014). Current and upcoming approaches to exploit the reversibility of epigenetic mutations in breast cancer. Breast Cancer Res. 16 (4), 412. doi:10.1186/s13058-014-0412-z
Falchook, Gerald S., Fu, Siqing, Aung, Naing, Hong, David S., Hu, Wei, Moulder, Stacy, et al. (2013). Methylation and histone deacetylase inhibition in combination with platinum treatment in patients with advanced malignancies. Invest. New Drugs 31 (5), 1192–1200. doi:10.1007/s10637-013-0003-3
Farrow, B., Rychahou, P., O’Connor, K. L., and Evers, B. M. (2003). Butyrate inhibits pancreatic cancer invasion. J. Gastrointest. Surg. 7 (7), 864–870. doi:10.1007/s11605-003-0031-y
J. Ferlay, M. Ervik, F. Lam, M. Colombet, L. Mery, M. Piñeroset al. (Eds.) (2020). Global Cancer Observatory: Cancer Today. International Agency for Research on Cancer. Available at: gco.iarc.fr/today (Accessed November 25, 2020).
Ferrari, A. C., Alumkal, J. J., Stein, M. N., Taplin, M. E., Babb, J., Barnett, E. S., et al. (2019). Epigenetic therapy with panobinostat combined with bicalutamide rechallenge in castration-resistant prostate cancer. Clin. Cancer Res. 25 (1), 52–63. doi:10.1158/1078-0432.ccr-18-1589
Fischer, D. D., Cai, R., Bhatia, U., Asselbergs, F. A., Song, C., Terry, R., et al. (2002). Isolation and characterization of a novel class II histone deacetylase, HDAC10. J. Biol. Chem. 277 (8), 6656–6666. doi:10.1074/jbc.m108055200
Fiskus, W., Ren, Y., Mohapatra, A., Bali, P., Mandawat, A., Rao, R., et al. (2007). Hydroxamic acid analogue histone deacetylase inhibitors attenuate estrogen receptor-α levels and transcriptional activity: A result of hyperacetylation and inhibition of chaperone function of heat shock protein 90. Clin. Cancer Res. 13 (16), 4882–4890. doi:10.1158/1078-0432.ccr-06-3093
Fitzmaurice, C., Allen, C., Barber, R. M., Barregard, L., Bhutta, Z. A., Brenner, H., et al. (2017). Global, regional, and national cancer incidence, mortality, years of life lost, years lived with disability, and disability-adjusted life-years for 32 cancer groups, 1990 to 2015: A systematic analysis for the global burden of disease study. JAMA Oncol. 3 (4), 524–548. doi:10.1001/jamaoncol.2016.5688
Fraga, M. F., Ballestar, E., Villar-Garea, A., Boix-Chornet, M., Espada, J., Schotta, G., et al. (2005). Loss of acetylation at Lys16 and trimethylation at Lys20 of histone H4 is a common hallmark of human cancer. Nat. Genet. 37 (4), 391–400. doi:10.1038/ng1531
Fragomeni, S. M., Sciallis, A., and Jeruss, J. S. (2018). Molecular subtypes and local-regional control of breast cancer. Surg. Oncol. Clin. N. Am. 27 (1), 95–120. doi:10.1016/j.soc.2017.08.005
Fu, M., Liu, M., Sauve, A. A., Jiao, X., Zhang, X., Wu, X., et al. (2006). Hormonal control of androgen receptor function through SIRT1. Mol. Cell. Biol. 26 (21), 8122–8135. doi:10.1128/mcb.00289-06
Fu, M., Rao, M., Wang, C., Sakamaki, T., Wang, J., Di Vizio, D., et al. (2003). Acetylation of androgen receptor enhances coactivator binding and promotes prostate cancer cell growth. Mol. Cell. Biol. 23 (23), 8563–8575. doi:10.1128/mcb.23.23.8563-8575.2003
Gao, L., Cueto, M. A., Asselbergs, F., and Atadja, P. (2002). Cloning and functional characterization of HDAC11, a novel member of the human histone deacetylase family. J. Biol. Chem. 277 (28), 25748–25755. doi:10.1074/jbc.m111871200
García, S., Mercado-Sánchez, I., Bahena, L., Alcaraz, Y., García-Revilla, M. A., Robles, J., et al. (2020). Design of fluorescent coumarin-hydroxamic acid derivatives as inhibitors of HDACs: Synthesis, anti-proliferative evaluation and docking studies. Molecules 25 (21), 5134. doi:10.3390/molecules25215134
Gass-Jégu, F., Gschwend, A., Gairard-Dory, A. C., Mennecier, B., Tebacher-Alt, M., Gourieux, B., et al. (2016). Gastrointestinal perforations in patients treated with erlotinib: A report of two cases with fatal outcome and literature review. Lung Cancer 99, 76–78. doi:10.1016/j.lungcan.2016.06.012
Gerber, D. E., Boothman, D. A., Fattah, F. J., Dong, Y., Zhu, H., Skelton, R. A., et al. (2015). Phase 1 study of romidepsin plus erlotinib in advanced non- small cell lung cancer. Lung Cancer 90, 534–541. doi:10.1016/j.lungcan.2015.10.008
Ginsburg, E., Salomon, D., Sreevalsan, T., and Freese, E. (1973). Growth inhibition and morphological changes caused by lipophilic acids in mammalian cells. Proc. Natl. Acad. Sci. U. S. A. 70 (8), 2457–2461. doi:10.1073/pnas.70.8.2457
Giuliano, M., Schiff, R., Osborne, C. K., and Trivedi, M. V. (2011). Biological mechanisms and clinical implications of endocrine resistance in breast cancer. Breast 20, S42–S49. doi:10.1016/s0960-9776(11)70293-4
Glaser, K. B., Staver, M. J., Waring, J. F., Stender, J., Ulrich, R. G., Davidsen, S. K., et al. (2003). Gene expression profiling of multiple histone deacetylase (HDAC) inhibitors: Defining a common gene set produced by HDAC inhibition in T24 and MDA carcinoma cell lines. Mol. Cancer Ther. 2 (2), 151–163.
Goehringer, N., Biersack, B., Peng, Y., Schobert, R., Herling, M., Ma, A., et al. (2021). Anticancer activity and mechanisms of action of new chimeric EGFR/HDAC-inhibitors. Int. J. Mol. Sci. 22 (16), 8432. doi:10.3390/ijms22168432
Gong, P., Wang, Y., and Jing, Y. (2019). Apoptosis induction byhistone deacetylase inhibitors in cancer cells: Role of Ku70. Int. J. Mol. Sci. 20 (7), 1601. doi:10.3390/ijms20071601
Gradilone, S. A., Radtke, B. N., Bogert, P. S., Huang, B. Q., Gajdos, G. B., LaRusso, N. F., et al. (2013). HDAC6 inhibition restores ciliary expression and decreases tumor growth. Cancer Res. 73 (7), 2259–2270. doi:10.1158/0008-5472.can-12-2938
Gromek, S. M., deMayo, J. A., Maxwell, A. T., West, A. M., Pavlik, C. M., Zhao, Z., et al. (2016). Synthesis and biological evaluation of santacruzamate A analogues for anti-proliferative and immunomodulatory activity. Bioorg. Med. Chem. 24 (21), 5183–5196. doi:10.1016/j.bmc.2016.08.040
Guardiola, A. R., and Yao, T. P. (2002). Molecular cloning and characterization of a novel histone deacetylase HDAC10. J. Biol. Chem. 277 (5), 3350–3356. doi:10.1074/jbc.m109861200
Guerriero, J. L., Sotayo, A., Ponichtera, H. E., Castrillon, J. A., Pourzia, A. L., Schad, S., et al. (2017). Class IIa HDAC inhibition reduces breast tumours and metastases through anti-tumour macrophages. Nature 543 (7645), 428–432. doi:10.1038/nature21409
Haigis, M. C., and Sinclair, D. A. (2010). Mammalian sirtuins: Biological insights and disease relevance. Annu. Rev. Pathol. Mech. Dis. 5, 253–295. doi:10.1146/annurev.pathol.4.110807.092250
Hait, N. C., Allegood, J., Maceyka, M., Strub, G. M., Harikumar, K. B., Singh, S. K., et al. (2009). Regulation of histone acetylation in the nucleus by sphingosine-1-phosphate. Science 325 (5945), 1254–1257. doi:10.1126/science.1176709
Hajra, K. M., and Fearon, E. R. (2002). Cadherin and catenin alterations in human cancer. Genes. Chromosom. Cancer 34 (3), 255–268. doi:10.1002/gcc.10083
Halkidou, K., Cook, S., Leung, H. Y., Neal, D. E., and Robson, C. N. (2004). Nuclear accumulation of histone deacetylase 4 (HDAC4) coincides with the loss of androgen sensitivity in hormone refractory cancer of the prostate. Eur. Urol. 45 (3), 382–389. doi:10.1016/j.eururo.2003.10.005
Hao, Y., Wang, G., Lin, C., Li, D., Ji, Z., Gao, F., et al. (2017). Valproic acid induces decreased expression of H19 promoting cell apoptosis in A549 cells. DNA Cell. Biol. 36 (6), 428–435. doi:10.1089/dna.2016.3542
Hayden, A. J., Catton, C., and Pickles, T. (2010). Radiation therapy in prostate cancer: A risk-adapted strategy. Curr. Oncol. 17 (s2), 18–24. doi:10.3747/co.v17i0.704
Hellerstedt, B. A., and Pienta, K. J. (2002). The current state of hormonal therapy for prostate cancer. CA a cancer J. Clin. 52 (3), 154–179. doi:10.3322/canjclin.52.3.154
Hervouet, E., Claude-Taupin, A., Gauthier, T., Perez, V., Fraichard, A., Adami, P., et al. (2015). The autophagy GABARAPL1 gene is epigenetically regulated in breast cancer models. BMC cancer 15 (1), 729. doi:10.1186/s12885-015-1761-4
Hieu, D. T., Anh, D. T., Tuan, N. M., Hai, P.-T., Huong, L.-T.-T., Kim, J., et al. (2018). Design, synthesis and evaluation of novel N -hydroxybenzamides/N -hydroxypropenamides incorporating quinazolin-4(3 H )-ones as histone deacetylase inhibitors and antitumor agents. Bioorg. Chem. 76, 258–267. doi:10.1016/j.bioorg.2017.12.007
Holkova, B., Kmieciak, M., Bose, P., Yazbeck, V. Y., Barr, P. M., Tombes, M. B., et al. (2016). Phase 1 trial of carfilzomib (PR-171) in combination with vorinostat (SAHA) in patients with relapsed or refractory B-cell lymphomas. Leuk. Lymphoma 57, 635–643. doi:10.3109/10428194.2015.1075019
Hontecillas-Prieto, L., Flores-Campos, R., Silver, A., de Álava, E., Hajji, N., and García-Domínguez, D. J. (2020). Synergistic enhancement of cancer therapy using HDAC inhibitors: Opportunity for clinical trials. Front. Genet. 11 (September). doi:10.3389/fgene.2020.578011
Hsu, K. W., Huang, C. Y., Tam, K. W., Lin, C. Y., Huang, L. C., Lin, C. L., et al. (2018). The application of non-invasive apoptosis detection sensor (NIADS) on histone deacetylation inhibitor (HDACi)-induced breast cancer cell death. Int. J. Mol. Sci. 19 (2), 452. doi:10.3390/ijms19020452
Hu, C., Xia, H., Bai, S., Zhao, J., Edwards, H., Li, X., et al. (2020). CUDC‐907, a novel dual PI3K and HDAC inhibitor, in prostate cancer: Antitumour activity and molecular mechanism of action. J. Cell. Mol. Med. 24 (13), 7239–7253. doi:10.1111/jcmm.15281
Hu, E., Chen, Z., Fredrickson, T., Zhu, Y., Kirkpatrick, R., Zhang, G. F., et al. (2000). Cloning and characterization of a novel human class I histone deacetylase that functions as a transcription repressor. J. Biol. Chem. 275 (20), 15254–15264. doi:10.1074/jbc.m908988199
Hu, W. Y., Xu, L., Chen, B., Ou, S., Muzzarelli, K. M., Hu, D. P., et al. (2019). Targeting prostate cancer cells with enzalutamide‐HDAC inhibitor hybrid drug 2‐75. Prostate 79 (10), 1166–1179. doi:10.1002/pros.23832
Huan, L. C., Tran, P.-T., Phuong, C. V., Duc, P. H., Anh, D. T., Hai, P. T., et al. (2019). Novel 3, 4-dihydro-4-oxoquinazoline-based acetohydrazides: Design, synthesis and evaluation of antitumor cytotoxicity and caspase activation activity. Bioorg. Chem. 92, 103202. doi:10.1016/j.bioorg.2019.103202
Huang, F. I., Wu, Y. W., Sung, T. Y., Liou, J. P., Lin, M. H., Pan, S. L., et al. (2019). MPT0G413, a novel HDAC6-selective inhibitor, and bortezomib synergistically exert anti-tumor activity in multiple myeloma cells. Front. Oncol. 9, 249. doi:10.3389/fonc.2019.00249
Huang, H., Liu, N., Guo, H., Liao, S., Li, X., Yang, C., et al. (2012). L-carnitine is an endogenous HDAC inhibitor selectively inhibiting cancer cell growth in vivo and in vitro. PloS one 7 (11), e49062. doi:10.1371/journal.pone.0049062
Hubbert, C., Guardiola, A., Shao, R., Kawaguchi, Y., Ito, A., Nixon, A., et al. (2002). HDAC6 is a microtubule-associated deacetylase. Nature 417 (6887), 455–458. doi:10.1038/417455a
Ibrahim, T. S., Sheha, T. A., Abo-Dya, N. E., AlAwadh, M. A., Alhakamy, N. A., Abdel-Samii, Z. K., et al. (2020). Design, synthesis and anticancer activity of novel valproic acid conjugates with improved histone deacetylase (HDAC) inhibitory activity. Bioorg. Chem. 99, 103797. doi:10.1016/j.bioorg.2020.103797
Igase, M., Fujiki, N., Shibutani, S., Sakai, H., Noguchi, S., Nemoto, Y., et al. (2020). Tenovin-6 induces the SIRT-independent cell growth suppression and blocks autophagy flux in canine hemangiosarcoma cell lines. Exp. Cell. Res. 388 (1), 111810. doi:10.1016/j.yexcr.2019.111810
Imai, S. i., and Guarente, L. (2014). NAD+ and sirtuins in aging and disease. Trends Cell. Biol. 24, 464–471. doi:10.1016/j.tcb.2014.04.002
Isaacs, J. T. (1994). Role of androgens in prostatic cancer. Vitam. Horm. 49, 433–502. doi:10.1016/s0083-6729(08)61152-8
Itazaki, H., Nagashima, K., Sugita, K., Yoshida, H., Kawamura, Y., Yasuda, Y., et al. (1990). Isolation and structural elucidation of new cyclotetrapeptides, trapoxins A and B, having detransformation activities as antitumor agents. J. Antibiot. (Tokyo). 43 (12), 1524–1532. doi:10.7164/antibiotics.43.1524
Jadhavar, P. S., Ramachandran, S. A., Riquelme, E., Gupta, A., Quinn, K. P., Shivakumar, D., et al. (2016). Targeting prostate cancer with compounds possessing dual activity as androgen receptor antagonists and HDAC6 inhibitors. Bioorg. Med. Chem. Lett. 26 (21), 5222–5228. doi:10.1016/j.bmcl.2016.09.058
Jang, E. R., Lim, S. J., Lee, E. S., Jeong, G., Kim, T. Y., Bang, Y. J., et al. (2004). The histone deacetylase inhibitor trichostatin A sensitizes estrogen receptor α-negative breast cancer cells to tamoxifen. Oncogene 23 (9), 1724–1736. doi:10.1038/sj.onc.1207315
Jeong, B. C., Hong, C. Y., Chattopadhyay, S., Park, J. H., Gong, E. Y., Kim, H. J., et al. (2004). Androgen receptor corepressor-19 kDa (ARR19), a leucine-rich protein that represses the transcriptional activity of androgen receptor through recruitment of histone deacetylase. Mol. Endocrinol. 18 (1), 13–25. doi:10.1210/me.2003-0065
Jiang, Z., Li, W., Hu, X., Zhang, Q., Sun, T., Cui, S., et al. (2019). Tucidinostat plus exemestane for postmenopausal patients with advanced, hormone receptor-positive breast cancer (ACE): A randomised, double-blind, placebo-controlled, phase 3 trial. Lancet Oncol. 20 (6), 806–815. doi:10.1016/s1470-2045(19)30164-0
Johnstone, R. W., and Licht, J. D. (2003). Histone deacetylase inhibitors in cancer therapy: Is transcription the primary target? Cancer Cell. 4 (1), 13–18. doi:10.1016/s1535-6108(03)00165-x
Juan, L. J., Shia, W. J., Chen, M. H., Yang, W. M., Seto, E., Lin, Y. S., et al. (2000). Histone deacetylases specifically down-regulate p53-dependent gene activation. J. Biol. Chem. 275 (27), 20436–20443. doi:10.1074/jbc.m000202200
Jung, K. H., Noh, J. H., Kim, J. K., Eun, J. W., Bae, H. J., Xie, H. J., et al. (2012). HDAC2 overexpression confers oncogenic potential to human lung cancer cells by deregulating expression of apoptosis and cell cycle proteins. J. Cell. Biochem. 113 (6), 2167–2177. doi:10.1002/jcb.24090
Kao, H. Y., Downes, M., Ordentlich, P., and Evans, R. M. (2000). Isolation of a novel histone deacetylase reveals that class I and class II deacetylases promote SMRT-mediated repression. Genes. Dev. 14 (1), 55–66. doi:10.1101/gad.14.1.55
Kao, H. Y., Lee, C. H., Komarov, A., Han, C. C., and Evans, R. M. (2002). Isolation and characterization of mammalian HDAC10, a novel histone deacetylase. J. Biol. Chem. 277 (1), 187–193. doi:10.1074/jbc.m108931200
Karsli-Ceppioglu, S., Dagdemir, A., Judes, G., Ngollo, M., Penault-Llorca, F., Pajon, A., et al. (2014). Epigenetic mechanisms of breast cancer: An update of the current knowledge. Epigenomics 6 (6), 651–664. doi:10.2217/epi.14.59
Kaufman, J. L., Mina, R., Jakubowiak, A. J., Zimmerman, T. L., Wolf, J. J., Lewis, C., et al. (2019). Combining carfilzomib and panobinostat to treat relapsed/refractory multiple myeloma: Results of a multiple myeloma research consortium phase I studyfilzomib and panobinostat to treat relapsed/refractory multiple myeloma: Results of a multiple myeloma research consortiumphaseIstudy. Blood Cancer J. 9, 3. doi:10.1038/s41408-018-0154-8
Kaushik, D., Vashistha, V., Isharwal, S., Sediqe, S. A., and Lin, M. F. (2015). Histone deacetylase inhibitors in castration-resistant prostate cancer: Molecular mechanism of action and recent clinical trials. Ther. Adv. Urology 7 (6), 388–395. doi:10.1177/1756287215597637
Khabele, Dineo (2014). The therapeutic potential of class I selective histone deacetylase inhibitors in ovarian cancer. Front. Oncol. 4 (May), 111. doi:10.3389/fonc.2014.00111
Kida, Y., and Goligorsky, M. S. (2016). Sirtuins, cell senescence, and vascular aging. Can. J. Cardiol. 32 (5), 634–641. doi:10.1016/j.cjca.2015.11.022
Kim, J. K., Noh, J. H., Eun, J. W., Jung, K. H., Bae, H. J., Shen, Q., et al. (2013). Targeted inactivation of HDAC2 restores p16INK4a activity and exerts antitumor effects on human gastric cancer. Mol. Cancer Res. 11 (1), 62–73. doi:10.1158/1541-7786.mcr-12-0332
Kim, Y. B., Ki, S. W., Yosnida, M., and Horinouchi, S. (2000). Mechanism of cell cycle arrest caused by histone deacetylase inhibitors in human carcinoma cells. J. Antibiot. (Tokyo). 53 (10), 1191–1200. doi:10.7164/antibiotics.53.1191
Knipstein, J., and Gore, L. (2011). Entinostat for treatment of solid tumors and hematologic malignancies. Expert Opin. investigational drugs 20 (10), 1455–1467. doi:10.1517/13543784.2011.613822
Kobayashi, T., Nakazono, K., Tokuda, M., Mashima, Y., Dynlacht, B. D., Itoh, H., et al. (2017). HDAC 2 promotes loss of primary cilia in pancreatic ductal adenocarcinoma. EMBO Rep. 18 (2), 334–343. doi:10.15252/embr.201541922
Koboldt, D. C., Fulton, R., McLellan, M., Schmidt, H., Kalicki-Veizer, J., McMichael, J., et al. (2012). Comprehensive molecular portraits of human breast tumors. Nature 490 (7418), 61–70. doi:10.1038/nature11412
Kochan, D. Z., and Kovalchuk, O. (2015). Circadian disruption and breast cancer: An epigenetic link? Oncotarget 6 (19), 16866–16882. doi:10.18632/oncotarget.4343
Koeneke, E., Witt, O., and Oehme, I. (2015). HDAC family members intertwined in the regulation of autophagy: A druggable vulnerability in aggressive tumor entities. Cells 4 (2), 135–168. doi:10.3390/cells4020135
Krusche, C. A., Wülfing, P., Kersting, C., Vloet, A., Böcker, W., Kiesel, L., et al. (2005). Histone deacetylase-1 and-3 protein expression in human breast cancer: A tissue microarray analysis. Breast Cancer Res. Treat. 90 (1), 15–23. doi:10.1007/s10549-004-1668-2
Kulp, S. K., Chen, C. S., Wang, D. S., Chen, C. Y., and Chen, C. S. (2006). Antitumor effects of a novel phenylbutyrate-based histone deacetylase inhibitor, (S)-HDAC-42, in prostate cancer. Clin. Cancer Res. 12 (17), 5199–5206. doi:10.1158/1078-0432.ccr-06-0429
Kumar, V., Akhtar, N., and Gupta, S. (2017). Neoadjuvant chemotherapy in epithelial ovarian cancer: An institutional experience. J. Cancer Res. Ther. 13.
Kuzmichev, A., Margueron, R., Vaquero, A., Preissner, T. S., Scher, M., Kirmizis, A., et al. (2005). Composition and histone substrates of polycomb repressive group complexes change during cellular differentiation. Proc. Natl. Acad. Sci. U. S. A. 102 (6), 1859–1864. doi:10.1073/pnas.0409875102
Lahm, A., Paolini, C., Pallaoro, M., Nardi, M. C., Jones, P., Neddermann, P., et al. (2007). Unraveling the hidden catalytic activity of vertebrate class IIa histone deacetylases. Proc. Natl. Acad. Sci. U. S. A. 104 (44), 17335–17340. doi:10.1073/pnas.0706487104
Lai, C. J., Bao, R., Tao, X. U., Wang, J., Atoyan, R., Qu, H., et al. (2010). CUDC-101, a multitargeted inhibitor of histone deacetylase, epidermal growth factor receptor, and human epidermal growth factor receptor 2, exerts potent anticancer activity. Cancer Res. 70 (9), 3647–3656. doi:10.1158/0008-5472.can-09-3360
Lam, H. C., Cloonan, S. M., Bhashyam, A. R., Haspel, J. A., Singh, A., Sathirapongsasuti, J. F., et al. (2013). Histone deacetylase 6–mediated selective autophagy regulates COPD-associated cilia dysfunction. J. Clin. Invest. 123 (12), 5212–5230. doi:10.1172/jci69636
Lee, B. B., Kim, Y., Kim, D., Cho, E. Y., Han, J., Kim, H. K., et al. (2019). Metformin and tenovin-6 synergistically induces apoptosis through LKB1-independent SIRT1 down-regulation in non-small cell lung cancer cells. J. Cell. Mol. Med. 23, 2872–2889. doi:10.1111/jcmm.14194
Lee, C. H., Akin-Olugbade, O., and Kirschenbaum, A. (2011). Overview of prostate anatomy, histology, and pathology. Endocrinol. Metab. Clin. North Am. 40 (3), 565–575. doi:10.1016/j.ecl.2011.05.012
Lee, J. T., and Gu, W. (2013). SIRT1: Regulator of p53 deacetylation. Genes. & cancer 4 (3-4), 112–117. doi:10.1177/1947601913484496
Lee, Y. J., Won, A. J., Lee, J., Jung, J. H., Yoon, S., Lee, B. M., et al. (2012). Molecular mechanism of SAHA on regulation of autophagic cell death in tamoxifen-resistant MCF-7 breast cancer cells. Int. J. Med. Sci. 9 (10), 881–893. doi:10.7150/ijms.5011
Lee, Y. S., Lim, K. H., Guo, X., Kawaguchi, Y., Gao, Y., Barrientos, T., et al. (2008). The cytoplasmic deacetylase HDAC6 is required for efficient oncogenic tumorigenesis. Cancer Res. 68 (18), 7561–7569. doi:10.1158/0008-5472.can-08-0188
Li, H., Tian, Z., Qu, Y., Yang, Q., Guan, H., Shi, B., et al. (2019). SIRT7 promotes thyroid tumorigenesis through phosphorylation and activation of Akt and p70S6K1 via DBC1/SIRT1 axis. Oncogene 38 (3), 345–359. doi:10.1038/s41388-018-0434-6
Li, X., Kamenecka, T. M., and Cameron, M. D. (2010). Cytochrome P450-mediated bioactivation of the epidermal growth factor receptor inhibitor erlotinib to a reactive electrophile. Drug Metab. Dispos. 38 (7), 1238–1245. doi:10.1124/dmd.109.030361
Li, X., Yin, S., Meng, Y., Sakr, W., and Sheng, S. (2006). Endogenous inhibition of histone deacetylase 1 by tumor-suppressive maspin. Cancer Res. 66 (18), 9323–9329. doi:10.1158/0008-5472.can-06-1578
Li, Y., and Seto, E. (2016). HDACs and HDAC inhibitors in cancer development and therapy. Cold Spring Harb. Perspect. Med. 6, a026831. doi:10.1101/cshperspect.a026831
Li, Z., and Zhu, W. G. (2014). Targeting histone deacetylases for cancer therapy: From molecular mechanisms to clinical implications. Int. J. Biol. Sci. 10 (7), 757–770. doi:10.7150/ijbs.9067
Lin, J., Elkon, J., Ricart, B., Palmer, E., Zevallos-Delgado, C., Noonepalle, S., et al. (2021). Phase I study of entinostat in combination with enzalutamide for treatment of patients with metastatic castration-resistant prostate cancer. Oncol. 26 (12), e2136–42. https://clinicaltrials.gov/ct2/show/NCT03829930. doi:10.1002/onco.13957
Lin, R. J., Sternsdorf, T., Tini, M., and Evans, R. M. (2001). Transcriptional regulation in acute promyelocytic leukemia. Oncogene 20 (49), 7204–7215. doi:10.1038/sj.onc.1204853
Lin, S. J., Defossez, P. A., and Guarente, L. (2000). Requirement of NAD and SIR2 for life-span extension by calorie restriction in Saccharomyces cerevisiae. Science 289 (5487), 2126–2128. doi:10.1126/science.289.5487.2126
Losson, H., Schnekenburger, M., Dicato, M., and Diederich, M. (2016). Natural compound histone deacetylase inhibitors (HDACi): Synergy with inflammatory signaling pathway modulators and clinical applications in cancer. Molecules 21 (11), 1608. doi:10.3390/molecules21111608
Louis, M., Rosato, R. R., Brault, L., Osbild, S., Battaglia, E., Yang, X. H., et al. (2004). The histone deacetylase inhibitor sodium butyrate induces breast cancer cell apoptosis through diverse cytotoxic actions including glutathione depletion and oxidative stress. Int. J. Oncol. 25 (6), 1701–1711. doi:10.3892/ijo.25.6.1701
Luchenko, V. L., Litman, T., Chakraborty, A. R., Heffner, A., Devor, C., Wilkerson, J., et al. (2014). Histone deacetylase inhibitor-mediated cell death is distinct from its global effect on chromatin. Mol. Oncol. 8 (8), 1379–1392. doi:10.1016/j.molonc.2014.05.001
Luo, J., Su, F., Chen, D., Shiloh, A., and Gu, W. (2000). Deacetylation of p53 modulates its effect on cell growth and apoptosis. Nature 408 (6810), 377–381. doi:10.1038/35042612
Marijon, H., Lee, D. H., Ding, L., Sun, H., Gery, S., de Gramont, A., et al. (2018). Co-targeting poly (ADP-ribose) polymerase (PARP) and histone deacetylase (HDAC) in triple-negative breast cancer: Higher synergism in BRCA mutated cells. Biomed. Pharmacother. 99, 543–551. doi:10.1016/j.biopha.2018.01.045
Matulonis, U., Berlin, S., Lee, H., Whalen, C., Obermayer, E., Penson, R., et al. (2015). Phase I study of combination of vorinostat, carboplatin, and gemcitabine in women with recurrent, platinum-sensitive epithelial ovarian, fallopian tube, or peritoneal cancer. Cancer Chemother. Pharmacol. 76 (2), 417–423.
Mendivil, A. A., Micha, J. P., Brown, J. V., Rettenmaier, M. A., Abaid, L. N., Lopez, K. L., et al. (2013). Increased incidence of severe gastrointestinal events with first-line paclitaxel, carboplatin, and vorinostat chemotherapy for advanced-stage epithelial ovarian, primary peritoneal, and fallopian tube cancer. Int. J. Gynecol. Cancer 23 (3).
Meng, Y., Jin, J., Gong, C., Miao, H., Tao, Z., Li, T., et al. (2021). Phase II study of chidamide in combination with cisplatin in patients with metastatic triple-negative breast cancer. Ann. Palliat. Med. 8, 11255–11264. doi:10.21037/apm-21-1139
Messier, T. L., Gordon, J. A., Boyd, J. R., Tye, C. E., Browne, G., Stein, J. L., et al. (2016). Histone H3 lysine 4 acetylation and methylation dynamics define breast cancer subtypes. Oncotarget 7 (5), 5094–5109. doi:10.18632/oncotarget.6922
Millard, T. A., Wages, N. A., Petroni, G. R., Brenin, C. M., and Dillon, P. M. (2019). “A pilot study of the combination of entinostat with capecitabine in metastatic and high risk breast cancer after neoadjuvant therapy,” in Cancer research (615 Chestnut ST, 17th Floor, Philadelphia, PA 19106-4404 USA: Amer Assoc Cancer Research), 79. https://clinicaltrials.gov/ct2/show/NCT03473639.4
Miller, E. T., Chamie, K., Kwan, L., Lewis, M. S., Knudsen, B. S., Garraway, I. P., et al. (2017). Impact of treatment on progression to castration‐resistance, metastases, and death in men with localized high‐grade prostate cancer. Cancer Med. 6 (1), 163–172. doi:10.1002/cam4.981
Mishima, Y., Santo, L., Eda, H., Cirstea, D., Nemani, N., Yee, A. J., et al. (2015). Ricolinostat (ACY-1215) induced inhibition ofaggresome formation accelerates carfilzomib-induced multiple myeloma cell death. Br. J. Haematol. 169, 423–434. doi:10.1111/bjh.13315
Miska, E. A., Karlsson, C., Langley, E., Nielsen, S. J., Pines, J., and Kouzarides, T. (1999). HDAC4 deacetylase associates with and represses the MEF2 transcription factor. EMBO J. 18 (18), 5099–5107. doi:10.1093/emboj/18.18.5099
Mita, A., Loeffler, M., Bui, N., Mehrling, T., Rimmel, B. J., Natale, R. B., et al. (2019). Abstract CT023: Dose escalation of tinostamustine in patients with advanced solid tumors. Cancer Res. 79 (13_Suppl. ment), CT023. https://clinicaltrials.gov/ct2/show/NCT03345485. doi:10.1158/1538-7445.am2019-ct023
Molife, L. R., Attard, G., Fong, P. C., Karavasilis, V., Reid, A. H., Patterson, S., et al. (2010). Phase II, two-stage, single-arm trial of the histone deacetylase inhibitor (HDACi) romidepsin in metastatic castration-resistant prostate cancer (CRPC). Ann. Oncol. 21 (1), 109–113. doi:10.1093/annonc/mdp270
Montgomery, R. B., Mostaghel, E. A., Vessella, R., Hess, D. L., Kalhorn, T. F., Higano, C. S., et al. (2008). Maintenance of intratumoral androgens in metastatic prostate cancer: A mechanism for castration-resistant tumor growth. Cancer Res. 68 (11), 4447–4454. doi:10.1158/0008-5472.can-08-0249
Montironi, R., Pomante, R., Diamanti, L., and Magi-Galluzzi, C. (1998). Apoptosis in prostatic adenocarcinoma following complete androgen ablation. Urol. Int. 60 (Suppl. 1), 25–30. doi:10.1159/000056542
Moreira, D. M., Howard, L. E., Sourbeer, K. N., Amarasekara, H. S., Chow, L. C., Cockrell, D. C., et al. (2017). Predicting time from metastasis to overall survival in castration-resistant prostate cancer: Results from SEARCH. Clin. Genitourin. cancer 15 (1), 60–66.e2. doi:10.1016/j.clgc.2016.08.018
Morrison, D. J., and Preston, T. (2016). Formation of short chain fatty acids by the gut microbiota and their impact on human metabolism. Gut microbes 7 (3), 189–200. doi:10.1080/19490976.2015.1134082
Moufarrij, S., Dandapani, M., Arthofer, E., Gomez, S., Srivastava, A., Lopez-Acevedo, M., et al. (2019). Epigenetic therapy for ovarian cancer: Promise and progress. Clin. Epigenetics 11 (1), 7. doi:10.1186/s13148-018-0602-0
Moufarrij, S., Srivastava, A., Gomez, S., Hadley, M., Palmer, E., Austin, P. T., et al. (2020). Combining DNMT and HDAC6 inhibitors increases anti-tumor immune signaling and decreases tumor burden in ovarian cancer. Sci. Rep. 10, 3470. doi:10.1038/s41598-020-60409-4
Munster, P. N. (2020). Reversing therapy resistance with epigenetic-immune modification. (Pembrolizumab, Vorinostat, Tamoxifen). Available at: https://clinicaltrials.gov/ct2/show/NCT02395627.
Munster, P. N., Thurn, K. T., Thomas, S., Raha, P., Lacevic, M., Miller, A., et al. (2011). A phase II study of the histone deacetylase inhibitor vorinostat combined with tamoxifen for the treatment of patients with hormone therapy-resistant breast cancer. Br. J. Cancer 104 (12), 1828–1835. doi:10.1038/bjc.2011.156
National Cancer Institute (2022). Entinostat, nivolumab, and ipilimumab in treating patients with solid tumors that are metastatic or cannot be removed by surgery or locally advanced or metastatic HER2-negative breast cancer. Available at: https://clinicaltrials.gov/ct2/show/NCT02453620.
Natoni, F., Diolordi, L., Santoni, C., and Montani, M. S. (2005). Sodium butyrate sensitises human pancreatic cancer cells to both the intrinsic and the extrinsic apoptotic pathways. Biochimica Biophysica Acta - Mol. Cell. Res. 1745 (3), 318–329. doi:10.1016/j.bbamcr.2005.07.003
North, B. J., Almeciga-Pinto, I., Tamang, D., Yang, M., Jones, S. S., Quayle, S. N., et al. (2017). Enhancement of pomalidomide anti-tumor response with ACY- 241, a selective HDAC6 inhibitor. PLoS One 12, e0173507. doi:10.1371/journal.pone.0173507
Nyante, S. J., Lee, S. S., Benefield, T. S., Hoots, T. N., and Henderson, L. M. (2017). The association between mammographic calcifications and breast cancer prognostic factors in a population‐based registry cohort. Cancer 123 (2), 219–227. doi:10.1002/cncr.30281
Ojha, R., Chen, I. C., Hsieh, C. M., Nepali, K., Lai, R. W., Hsu, K. C., et al. (2021). Installation of pargyline, a LSD1 inhibitor, in the HDAC inhibitory template culminated in the identification of a tractable Antiprostate cancer agent. J. Med. Chem. 64 (24), 17824–17845. https://clinicaltrials.gov/ct2/show/NCT05268666. doi:10.1021/acs.jmedchem.1c00966
Ota, H., Tokunaga, E., Chang, K., Hikasa, M., Iijima, K., Eto, M., et al. (2006). Sirt1 inhibitor, Sirtinol, induces senescence-like growth arrest with attenuated Ras–MAPK signaling in human cancer cells. Oncogene 25 (2), 176–185. doi:10.1038/sj.onc.1209049
Özdağ, H., Teschendorff, A. E., Ahmed, A. A., Hyland, S. J., Blenkiron, C., Bobrow, L., et al. (2006). Differential expression of selected histone modifier genes in human solid cancers. BMC genomics 7 (1), 90. doi:10.1186/1471-2164-7-90
Page, D. B., Bear, H., Prabhakaran, S., Gatti-Mays, M. E., Thomas, A., Cobain, E., et al. (2019). Two may be better than one: PD-1/PD-L1 blockade combination approaches in metastatic breast cancer. NPJ breast cancer 5 (1), 34. https://clinicaltrials.gov/ct2/show/NCT02708680. doi:10.1038/s41523-019-0130-x
Palmieri, D., Lockman, P. R., Thomas, F. C., Hua, E., Herring, J., Hargrave, E., et al. (2009). Vorinostat inhibits brain metastatic colonization in a model of triple-negative breast cancer and induces DNA double-strand breaks. Clin. Cancer Res. 15 (19), 6148–6157. doi:10.1158/1078-0432.ccr-09-1039
Pant, K., Peixoto, E., Richard, S., and Gradilone, S. A. (2020). Role of histone deacetylases in carcinogenesis: Potential role in cholangiocarcinoma. Cells 9 (3), 780. doi:10.3390/cells9030780
Pant, K., Saraya, A., and Venugopal, S. K. (2017a). Oxidative stress plays a key role in butyrate-mediated autophagy via Akt/mTOR pathway in hepatoma cells. Chemico-biological Interact. 273, 99–106. doi:10.1016/j.cbi.2017.06.001
Pant, K., Yadav, A. K., Gupta, P., Islam, R., Saraya, A., Venugopal, S. K., et al. (2017b). Butyrate induces ROS-mediated apoptosis by modulating miR-22/SIRT-1 pathway in hepatic cancer cells. Redox Biol. 12, 340–349. doi:10.1016/j.redox.2017.03.006
Park, H., Garrido-Laguna, I., Naing, A., Fu, S., Falchook, G. S., Piha-Paul, S. A., et al. (2016). Phase I dose-escalation study of the mTOR inhibitor sirolimus and the HDAC inhibitor vorinostat in patients with advanced malignancy. Oncotarget 7 (41), 67521–67531. https://clinicaltrials.gov/ct2/show/NCT01174199. doi:10.18632/oncotarget.11750
Patra, S. K., Patra, A., and Dahiya, R. (2001). Histone deacetylase and DNA methyltransferase in human prostate cancer. Biochem. biophysical Res. Commun. 287 (3), 705–713. doi:10.1006/bbrc.2001.5639
Peinado, H., Ballestar, E., Esteller, M., and Cano, A. (2004). Snail mediates E-cadherin repression by the recruitment of the Sin3A/histone deacetylase 1 (HDAC1)/HDAC2 complex. Mol. Cell. Biol. 24 (1), 306–319. doi:10.1128/mcb.24.1.306-319.2004
Peng, F.-W., Xuan, J., Wu, T.-T., Xue, J.-Y., Ren, Z.-W., Liu, D.-K., et al. (2016). Design, synthesis and biological evaluation of N-phenylquinazolin-4-amine hybrids as dual inhibitors of VEGFR-2 and HDAC. Eur. J. Med. Chem. 109, 1–12. doi:10.1016/j.ejmech.2015.12.033
Perlmutter, M. A., and Lepor, H. (2007). Androgen deprivation therapy in the treatment of advanced prostate cancer. Rev. Urol. 9 (Suppl. 1), S3–S8.
Pham, M. M., Hinchcliff, E., Avila, M., and Westin, S. N. (2021). The clinical challenges, trials, and errors of combatting poly (ADP-Ribose) polymerase inhibitors resistance. Cancer J. 27 (6), 491–500. https://clinicaltrials.gov/ct2/show/NCT04703920. doi:10.1097/ppo.0000000000000562
Qian, C., Lai, C. J., Bao, R., Wang, D. G., Wang, J., Xu, G. X., et al. (2012). Cancer network disruption by a single molecule inhibitor targeting both histone deacetylase activity and phosphatidylinositol 3-kinase signaling. Clin. cancer Res. 18 (15), 4104–4113. doi:10.1158/1078-0432.ccr-12-0055
Rajan, P. K., Udoh, U. A., Sanabria, J. D., Banerjee, M., Smith, G., Schade, M. S., et al. (2020). The role of histone acetylation-/methylation-mediated apoptotic gene regulation in hepatocellular carcinoma. Int. J. Mol. Sci. 21 (23), 8894. doi:10.3390/ijms21238894
Ramarao-Milne, P., Kondrashova, O., Barry, S., Hooper, J. D., Lee, J. S., Waddell, N., et al. (2021). Histone modifying enzymes in gynaecological cancers. Cancers 13 (4), 816. https://clinicaltrials.gov/ct2/show/NCT04703920. doi:10.3390/cancers13040816
Rathkopf, D. E., Picus, J., Hussain, A., Ellard, S., Chi, K. N., Nydam, T., et al. (2013). A phase 2 study of intravenous panobinostat in patients with castration-resistant prostate cancer. Cancer Chemother. Pharmacol. 72 (3), 537–544. https://clinicaltrials.gov/ct2/show/NCT00667862. doi:10.1007/s00280-013-2224-8
Rawla, P. (2019). Epidemiology of prostate cancer. World J. Oncol. 10 (2), 63–89. doi:10.14740/wjon1191
Rexer, B. N., and Arteaga, C. L. (2012). Intrinsic and acquired resistance to HER2-targeted therapies in HER2 gene-amplified breast cancer: Mechanisms and clinical implications. Crit. Rev. Oncog. 17 (1)–16. doi:10.1615/critrevoncog.v17.i1.20
Rhodes, D. (1997). The nucleosome core all wrapped up. Nature 389 (6648), 231–232. doi:10.1038/38386
Richardson, P. G., Hungria, V. T., Yoon, S. S., Beksac, M., Dimopoulos, M. A., Elghandour, A., et al. (2016). Panobinostatplusbortezomibanddexamethasone in previously treated multiple myeloma: Outcomes by prior treatment. Blood 127, 713–721. doi:10.1182/blood-2015-09-665018
Riggs, M. G., Whittaker, R. G., Neumann, J. R., and Ingram, V. M. (1977). n-Butyrate causes histone modification in HeLa and Friend erythroleukaemia cells. Nature 268 (5619), 462–464. doi:10.1038/268462a0
Rizzolo, P., Silvestri, V., Tommasi, S., Pinto, R., Danza, K., Falchetti, M., et al. (2013). Male breast cancer: Genetics, epigenetics, and ethical aspects. Ann. Oncol. 24, viii75–82. doi:10.1093/annonc/mdt316
Ropero, S., and Esteller, M. (2007). The role of histone deacetylases (HDACs) in human cancer. Mol. Oncol. 1 (1), 19–25. doi:10.1016/j.molonc.2007.01.001
Rosati, R., Chen, B., Patki, M., McFall, T., Ou, S., Heath, E., et al. (2016). Hybrid enzalutamide derivatives with histone deacetylase inhibitor activity decrease heat shock protein 90 and androgen receptor levels and inhibit viability in enzalutamide-resistant C4-2 prostate cancer cells. Mol. Pharmacol. 90 (3), 225–237. doi:10.1124/mol.116.103416
Rubin, E. H., Agrawal, N. G., Friedman, E. J., Scott, P., Mazina, K. E., Sun, L., et al. (2006). A study to determine the effects of food and multiple dosing on the pharmacokinetics of vorinostat given orally to patients with advanced cancer. Clin. Cancer Res. 12 (23), 7039–7045. doi:10.1158/1078-0432.ccr-06-1802
Ruscetti, M., Dadashian, E. L., Guo, W., Quach, B., Mulholland, D. J., Park, J. W., et al. (2016). HDAC inhibition impedes epithelial–mesenchymal plasticity and suppresses metastatic, castration-resistant prostate cancer. Oncogene 35 (29), 3781–3795. doi:10.1038/onc.2015.444
Saha, S., Pal, D., and Kumar, S. (2016). Design, synthesis and antiproliferative activity of hydroxyacetamide derivatives against HeLa cervical carcinoma cell and breast cancer cell line. Trop. J. Pharm. Res. 15 (7), 1401. doi:10.4314/tjpr.v15i7.8
Salimi, V., Shahsavari, Z., Safizadeh, B., Hosseini, A., Khademian, N., Tavakoli-Yaraki, M., et al. (2017). Sodium butyrate promotes apoptosis in breast cancer cells through reactive oxygen species (ROS) formation and mitochondrial impairment. Lipids Health Dis. 16 (1), 208. doi:10.1186/s12944-017-0593-4
Sanaei, M., and Kavoosi, F. (2019). Histone deacetylases and histone deacetylase inhibitors: Molecular mechanisms of action in various cancers. Adv. Biomed. Res. 8, 63. doi:10.4103/abr.abr_142_19
Schech, A., Kazi, A., Yu, S., Shah, P., and Sabnis, G. (2015). Histone deacetylase inhibitor entinostat inhibits tumor-initiating cells in triple-negative breast cancer cells. Mol. cancer Ther. 14 (8), 1848–1857. doi:10.1158/1535-7163.mct-14-0778
Schipper, H., Alla, V., Meier, C., Nettelbeck, D. M., Herchenröder, O., Pützer, B. M., et al. (2014). Eradication of metastatic melanoma through cooperative expression of RNA-based HDAC1 inhibitor and p73 by oncolytic adenovirus. Oncotarget 5 (15), 5893–5907. doi:10.18632/oncotarget.1839
Schröder, R., Illert, A. L., Erbes, T., Flotho, C., Lübbert, M., Duque-Afonso, J., et al. (2021). The epigenetics of breast cancer–Opportunities for diagnostics, risk stratification and therapy. Epigenetics 24, 612–624. https://clinicaltrials.gov/ct2/show/NCT04190056. doi:10.1080/15592294.2021.1940644
Seto, E., and Yoshida, M. (2014). Erasers of histone acetylation: The histone deacetylase enzymes. Cold Spring Harb. Perspect. Biol. 6 (4), a018713. doi:10.1101/cshperspect.a018713
Shah, P., Gau, Y., and Sabnis, G. (2014). Histone deacetylase inhibitor entinostat reverses epithelial to mesenchymal transition of breast cancer cells by reversing the repression of E-cadherin. Breast Cancer Res. Treat. 143 (1), 99–111. doi:10.1007/s10549-013-2784-7
Shankar, S., and Srivastava, R. K. (2008). Histone deacetylase inhibitors: Mechanisms and clinical significance in cancer: HDAC inhibitor-induced apoptosis. Adv. Exp. Med. Biol. 615, 261–298. doi:10.1007/978-1-4020-6554-5_13
Shaytan, A. K., Landsman, D., and Panchenko, A. R. (2015). Nucleosome adaptability conferred by sequence and structural variations in histone H2A–H2B dimers. Curr. Opin. Struct. Biol. 32, 48–57. doi:10.1016/j.sbi.2015.02.004
Siegel, R. L., Miller, K. D., Fuchs, H. E., and Jemal, A. (2022). Cancer statistics, 2022. Ca. A Cancer J. Clin. 12, 7–33. doi:10.3322/caac.21708
Siegel, R. L., Miller, K. D., and Jemal, A. (2019). Cancer statistics, 2019. Ca. A Cancer J. Clin. 69 (1), 7–34. doi:10.3322/caac.21551
Singal, R., Ramachandran, K., Gordian, E., Quintero, C., Zhao, W., Reis, I. M., et al. (2015). Phase I/II study of azacitidine, docetaxel, and prednisone in patients with metastatic castration-resistant prostate cancer previously treated with docetaxel-based therapy. Clin. Genitourin. cancer 13 (1), 22–31. https://clinicaltrials.gov/ct2/show/NCT00503984. doi:10.1016/j.clgc.2014.07.008
Singh, A. K., Bishayee, A., and Pandey, A. K. (2018). Targeting histone deacetylases with natural and synthetic agents: An emerging anticancer strategy. Nutrients 10 (6), 731. doi:10.3390/nu10060731
Singh, A., Patel, V. K., and Rajak, H. (2021). Appraisal of pyrrole as connecting unit in hydroxamic acid based histone deacetylase inhibitors: Synthesis, anticancer evaluation and molecular docking studies. J. Mol. Struct. 1240, 130590. doi:10.1016/j.molstruc.2021.130590
Singh, B. N., Zhou, H., Li, J., Tipton, T., Wang, B., Guo, S., et al. (2011). Preclinical studies on histone deacetylase inhibitors as therapeutic reagents for endometrial and ovarian cancers. Future Oncol. 7 (12), 1415–1428. doi:10.2217/fon.11.124
Smith, Haller J., Michael Straughn, J., Buchsbaum, Donald J., and Arend, Rebecca C. (2017). Epigenetic therapy for the treatment of epithelial ovarian cancer: A clinical review. Gynecol. Oncol. Rep. 20, 81–86. doi:10.1016/j.gore.2017.03.007
Smith, J. G., Yokoyama, W. H., and German, J. B. (1998). Butyric acid from the diet: Actions at the level of gene expression. Crit. Rev. Food Sci. Nutr. 38 (4), 259–297. doi:10.1080/10408699891274200
Sørlie, T., Perou, C. M., Tibshirani, R., Aas, T., Geisler, S., Johnsen, H., et al. (2001). Gene expression patterns of breast carcinomas distinguish tumor subclasses with clinical implications. Proc. Natl. Acad. Sci. U. S. A. 98 (19), 10869–10874. doi:10.1073/pnas.191367098
Stanbrough, M., Bubley, G. J., Ross, K., Golub, T. R., Rubin, M. A., Penning, T. M., et al. (2006). Increased expression of genes converting adrenal androgens to testosterone in androgen-independent prostate cancer. Cancer Res. 66 (5), 2815–2825. doi:10.1158/0008-5472.can-05-4000
Stenzel, K., Hamacher, A., Hansen, F. K., Gertzen, C. G. W., Senger, J., Marquardt, V., et al. (2017). Alkoxyurea-based histone deacetylase inhibitors increase cisplatin potency in chemoresistant cancer cell lines. J. Med. Chem. 60 (13), 5334–5348. doi:10.1021/acs.jmedchem.6b01538
Stoyanova, T., Cooper, A. R., Drake, J. M., Liu, X., Armstrong, A. J., Pienta, K. J., et al. (2013). Prostate cancer originating in basal cells progresses to adenocarcinoma propagated by luminal-like cells. Proc. Natl. Acad. Sci. U. S. A. 110 (50), 20111–20116. doi:10.1073/pnas.1320565110
Sun, H., Mediwala, S. N., Szafran, A. T., Mancini, M. A., and Marcelli, M. (2016). CUDC-101, a novel inhibitor of full-length androgen receptor (flAR) and androgen receptor variant 7 (AR-V7) activity: Mechanism of action and in vivo efficacy. Horm. Cancer 7 (3), 196–210. doi:10.1007/s12672-016-0257-2
Tae, I. H., Park, E. Y., Dey, P., Son, J. Y., Lee, S. Y., Jung, J. H., et al. (2018). Novel SIRT1 inhibitor 15-deoxy-Δ12, 14-prostaglandin J2 and its derivatives exhibit anticancer activity through apoptotic or autophagic cell death pathways in SKOV3 cells. Int. J. Oncol. 53 (6), 2518–2530. doi:10.3892/ijo.2018.4561
Takai, N., Narahara, H., Takai, N., and Narahara, H. (2007). Human endometrial and ovarian cancer cells: Histone deacetylase inhibitors exhibit antiproliferative activity, potently induce cell cycle arrest, and stimulate apoptosis. Curr. Med. Chem. 14 (24), 2548–2553. doi:10.2174/092986707782023299
Takai, Noriyuki, and Narahara, Hisashi (2010). Histone deacetylase inhibitor therapy in epithelial ovarian cancer. J. Oncol. 2010, 1–6. doi:10.1155/2010/458431
Tan, Jiahuai, Cang, Shundong, Ma, Yuehua, Petrillo, Richard L., and Liu, Delong (2010). Novel histone deacetylase inhibitors in clinical trials as anti-cancer agents. J. Hematol. Oncol. 3, 5–13. doi:10.1186/1756-8722-3-5
Tate, C. R., Rhodes, L. V., Segar, H. C., Driver, J. L., Pounder, F. N., Burow, M. E., et al. (2012). Targeting triple-negative breast cancer cells with the histone deacetylase inhibitor panobinostat. Breast Cancer Res. 14 (3), R79. doi:10.1186/bcr3192
Telles, E., and Seto, E. (2012). Modulation of cell cycle regulators by HDACs. Front. Biosci. 4, 303–839. doi:10.2741/s303
Terao, Y., Nishida, J. I., Horiuchi, S., Rong, F., Ueoka, Y., Matsuda, T., et al. (2001). Sodium butyrate induces growth arrest and senescence‐like phenotypes in gynecologic cancer cells. Int. J. Cancer 94 (2), 257–267.
Terranova-Barberio, M., Thomas, S., Ali, N., Pawlowska, N., Park, J., Krings, G., et al. (2017). HDAC inhibition potentiates immunotherapy in triple negative breast cancer. Oncotarget 8 (69), 114156–114172. doi:10.18632/oncotarget.23169
Thomas, S., and Munster, P. N. (2009). Histone deacetylase inhibitor induced modulation of anti-estrogen therapy. Cancer Lett. 280 (2), 184–191. doi:10.1016/j.canlet.2008.12.026
Tian, Y., Liu, G., Wang, H., Tian, Z., Cai, Z., Zhang, F., et al. (2017). Valproic acid sensitizes breast cancer cells to hydroxyurea through inhibiting RPA2 hyperphosphorylation-mediated DNA repair pathway. DNA repair 58, 1–12. doi:10.1016/j.dnarep.2017.08.002
Tomita, Y., Lee, M. J., Lee, S., Tomita, S., Chumsri, S., Cruickshank, S., et al. (2016). The interplay of epigenetic therapy and immunity in locally recurrent or metastatic estrogen receptor-positive breast cancer: Correlative analysis of ENCORE 301, a randomized, placebo-controlled phase II trial of exemestane with or without entinostat. Oncoimmunology 5 (11), e1219008. doi:10.1080/2162402x.2016.1219008
Trapani, D., Esposito, A., Criscitiello, C., Mazzarella, L., Locatelli, M., Minchella, I., et al. (2017). Entinostat for the treatment of breast cancer. Expert Opin. investigational drugs 26 (8), 965–971. doi:10.1080/13543784.2017.1353077
Tryfonidis, K., Zardavas, D., Katzenellenbogen, B. S., and Piccart, M. (2016). Endocrine treatment in breast cancer: Cure, resistance and beyond. Cancer Treat. Rev. 50, 68–81. doi:10.1016/j.ctrv.2016.08.008
Tsuji, N., Kobayashi, M., Nagashima, K., Wakisaka, Y., and Koizumi, K. (1976). A new antifungal antibiotic, trichostatin. J. Antibiot. (Tokyo). 29 (1), 1–6. doi:10.7164/antibiotics.29.1
Ukimura, O. (2010). A randomized trial of external beam radiotherapy versus cryoablation in patients with localized prostate cancer. J. Endourology 24 (8), 1217–1219.
Vaishampayan, U. N., Narayan, V., Wise, D., Lang, J. M., Lowentritt, B. H., Mellado, B., et al. (2018). A phase Ib open-label, dose escalation and expansion study to investigate the safety, pharmacokinetics, pharmacodynamics and clinical activity of GSK525762 in combination with abiraterone or enzalutamide in metastatic castrate-resistant prostate cancer. J. Clin. Oncol. 36 (6_Suppl. l), TPS391. doi:10.1200/JCO.2018.36.6_suppl.TPS391
Vaziri, H., Dessain, S. K., Eaton, E. N., Imai, S. I., Frye, R. A., Pandita, T. K., et al. (2001). hSIR2SIRT1 functions as an NAD-dependent p53 deacetylase. Cell. 107 (2), 149–159. doi:10.1016/s0092-8674(01)00527-x
Ververis, K., and Karagiannis, T. C. (2012). An atlas of histone deacetylase expression in breast cancer: Fluorescence methodology for comparative semi-quantitative analysis. Am. J. Transl. Res. 4 (1), 24–43.
Vogelauer, M., Krall, A. S., McBrian, M. A., Li, J. Y., and Kurdistani, S. K. (2012). Stimulation of histone deacetylase activity by metabolites of intermediary metabolism. J. Biol. Chem. 287 (38), 32006–32016. doi:10.1074/jbc.m112.362467
Vogl, D. T., Raje, N., Jagannath, S., Richardson, P., Hari, P., Orlowski, R., et al. (2017). Ricolinostat, the first selective histone deacetylase 6 inhibitor, in combination with bortezomib and dexamethasone for relapsed or refractory multiple myeloma. Clin. Cancer Res. 23, 3307–3315. doi:10.1158/1078-0432.ccr-16-2526
Von Burstin, J., Eser, S., Paul, M. C., Seidler, B., Brandl, M., Messer, M., et al. (2009). E-cadherin regulates metastasis of pancreatic cancer in vivo and is suppressed by a SNAIL/HDAC1/HDAC2 repressor complex. Gastroenterology 137 (1), 361–371.e5. doi:10.1053/j.gastro.2009.04.004
Waltregny, D., North, B., Van Mellaert, F., de Leval, J., Verdin, E., Castronovo, V., et al. (2004). Screening of histone deacetylases (HDAC) expression in human prostate cancer reveals distinct class I HDAC profiles between epithelial and stromal cells. Eur. J. Histochem. 48 (3), 273–290.
Wang, C., Chen, L., Hou, X., Li, Z., Kabra, N., Ma, Y., et al. (2006). Interactions between E2F1 and SirT1 regulate apoptotic response to DNA damage. Nat. Cell. Biol. 8 (9), 1025–1031. doi:10.1038/ncb1468
Wang, H. G., Huang, X. D., Shen, P., Li, L. R., Xue, H. T., Ji, G. Z., et al. (2013). Anticancer effects of sodium butyrate on hepatocellular carcinoma cells in vitro. Int. J. Mol. Med. 31 (4), 967–974. doi:10.3892/ijmm.2013.1285
Wang, J., Kim, T. H., Ahn, M. Y., Lee, J., Jung, J. H., Choi, W. S., et al. (2012). Sirtinol, a class III HDAC inhibitor, induces apoptotic and autophagic cell death in MCF-7 human breast cancer cells. Int. J. Oncol. 41 (3), 1101–1109. doi:10.3892/ijo.2012.1534
Wang, L., Li, H., Ren, Y., Zou, S., Fang, W., Jiang, X., et al. (2016). Targeting HDAC with a novel inhibitor effectively reverses paclitaxel resistance in non-small cell lung cancer via multiple mechanisms. Cell. Death Dis. 7 (1), e2063. doi:10.1038/cddis.2015.328
Wang, S. (2022). Tucidinosta combined with metronomic capecitabine and endocrine therapy for advanced HR-positive, HER2-negative breast cancer after CDK4/6 inhibitor. Available at: https://www.clinicaltrials.gov/ct2/show/NCT05411380.
Wang, X., Qi, Y., Kong, X., Zhai, J., Li, Y., Song, Y., et al. (2019). Immunological therapy: A novel thriving area for triple-negative breast cancer treatment. Cancer Lett. 442, 409–428. https://clinicaltrials.gov/ct2/show/NCT02393794. doi:10.1016/j.canlet.2018.10.042
Wang, Y., He, J., Liao, M., Hu, M., Li, W., Ouyang, H., et al. (2019). An overview of Sirtuins as potential therapeutic target: Structure, function and modulators. Eur. J. Med. Chem. 161, 48–77. doi:10.1016/j.ejmech.2018.10.028
Wang, Y. C., Peterson, S. E., and Loring, J. F. (2014). Protein post-translational modifications and regulation of pluripotency in human stem cells. Cell. Res. 24 (2), 143–160. doi:10.1038/cr.2013.151
Wang, Z. A., Toivanen, R., Bergren, S. K., Chambon, P., and Shen, M. M. (2014). Luminal cells are favored as the cell of origin for prostate cancer. Cell. Rep. 8 (5), 1339–1346. doi:10.1016/j.celrep.2014.08.002
Wawruszak, A., Borkiewicz, L., Okon, E., Kukula-Koch, W., Afshan, S., Halasa, M., et al. (2021a). Vorinostat (SAHA) and breast cancer: An overview. Cancers 13 (18), 4700. https://clinicaltrials.gov/ct2/show/NCT03742245. doi:10.3390/cancers13184700
Wawruszak, A., Halasa, M., Okon, E., Kukula-Koch, W., and Stepulak, A. (2021b). Valproic acid and breast cancer: State of the art in 2021. Cancers 13 (14), 3409. https://clinicaltrials.gov/ct2/show/NCT04315233. doi:10.3390/cancers13143409
Wei, M., Xie, M., Zhang, Z., Wei, Y., Zhang, J., Pan, H., et al. (2020). Design and synthesis of novel Flavone-based histone deacetylase inhibitors antagonizing activation of STAT3 in breast cancer. Eur. J. Med. Chem. 206, 112677. doi:10.1016/j.ejmech.2020.112677
Weichert, W., Röske, A., Gekeler, V., Beckers, T., Stephan, C., Jung, K., et al. (2008). Histone deacetylases 1, 2 and 3 are highly expressed in prostate cancer and HDAC2 expression is associated with shorter PSA relapse time after radical prostatectomy. Br. J. Cancer 98 (3), 604–610. doi:10.1038/sj.bjc.6604199
West, A. C., and Johnstone, R. W. (2014). New and emerging HDAC inhibitors for cancer treatment. J. Clin. Invest. 124 (1), 30–39. doi:10.1172/jci69738
Wheler, J. J., Janku, F., Falchook, G. S., Jackson, T. L., Fu, S., Naing, A., et al. (2014). Phase I study of anti-VEGF monoclonal antibody bevacizumab and histone deacetylase inhibitor valproic acid in patients with advanced cancers. Cancer Chemother. Pharmacol. 73 (3), 495–501. https://clinicaltrials.gov/ct2/show/NCT00530907. doi:10.1007/s00280-014-2384-1
Wu, V. S., Kanaya, N., Lo, C., Mortimer, J., and Chen, S. (2015). From bench to bedside: What do we know about hormone receptor-positive and human epidermal growth factor receptor 2-positive breast cancer. J. Steroid Biochem. Mol. Biol. 153, 45–53. doi:10.1016/j.jsbmb.2015.05.005
Yamashita, M., Tahara, T., Hayakawa, S., Matsumoto, H., Wada, S.-i., Tomioka, K., et al. (2018). Synthesis and biological evaluation of histone deacetylase and DNA topoisomerase II-Targeted inhibitors. Bioorg. Med. Chem. 26 (8), 1920–1928. doi:10.1016/j.bmc.2018.02.042
Yang, Qilian, Yang, Yuqing, Zhou, Nianxin, Tang, Kexin, Lau, Wayne Bond, Lau, Bonnie, et al. (2018). Epigenetics in ovarian cancer: Premise, properties, and perspectives. Mol. Cancer 17 (1), 109. doi:10.1186/s12943-018-0855-4
Yano, Mitsutake, Yasuda, Masanori, Sakaki, Mika, Nagata, Koji, Fujino, Takashi, Arai, Eiichi, et al. (2018). Association of histone deacetylase expression with histology and prognosis of ovarian cancer. Oncol. Lett. 15 (3), 3524–3531. doi:10.3892/ol.2018.7726
Yao, D., Jiang, J., Zhang, H., Huang, Y., Huang, J., Wang, J., et al. (2021). Design, synthesis and biological evaluation of dual mTOR/HDAC6 inhibitors in MDA-MB-231 cells. Bioorg. Med. Chem. Lett. 47, 128204. doi:10.1016/j.bmcl.2021.128204
Yao, D., Li, C., Jiang, J., Huang, J., Wang, J., He, Z., et al. (2020). Design, synthesis and biological evaluation of novel HDAC inhibitors with improved pharmacokinetic profile in breast cancer. Eur. J. Med. Chem. 205, 112648. doi:10.1016/j.ejmech.2020.112648
Yardley, D. A., Ismail-Khan, R. R., Melichar, B., Lichinitser, M., Munster, P. N., Klein, P. M., et al. (2013). Randomized phase II, double-blind, placebo-controlled study of exemestane with or without entinostat in postmenopausal women with locally recurrent or metastatic estrogen receptor-positive breast cancer progressing on treatment with a nonsteroidal aromatase inhibitor. J. Clin. Oncol. 31 (17), 2128–2135. doi:10.1200/jco.2012.43.7251
Yasui, W., Oue, N., Ono, S., Mitani, Y., Ito, R., Nakayama, H., et al. (2003). Histone acetylation and gastrointestinal carcinogenesis. Ann. N. Y. Acad. Sci. 983 (1), 220–231. doi:10.1111/j.1749-6632.2003.tb05977.x
Yee, A. J., Bensinger, W. I., Supko, J. G., Voorhees, P. M., Berdeja, J. G., Richardson, P. G., et al. (2016). Ricolinostat plus lenalidomide, and dexamethasone in relapsed or refractory multiple myeloma: A multicentre phase 1b trial. Lancet Oncol. 17, 1569–1578. doi:10.1016/s1470-2045(16)30375-8
Yeung, F., Hoberg, J. E., Ramsey, C. S., Keller, M. D., Jones, D. R., Frye, R. A., et al. (2004). Modulation of NF‐κB‐dependent transcription and cell survival by the SIRT1 deacetylase. EMBO J. 23 (12), 2369–2380. doi:10.1038/sj.emboj.7600244
Yin, Y., Zhang, M., Dorfman, R. G., Li, Y., Zhao, Z., Pan, Y., et al. (2017). Histone deacetylase 3 overexpression in human cholangiocarcinoma and promotion of cell growth via apoptosis inhibition. Cell. Death Dis. 8 (6), e2856. doi:10.1038/cddis.2016.457
Zhang, J., and Zhong, Q. (2014). Histone deacetylase inhibitors and cell death. Cell. Mol. Life Sci. 71 (20), 3885–3901. doi:10.1007/s00018-014-1656-6
Zhang, K., Yao, Y., Tu, Z., Liao, C., Wang, Z., Qiu, Y., et al. (2020). Discovery of class I histone deacetylase inhibitors based on romidpesin with promising selectivity for cancer cells. Future Med. Chem. 12 (4), 311–323. doi:10.4155/fmc-2019-0290
Zhang, Z., Yamashita, H., Toyama, T., Sugiura, H., Omoto, Y., Ando, Y., et al. (2004). HDAC6 expression is correlated with better survival in breast cancer. Clin. Cancer Res. 10 (20), 6962–6968. doi:10.1158/1078-0432.ccr-04-0455
Zhou, Q., Atadja, P., and Davidson, N. E. (2007). Histone deacetylase inhibitor LBH589 reactivates silenced estrogen receptor alpha (ER) gene expression without loss of DNA hypermethylation. Cancer Biol. Ther. 6 (1), 64–69. doi:10.4161/cbt.6.1.3549
Zhou, X., Marks, P. A., Rifkind, R. A., and Richon, V. M. (2001). Cloning and characterization of a histone deacetylase, HDAC9. Proc. Natl. Acad. Sci. U. S. A. 98 (19), 10572–10577. doi:10.1073/pnas.191375098
Ziadeh, T., and Kourie, H. R. (2021). Poly (ADP-ribose) polymerase inhibitors in prostate cancer: A cornerstone in precision oncology. Pharmacogenomics 22 (18), 1237–1250. https://clinicaltrials.gov/ct2/show/NCT04703920. doi:10.2217/pgs-2021-0119
Keywords: HDAC inhibitors (HDACi), prostate cancer, breast cancer, ovarian cancer, Histone deacetylase (HDAC)
Citation: Pramanik SD, Kumar Halder A, Mukherjee U, Kumar D, Dey YN and R M (2022) Potential of histone deacetylase inhibitors in the control and regulation of prostate, breast and ovarian cancer. Front. Chem. 10:948217. doi: 10.3389/fchem.2022.948217
Received: 19 May 2022; Accepted: 27 June 2022;
Published: 12 August 2022.
Edited by:
Ahmed Wahid, Alexandria University, EgyptReviewed by:
A. Ganesan, University of East Anglia, United KingdomJacob Peedicayil, Christian Medical College and Hospital, India
Copyright © 2022 Pramanik, Kumar Halder, Mukherjee, Kumar, Dey and R. This is an open-access article distributed under the terms of the Creative Commons Attribution License (CC BY). The use, distribution or reproduction in other forums is permitted, provided the original author(s) and the copyright owner(s) are credited and that the original publication in this journal is cited, in accordance with accepted academic practice. No use, distribution or reproduction is permitted which does not comply with these terms.
*Correspondence: Yadu Nandan Dey, eWFkdW5hbmRhbjEzMkBnbWFpbC5jb20=; Mogana R, bW9nYW5hQHVjc2l1bml2ZXJzaXR5LmVkdS5teQ==
†These authors have contributed equally to this work