- 1UM-SJTU Joint Institute, Shanghai Jiaotong University, Shanghai, China
- 2State Key Laboratory of Chemical Engineering, East China University of Science and Technology, Shanghai, China
- 3Department of Chemical Engineering, Shanghai Jiao Tong University, Shanghai, China
Methane (CH4) is one of the cleanest fossil fuel resources and is playing an increasingly indispensable role in our way to carbon neutrality, by providing less carbon-intensive heat and electricity worldwide. On the other hand, the atmospheric concentration of CH4 has raced past 1,900 ppb in 2021, almost triple its pre-industrial levels. As a greenhouse gas at least 86 times as potent as carbon dioxide (CO2) over 20 years, CH4 is becoming a major threat to the global goal of deviating Earth temperature from the +2°C scenario. Consequently, all CH4-powered facilities must be strictly coupled with remediation plans for unburned CH4 in the exhaust to avoid further exacerbating the environmental stress, among which catalytic CH4 combustion (CMC) is one of the most effective strategies to solve this issue. Most current CMC catalysts are noble-metal-based owing to their outstanding C–H bond activation capability, while their high cost and poor thermal stability have driven the search for alternative options, among which transition metal oxide (TMO) catalysts have attracted extensive attention due to their Earth abundance, high thermal stability, variable oxidation states, rich acidic and basic sites, etc. To date, many TMO catalysts have shown comparable catalytic performance with that of noble metals, while their fundamental reaction mechanisms are explored to a much less extent and remain to be controversial, which hinders the further optimization of the TMO catalytic systems. Therefore, in this review, we provide a systematic compilation of the recent research advances in TMO-based CMC reactions, together with their detailed reaction mechanisms. We start with introducing the scientific fundamentals of the CMC reaction itself as well as the unique and desirable features of TMOs applied in CMC, followed by a detailed introduction of four different kinetic reaction models proposed for the reactions. Next, we categorize the TMOs of interests into single and hybrid systems, summarizing their specific morphology characterization, catalytic performance, kinetic properties, with special emphasis on the reaction mechanisms and interfacial properties. Finally, we conclude the review with a summary and outlook on the TMOs for practical CMC applications. In addition, we also further prospect the enormous potentials of TMOs in producing value-added chemicals beyond combustion, such as direct partial oxidation to methanol.
1 Introduction
CH4 is the cleanest fossil fuel with the highest energy content (50–55 MJ/kg) among all sources. It emits 50–60% less CO2, ∼ 80% less nitrogen oxides (NOx), and almost negligible amount of toxic air pollutants including SOx, Hg, and PM 2.5 compared to that of coals (He L. et al., 2020; Zhang et al., 2021). Today, it has been well recognized that CH4 will play an indispensable role in the paradigm shift to a more sustainable planet. However, as the main component of natural gas, CH4 is the second-largest greenhouse gas after CO2 with a global warming potential almost 86 times that of CO2 over 20 years (He Y. L. et al., 2020).
Since the beginning of the industrial revolution, CH4 emissions have raced past 1,900 ppb in 2021, almost triple its pre-industrial levels, and contributed about 20% to the global greenhouse effect so far (Pratt and Tate, 2018; Palella et al., 2021). Specifically in China, circumstances like the incomplete combustion of fossil fuels, natural gas extraction, animal enteric fermentation, crop cultivation, agricultural residue incineration, and solid waste landfills, etc., have resulted in an annual atmospheric CH4 emission taking up about 60% of the total non-CO2 greenhouse gas emissions (Teng et al., 2019; Gong and Zeng, 2021). In this sense, the environmental benefits of CH4 resource itself will be largely balanced by the unburned emission, if no strict remediation plan in the exhaust stream is considered.
Catalytic combustion is one of the most effective means for CH4 utilization for both clean power generation and emission control (Setiawan et al., 2015; Seeburg et al., 2018). Compared to the traditional flame combustion, where high operational cost and toxic substances such as carbon monoxide (CO) and NOx caused by the high combustion temperatures (>1,400°C) are almost inevitable (Stoian et al., 2021), flameless combustion of CH4 under the aid of a solid catalyst allows for not only a much lower operation temperature and much reduced NOx emission but also a wider range of air-to-fuel ratio with a more stable and efficient combustion process outside the combustion limits (He L. et al., 2020).
At the heart of the CMC reaction lies the development of combustion catalysts, of which the catalytic performance and the cost should be properly balanced for practical implementations. To date, the most extensively explored CMC catalysts are precious metals like palladium, platinum, and rhodium, as is the case in the three-way catalytic converter for emission control in automobiles (Choya et al., 2021).
Noble metal catalysts are typically good at activating the C–H and O–O bonds to form free radicals and triggering the chain reactions, thus driving CMC to a low-temperature regime, while their high cost has never stopped the community searches for promising alternatives of cheaper price (Liu et al., 2015). Over the past decades, TMOs have received tremendous attention as one of the most promising candidates for catalyzing CMC owing to their great Earth abundance, low toxicity, and many outstanding physiochemical properties. Comparable, if not superior, performance to noble-metal-based systems has been reached in some cases such as Fe2O3, Co3O4, etc., (Li J. A. et al., 2019; He Y. L. et al., 2020; Zheng Y. F. et al., 2020; Yang et al., 2022), while with that being said, their practical uses are still limited by the low-temperature activity, resistivity against water, and sulfur tolerance in the combustion environment. Further improvement of the catalytic performance of TMO catalysts would require a well understanding of the active site structures and the corresponding reaction mechanisms, which unfortunately still remain largely controversial at the current stage, primarily due to 1) the high structural complexity of oxides themselves, involving a variety of surface acidic and basic sites; 2) the competitions between lattice oxygen and the molecular oxygen as the oxidizing sources; 3) oxygen exchange behaviors at the solid–gas interface; and 4) various adsorbed oxygen species (Toscani et al., 2019).
This review presents a systematic compilation of TMO-catalyzed CMC with special focus on the reaction mechanisms. We start with a brief introduction of the scientific fundamentals of the CMC reaction. Then, we move on to discuss the unique and desirable features of TMOs for the CMC reaction and different CH4 activation pathways, followed by a detailed introduction of four different kinetic reaction models. Next, we categorize the TMOs of interests into single and mixed systems, summarizing their specific morphology characterization, catalytic performance, kinetic properties, with special emphasis on the reaction mechanisms and interfacial properties. Finally, we conclude the review with a summary and outlook on the TMOs for practical CMC applications. Last but not least, we also further prospect the enormous potentials of TMOs beyond CMC, such as partial oxidation to value-added chemicals.
2 Reaction fundamentals
2.1 Catalytic CH4 combustion reaction
CH4 is an extremely stable and highly symmetrical tetrahedral structure formed by four identical C–H bonds. In CH4, the sp3-hybridized carbon atom locates in the center of the regular tetrahedron, and four hydrogen atoms are distributed on the respective four vertices. CH4 possesses a high ionization potential (12.5 eV), a low electron affinity (4.4 eV), and a high C–H bond energy (434 kJ/mol), rendering both the nucleophilic and electrophilic attacks on CH4 extremely challenging under mild conditions (Vickers et al., 2015). The complete oxidation of CH4 proceeds through the following reaction (1):
As the combustion reaction is highly exothermic, side reactions such as CH4–CO2 reforming (Eq. 2), CH4-steam reforming (Eq. 3), reverse water gas shift reaction (Eq. 4), partial oxidation (Eq. 5), and water gas shift reaction (Eq. 6) are almost inevitable at high temperature, as shown in the following reactions (Horn and Schlögl, 2014):
In the 1970s, Pfefferle et al. proposed the process of “heterogeneous catalytic combustion” (Pfefferle and Pfefferle, 1987), where a solid catalyst can be used to combust CH4 in a flameless manner at a much lower reaction temperature (<500°C). Compared with traditional homogeneous flame combustion, the catalytic process owns advantages such as low light-off temperatures, reduced pollutant emission, wide operation ranges, and lower reactor requirement.
The CMC involves heterogeneous reactions, where complex processes such as mass transfer and heat transfer occur between the catalyst surface and the gas phase. Figure 1 shows the schematic relation between the reaction rate and temperature of CMC (He L. et al., 2020).
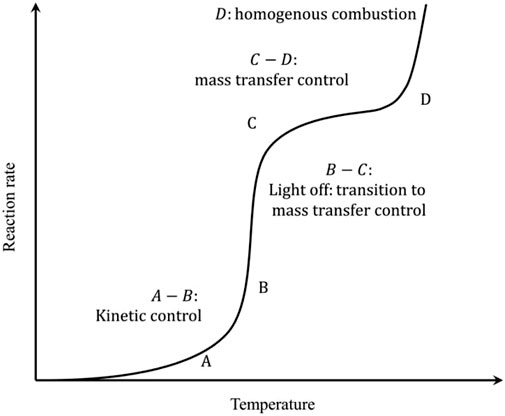
FIGURE 1. The reaction rate of CMC as a function of temperature. Reprinted with permission from He L. et al. (2020). Copyright (2020) Elsevier.
First part of the curve (A–B) is the kinetic-control zone, in which the reaction rate increases slowly with reaction temperature. At low temperatures, the reaction is limited by the intrinsic kinetics such as the dissociation rate of reactants over the catalytic surfaces. The second part of the curve (B–C) is known as the light-off regime where the reaction rate increases almost exponentially with the increase of the temperature. This is due to the drastic increase of the intrinsic reaction kinetics so that the overall reaction rates gradually transition to be controlled by the mass transfer rates. Upon further increase of the temperature (C–D), the mass transfer finally becomes the rate-limiting step, and there appears the rate plateau. The final part of the curve (D) falls under the regime of homogeneous combustion, where high temperature triggers the free radical formation and the chain reaction directly in the gas phase. The latter two high-temperature regimes impose strict requirements on the reactor design to maintain the catalyst structural stability as well as the sufficient mass and heat transfer rates. A more desirable operation window should fall nearby the light-off regime for milder reaction temperatures and sufficiently high reaction rates.
In CMC, other substances like water and sulfur are easy to get adsorbed onto catalyst surfaces under actual operation conditions; thus, the desirable catalysts for CMC should also possess a good anti-water and anti-sulfur poisoning ability beyond high low-temperature activity and thermal stability. In addition, the economic aspects should also be taken into considerations when it comes to large-scale applications.
2.2 Transition metal oxides
TMOs are one of the most important classes of solid catalysts that have been extensively used in a variety of important industrial processes, such as the water gas shift reaction, CO oxidation, methanol synthesis, etc., owing to their excellent thermal stability, variable valence states, and Earth abundance (Tepamatr et al., 2016; Arena et al., 2017; Huang et al., 2021). In water-containing feed, the surface of TMOs undergo acid–base reaction to form surface hydroxyl groups, which react with other reactants via proton or electron transfer (Yang et al., 2022). Transition metal elements could easily alternate between the low oxidation state and the high oxidation state, thus allowing for a facile release and restoration of the lattice oxygen via a redox cycle (Chen et al., 2015). Such redox and acid–base properties have rendered TMOs to be one of the most promising candidates for CMC reactions.
TMOs like Co3O4, Fe2O3, MnO2, etc., have shown catalytic activities comparable to that of noble metal-based systems in CMC (Pu et al., 2017; He Y. L. et al., 2020; Yang et al., 2021), while the performance can be largely affected by preparation method, carrier, doping, and experimental conditions (such as the presence of water vapor and sulfur). The most common preparation methods of TMOs include thermal decomposition, precipitation, combustion synthesis, hydrothermal method, dipping, and sol–gel method (Tang et al., 2008). The different preparation methods can lead to diverse structural properties such as particle size, dispersion, surface area, morphology, crystal plane, metal valence states, defects, and reactive oxygen species. Such variances in the TMO crystal structures may result in drastic differences in the expression of catalytic activity, as was found in Co3O4, Fe2O3, IrO2, etc., where only certain crystal facets are reactive for CMC reactions (Liang et al., 2017; He L. et al., 2020).
The main exposed crystal planes of TMOs with different morphologies have a significant effect on the catalytic activity of CMC. For example, the (111) and (112) crystal planes of Co3O4 and the (110) crystal planes of Fe2O3 are considered to be the best catalytic activity for CMC (Hu et al., 2008; He L. et al., 2020). In addition, the structure dimensionality also induces different catalytic properties due to quantum confinement and geometric effect, as was seen in Co3O4 where the 2D nanosheets and 3D nanoflowers possess drastically different catalytic performances (Jia Y. C. et al., 2016).
TMO-based catalysts are generally supported in CMC for improved particle dispersion, increased specific surface area, and enhanced catalytic performance. Both inactive and active supports are used to disperse TMOs in CMC. For example, Al2O3, as an inactive support, is an acidic oxide capable of adsorbing CH4 through acid–base pairing (Wang and Lin, 2004; Choya et al., 2018a). Active supports, such as CeO2, can directly participate in the CMC reaction through its excellent oxygen storage capacity (Pecchi et al., 2005; Li Y. X. et al., 2009).
Doping TMOs with an impurity element is also an effective strategy to improve their catalytic performance in CMC by inducing a great deal of lattice defects, acidic and basic sites, oxygen vacancies, synergistic effect, etc., (Li J. H. et al., 2009). The increased amount of oxygen vacancies can not only improve the mobility of bulk oxygen in the lattice but also creates additional adsorption sites to promote the molecular adsorption from the gas phase, reducing the activation barrier (Wang H. W. et al., 2019). The dopants in TMOs include metal and non-metal elements. Elements like Ca and Mg are often used as promoters, while Ce and Mn are doped to obtain oxygen vacancies (Pecchi et al., 2011; Chen Y. L. et al., 2021). N is a commonly used dopant in non-metal doping, which can create more lattice distortions and further increase active oxygen species (Buchneva et al., 2012; Li et al., 2018).
One of the most serious issues for TMO catalysts in CMC is their resistance to water poisoning. Most polar facets of TMOs are prone to dissociatively adsorb moisture through an acid–base reaction and form rich surface hydroxyl groups, while this process is highly reversible and self-dehydration can occur at elevated temperatures (He Y. L. et al., 2020). Water vapor on the surface of TMOs reacts with chemisorbed oxygen, which hinder the delivery of reactive oxygen species to active sites. Moreover, the formation of hydroxyl groups hinders the desorption of H2O and CO2 from the surface of TMOs (He L. et al., 2020). Water vapor poisoning is generally reversible owing to the competitive adsorption of water molecules with reactants. Some TMOs possess inherent water tolerant stability, such as NiO, owing to the modification of water for NiO. Tuning the structure and surface topography of the TMO catalysts is effective in obtaining resistance to water vapor (Liu et al., 2017; Xu et al., 2017). Hydrophobic modification of TMOs or their supports may also be an effective means to improve the water resistance and catalytic activity of catalysts (Kuo et al., 2014).
Sulfur poisoning is another serious issue for TMO catalysts in CMC. Natural gas contains a substantial amount of sulfur element, which can be oxidized into sulfur dioxide (SO2) and get adsorbed onto the catalyst surface of the catalyst; subsequent oxidation to sulfate could also occur and thus cause serious sulfur poisoning. Some TMOs, such as Cr2O3, inherently have excellent sulfur resistance due to its low affinity to acid gases (Ordóñez et al., 2008). Sacrificial component such as Mn-based oxide can be used to guarantee the sulfur resistance of the CMC catalyst (Zhong et al., 2019). Similarly, supports such as Al2O3 can react with SO2 to form sulfates, which protects active sites. But it is unfavorable for the recovery of TMO catalysts. In fuel-rich conditions, an elevated temperature (>500°C) is an effective means of recovery for sulfur-poisoned TMO catalysts (Gremminger et al., 2017).
In addition, carbon deposition can also cause serious catalyst deactivation in CMC, as commonly seen in carbonaceous reactions. Note that the effect can be remediated through carefully controlled reaction conditions, such as adjusting the air-to-fuel ratio, the space velocity, and the reaction temperature. In lean combustion scenario where the oxygen content is in excessive amount, the CHn species formed from CH4 dissociation can be rapidly oxidized by dioxygen to form CO2, thus avoiding coke formation. While if a rich fuel mixture is used, the insufficient oxygen content can result in the formation of coke-like carbon (Tao et al., 2015). Under the circumstance of oxygen source coming from lattice oxygen rather than the molecular oxygen in the gas phase, the CH4 to TMO catalyst feed ratio should be instead taken in consideration correspondingly. When the lattice oxygen in the TMO catalysts is insufficient to react with CH4, CH4 will decompose and then form carbon deposition (Wu and Ku, 2018). Another factor that can induce the catalyst deactivation is the space velocity, a small space velocity of the feed can lead to serious carbon deposition due to the long residence time between CHn species and the catalysts (He Y. L. et al., 2020). Furthermore, carbon deposition may also result from the decomposition of CH4 at high temperatures. Promoting coke oxidation can prolong the lifetime of TMO catalysts. TMOs with high oxygen storage capacity such as CeO2 can remove coke deposition through the release of lattice oxygen (Wang Y. N. et al., 2022). Therefore, sufficient oxygen content, reasonably short residence time, and low reaction temperature are necessary to reduce carbon deposition in CMC. For TMOs, in particular, a high oxygen storage capacity will be helpful to prevent coke formation.
2.3 Reaction mechanisms
The reaction mechanisms proposed for CMC over many TMOs remain to be controversial, primarily due to the high structural complexity, different oxygen sources, oxygen-exchange behaviors, etc. Such knowledge gaps hinder us from understanding the precise active site structures and the reaction pathways from a molecular level and consequently the optimization of TMO catalysts for CMC. To this end, the existing mechanistic studies of CMC over TMOs are summarized in this section.
2.3.1 C–H and O–O activation
Dissociation of the first C–H bond is the most critical step of CH4 activation and the following CMC reactions (Feyel et al., 2006). Fu et al. studied different C–H activation mechanisms over various TMOs using cluster model calculations. It was found that H abstraction is the primary working mechanism for the activation of CH4 on most TMOs such as Cr3O9 and Mo3O9, following the hydrogen atom transfer (HAT) route. H abstraction is a single-electron transfer process forming free radicals via the homolytic cleavage of the C–H bond, in which the H atom attacks the terminal oxygen atom from both trans and cis directions. In terms of activation energy, the H abstraction process in trans on the terminal oxygen is more favorable than that from the cis directions (Fu et al., 2006). Li et al. believed that there is also a proton-coupled electron transfer (PCET) process for the activation of C–H bonds on TMOs, that is, transition metal oxide ions and O2− as a Lewis acid–base pair are served as catalytic active sites. In the process, a proton is abstracted from CH4 by the Lewis basic O2−, while the methyl anion is transferred to the Lewis-acidic metal center (Li et al., 2016). Thus, the activation of CH4 on TMOs is usually closely related to the acidity and alkalinity of the surface.
In addition to CH4 activation, the molecular oxygen also plays a significant role in governing the catalytic performance in CMC. The composition and concentration of reactive oxygen species significantly affect the CMC reaction over TMOs. In CMC, there are primarily two types of oxygen species involved in the TMOs, including surface-adsorbed oxygen and lattice oxygen. According to Eq. 7, the dissociative adsorption of molecular oxygen will result in the formation of a series of different adsorbed oxygen species with electrophilic characters, in the sequence of superoxide O2−, peroxide O22−, and charged atomic species O−. The reaction ultimately leads to the incorporation of nucleophilic O2− into the lattice to form lattice oxygen (Wang Y. Q. et al., 2022). It is precisely because of the different activation forms of C–H bonds and oxygen molecules that the mechanism of CMC is such diverse
2.3.2 Kinetic models
The various forms of C–H and O–O activation mechanisms undoubtedly result in a complex reaction network for TMO-catalyzed CMC systems. According to the participation forms of different oxygen species, the proposed reaction mechanisms of the CMC currently include the following four mechanisms (Figure 2). Langmuir–Hinshelwood (L–H) mechanism and the Eley–Rideal (E–R) mechanism are dominated by surface-adsorbed oxygen, while the Mars van Krevelen (MvK) mechanism is dominated by lattice oxygen, and the two-term (T-T) mechanism is cooperatively controlled by the L–H mechanism and the MvK mechanism. The rate equations consistent with these mechanisms are as follows:
L–H mechanism. It is believed that the adsorption activation energy of O2 on the catalyst surface is much smaller than that of CH4, and O2 is preferentially adsorbed on the catalyst surface to form adsorbed oxygen. Meanwhile, adsorbed oxygen species are easier to combine with adsorbed CH4 than O2 due to their electrophilic attack on CH4, so that the hydrogen in CH4 is dissociated, destroying the stable structure of CH4 and generating active methyl radicals to promote the oxidation of CH4. The L–H mechanism is mainly found on noble metals and their supported oxide systems (Becker et al., 2011). Trimm et al. studied methane oxidation over the Pt/Al2O3 porous catalyst and found that the rate equation derived by experimental results was best fit with the L–H mechanism (Trimm and Lam, 1980).
E–R mechanism. In the E–R mechanism, the gaseous O2 is first adsorbed on the metal atoms of TMOs and then further activated to form surface-adsorbed oxygen, which reacts with gaseous CH4 to form active methyl species, finally oxidized to CO2 (Belessi et al., 2001). Veldsink et al. investigated the reaction rate of CMC over a commercially available CuO-γ-A12O3 catalyst and derived a kinetic rate equation from all experimental data. The rate equation is in good accordance with the E–R mechanism, in which catalyst with adsorption of O2, CO2, and H2O, but does not adsorb CH4 (Veldsink et al., 1995).
MvK mechanism. MvK is a redox mechanism where the catalyst will be reduced by CH4 at first, and then the active centers will be reoxidized by molecular oxygen uptakes. It includes surface oxygen reaction and lattice oxygen migration, in which the reaction pathway can be divided into the following steps: the surface dissociative adsorption of gaseous CH4 onto the active site of the catalyst to form adsorbed alkyl and hydroxyl groups, and the reaction spills over to form CO2 and H2O products taking the lattice oxygen as the oxidizing source. After product desorption, the active centers will be reduced with a lot of formation of lattice oxygen vacancies, which can be replenished by either lattice oxygen diffusion from the bulk phase or molecular oxygen adsorption from the gas phase. This reaction mechanism is found for several TMOs like Fe2O3 and Co3O4 (Zasada et al., 2017; He L. et al., 2020).
T-T mechanism. Due to the complex nature of CMC, there are some cases where describing the reaction process on the catalyst by a single mechanism is rather challenging, and then the T-T mechanism is proposed, that is, both surface adsorption oxygen and lattice oxygen participate in the reaction simultaneously (Han et al., 2008). Gaseous oxygen adsorbs on metal active sites and then dissociates to form surface-active oxygen species. It oxidizes CH4 together with lattice oxygen species that migrate from the bulk to the surface, and the lattice oxygen vacancies will be replenished by gaseous oxygen, resulting in some more suitable explanation of reaction mechanisms in CMC over TMOs (Wang et al., 2018).
Although in some cases that an explicit reaction model can be interpreted to well describe the reaction kinetics of TMO-catalyzed CMC reactions, such as Fe2O3, Co3O4, etc., the reaction mechanism may vary based on the structure of TMOs or the reaction conditions. For example, Zasada et al. systematically examined CMC over Co3O4 nanocubes, revealing that the content of oxygen vacancies significantly affects the reaction mechanism. In the low-temperature range (300–450°C), CH4 is primarily activated by monatomic oxygen species on the catalyst, in which facile decarboxylation and dehydroxylation leave the catalyst surface stoichiometric, thus following the L–H mechanism. From 450 to 650°C, the intrafacial dehydroxylation and decarboxylation of the catalyst accelerated the formation of oxygen vacancies, making the catalyst surface slightly reduced. Then, oxygen vacancies are virtually refilled by O2, which is in accordance with the coexistence of L–H and MvK mechanisms. Above 650°C, oxygen vacancies formed from the bulk Co3O4, so causing the active involvement of the lattice oxygen following the MvK mechanism. In this case, the varying reaction conditions change the catalyst redox states, in which the number of oxygen vacancies leads to a variety of reaction mechanisms (Zasada et al., 2017).
The mechanism of CMC may also be significantly altered by doping or substitution of other transition metal ions with different contents into transition metal oxides. Wang et al. demonstrated that the catalytic behavior can be regulated through the substitution of Co by Ni in spinel ZnNixCo2-xO4 oxides (Figure 3). In addition, the difference of catalytic behavior can be explained by the interaction between the O p-band center and metal d-band center. When the metal d-band center exhibits a higher position relative to the O p-band center in Ni-poor ZnNixCo2-xO4 spinel oxides, the catalyst shows a greater metal character, following the E–R mechanism, among which the first C–H bond cleavage and the H2O desorption are considered to be the rate-determining steps. On the contrary, Ni-rich spinel oxides with the higher O p-band center compared with the metal d-band center show greater oxygen character, in accordance with the MvK mechanism, in which the multiple lattice oxygen involved steps are crucial for the entire methane oxidation (Wang T. et al., 2019).
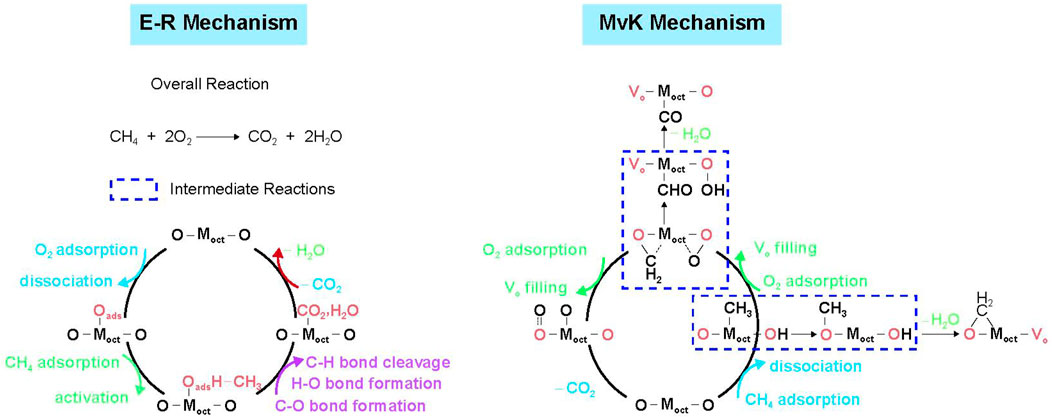
FIGURE 3. The proposed reaction pathways based on the E–R mechanism for Ni-poor spinel oxides and MvK mechanism for Ni-rich spinel oxides. Cited from Wang H. W. et al. (2019).
The mechanism of CMC may also be greatly affected by the reaction temperature. He et al. investigated CMC over α-Fe2O3 via a combined experimental and theoretical study and found that C16O2 formed first, followed by H216O, C16,18O2, H218O, and finally C18O2, where 18O2 was only used in the gaseous molecular oxygen. The numerical analysis of the mass balance revealed that the ratio 16O/18O > 1 at T < 385°C, then 16O/18O ≈ 1 at T ≈ 385°C, and finally 16O/18O < 1 at T > 385°C. These indicate that, below 385°C, the lattice oxygen plays a predominant active role in CMC reaction, in accordance with the MvK mechanism. However, above 385°C, the mechanism becomes more complex and difficult to analyze (He Y. L. et al., 2020).
3 Transition metal oxides for catalytic CH4 combustion
In the application of the CMC, the TMOs such as Co3O4, NiO, MnO2, Cr2O3, Fe2O3, CeO2, CuO, and their binary complex oxides have been widely studied. Therefore, the characteristics of these TMOs and the mechanisms of the CMC over these catalysts are comprehensively reviewed in the following sections in order to provide a general guideline about the construction of well-performed catalytic systems for the CMC. The catalytic performances of different TMOs for CMC are shown in Table 1.
3.1 Co3O4-based catalysts
3.1.1 Single Co3O4
Co3O4 is a p-type semiconducting metal oxide with a typical spinel structure, and it has an array based on a cubic close-packed oxide ion whose lattice parameter is a = 0.811 nm with the space group of Fd3m. In the crystal structure of Co3O4, Co2+ occupies the tetrahedral coordination and Co3+ occupies the octahedral coordination, respectively, with a mean cobalt oxidation state of +2.67. Co3O4 is considered to be one of the most effective co-oxidation catalysts due to the unfilled 3d orbital of Co, the weak Co–O bond strength, a high cycle frequency of redox, and a low barrier of oxygen vacancy. Owing to its unique physical and chemical properties, it has a wide range of applications in sensors, magnetic materials, lithium-ion batteries, and solar cells (Sanchis et al., 2021). In CMC, Co3O4 has attracted the attention of many researchers due to its high stability, variable valence, and excellent catalytic performance. Paredes et al. found the activity of different bulk TMOs catalysts in methane combustion in the following order: Co3O4 > Mn2O3> Cr2O3> CuO > NiO (Paredes et al., 2009). Therefore, Co3O4 is considered to be one of the best candidates for CH4 combustion catalysts among all TMOs and a promising alternative for noble metal combustion catalysts.
According to the previous literature, the common methods for preparing Co3O4 include precipitation, sol–gel method, hydrothermal synthesis, impregnation, thermal decomposition, and solid phase reaction. Choya et al. prepared several bulk Co3O4 catalysts by various synthesis methodologies, among which the solution combustion synthesis route, the basic grinding route, the calcination of the cobalt hydroxycarbonate route, and the precipitation with the sodium carbonate route showed better textural properties than the commercial catalyst due to the higher presence of Co3+ on their surface and further resulted in abundant lattice oxygen species. The catalysts exhibited higher catalytic activities due to their favored mobility of lattice oxygen species (Choya et al., 2022). Wang et al. prepared a series of Co3O4/γ-Al2O3 catalysts by a combination of incipient wetness impregnation (IWI) and subsequent combustion synthesis (CS) method. Results revealed that the CS method exhibited higher catalytic activity than the catalysts prepared by the IWI method, attributing to the higher surface area, lower Co3O4 crystallization, better dispersion, more surface Co3+, as well as easier and faster redox cycle between Co2+ and Co3+ (Wang et al., 2015).
Studies have shown that the catalytic behavior of Co3O4 catalysts strongly depends on its morphology, structure, and crystal planes, which endow them with different electron transfer abilities and different amounts of active sites, promoting catalytic activity and selectivity. The morphological characteristics can be regulated by the preparation method, such as spheres, nanorods, nanowires, nanobelts, and nanosheets.
Wang et al. prepared Co3O4 with the shape of nanosheets and nanospheres by the hydrothermal method in media of ethylene glycol and water, respectively. The concentration of ethylene glycol and the hydrothermal temperature significantly influenced the size and shape of the Co3O4, which showed that the Co3O4 nanosheets exhibited slightly higher catalytic performance than the Co3O4 nanoparticle, as more active oxygen species were found in the former (Wang et al., 2017). Chen et al. controllably synthesized Co3O4 nanocrystals with different morphologies (flower, hexagonal plate, hexagonal sheet, and cube), which showed that the properties of Co3O4 were closely related to the morphology. Compared to cubical Co3O4 with the (100) plane, the flower-like, hexagonal plate-like, and hexagonal sheet-like Co3O4 catalysts were more active, which may be due to more exposed (111) planes (Chen et al., 2016).
In addition, the spatial structures of TMOs also play an important role in the catalytic activity. Sun et al. prepared Co3O4 catalysts with different spatial structures, such as 0D (nanoparticles), 1D (nanorods), 2D (nanoplates), and 3D (mesoporous and microporous) structures. Among them, 2D structures (nanoplates) have the best catalytic activity, which is contributed to the high refractive index of the exposed (112) crystal planes and the role of surface-active species (such as surface-adsorbed oxygen and Co2+) in the catalytic reaction (Sun et al., 2016).
The use of supports can effectively improve the dispersion and prevent the particle agglomeration caused by the sintering of the Co3O4 catalyst, thereby improving the catalytic activity of the catalyst. Feng et al. deposited Co3O4 on the SmMn2O5 (SMO) support to prepare Co/SMO composite catalysts and evaluated the performance of Co/SMO catalysts in oxygen-enriched environments. The results showed that the Co/SMO-50% catalyst had high catalytic activity and strong durability. The strong interaction between Co3O4 and SmMn2O5 played a key role in dispersing and stabilizing Co3O4 by preventing catalyst sintering. Highly dispersed Co3O4 formed more surface lattice oxygen-oxidized CH4, leading to the transformation from Co3+ to Co2+, along with the formation of an oxygen vacancy, which could be compensated by the gaseous oxygen, so following the MvK mechanism (Feng et al., 2018). Dou et al. obtained Co3O4/CeO2 catalysts by supporting Co3O4 nanoparticles on CeO2 nanorods by the deposition precipitation method. The results showed that complete oxidation of CH4 on Co3O4/CeO2 (43.9 kJ/mol) was obviously lower than pure CeO2 (95.1 kJ/mol) and pure Co3O4 (89.7 kJ/mol). Co3O4/CeO2 showed synergistic effect, in which oxygen vacancies on the surface of CeO2 were active centers for activating molecular oxygen in the oxidation reaction, thus promoting the improvement of catalytic activity (Dou et al., 2018).
3.1.2 Doped Co3O4
The element doping has been considered as an effective way to adjust the surface and electronic structures of nanomaterials. Some studies show that introducing oxygen vacancies will have a huge effect on the oxidation reaction. In the element-doped catalyst, Co3O4, as the reactive site, actively participates in CMC, while the doping of other elements often creates more oxygen vacancies to promote the rapid migration of lattice oxygen (Rodríguez-Fernández et al., 2019). Zheng et al. believed that Co2+ was active species in Co3O4. Thus, they prepared Co-In-x oxide via a designed precipitation method by taking N-butylamine as the precipitant. The Co–In–O solid solution phase exhibited a superior activity by doping an appropriate amount of In3+ to replace the Co3+ site of octahedral position, increasing the proportion of active species Co2+, giving rise to abundant active oxygen species, improving reducibility, and optimizing surface acidity. Moreover, Co–In-x oxide also demonstrated excellent stability and water resistance, indicating that the doping of In3+ was beneficial to maintain a certain grain size and crystal phase (Zheng Y. F. et al., 2020). Yu et al. prepared the defective N-doped Co3O4 by efficient N2 plasma treatment for methane oxidation reaction. N-doped Co3O4 could synergistically boost the catalytic performance by increasing active surface oxygen, enhancing redox property, and promoting the C–H bond activation ability. This result may provide a valuable guidance for the defects engineering of Co3O4 for the application of CMC (Yu et al., 2020).
However, doping is not always favorable for the catalytic activity of Co3O4. Choya et al. prepared two bulk Co3O4 catalysts with and without residual sodium by precipitation method. It was found that the presence of Na+ had a negative impact on the properties of the Co3O4 catalyst due to diffusion and migration into the spinel lattice. The insertion of Na+ led to lattice distortion and induced a reduction of the Co3+ into Co2+ owing to high Lewis acidic properties, along with high electron density within the oxygen ions of the lattice, weakened Co–O bonds, and reduced lattice oxygen species, which pointed out that the doping may be detrimental to catalytic performance. In this sense, appropriate precipitants or preparation methods are important for achieving high-performance CMC catalysts (Choya et al., 2018b).
3.1.3 Binary Co3O4-catalysts
Li et al. reported the introduction of an electric field on the Co3O4/Ce0.75Zr0.25 catalyst and found that the presence of the electric field significantly promoted the catalytic oxidation activity of methane. Co3O4 provided active sites and oxygen species for methane oxidation, while CeO2 released lattice O species for the oxidation of CoO and Co to Co3O4. The electric field promoted the reduction of Ce4+ to Ce3+ and promoted the release of oxygen from the lattice. The chemisorption of methane mainly located in the newly formed tetrahedral Co3+ in the electric field rather than the active sites formed by octahedral Co3+ with gaseous oxygen. The adsorbed CH4 was oxidized to carbonates species immediately, followed by the formation of CO2 and refreshment of consumed O species by gaseous oxygen, which is similar to the typical MvK mechanism (Li L. et al., 2019).
3.1.4 Deactivation
Although Co3O4 shows a high catalytic activity for the CMC, it still cannot defeat noble metals owing to its low water and sulfur resistance. Generally, the inhibition of water vapor on the catalytic activity is probably due to its competition with the reactant for the active sites. The inhibition of SO2 is attributed to the formation of sulfate or sulfide on the surface of catalysts, which may cause the loss of active sites, or agglomeration and the loss of surface area. From the existing literature, this problem can be effectively solved when the TMOs are supported on the carriers or some additives are added to the TMOs (Li J. A. et al., 2019).
Li et al. prepared a series of CoOx embedded in the porous SiO2 matrix by a spontaneous deposition method, which showed enhanced catalytic activity as compared with the simply supported CoOx/SiO2 catalyst due to a higher ratio of Oads/Olatt and more active sites obtained by embedded CoOx/SiO2. Due to the competitive adsorption of water and reaction molecules on the active sites, water vapor inhibits the catalytic efficiency. While when H2O was removed, the CH4 conversion nearly fully recovered. Under moisture conditions, the embedded CoOx@SiO2 exhibited a high thermal stability and efficient moisture resistance due to the silica encapsulation (Li K. et al., 2019).
3.1.5 Mechanisms
According to the available mechanism study on CMC over TMOs, CMC over Co3O4 usually follows the MvK mechanism, which means that CH4 is actually oxidized by the oxygen species present in the Co3O4 lattice, followed by the generation of oxygen vacancies. Then, the O2 from the gas phase refills the oxygen vacancies, provoking the subsequent reoxidation of the Co3O4-basedcatalyst. Wu et al. prepared Co3O4–CeO2 mixed oxides using (NH4)2CO3, Na2CO3, and CO(NH2)2 as precipitation agents by a precipitation method. The Co3O4–CeO2 with the homogeneous precipitation of CO(NH2)2 showed excellent catalytic activity owing to the small crystallite size, easy reducibility of Co3+, and high surface Co3+ content at the Co3O4–CeO2 interface. The peculiar structure and morphology of CeO2 played a fundamental role in stabilizing the Co3O4 active phase against sintering and promoting its activity. The authors believed that the pathway of CMC over the Co3O4–CeO2 was in accordance with the MvK mechanism, in which the Ce4+/Ce3+ couple efficiently released oxygen (Wu et al., 2015).
Hu et al. investigated CMC on the Co3O4(110) surface with excellent catalytic performance by first-principles calculations as compared with that on the Co3O4(100) surface. It is found that the optimal reaction pathway of CMC over Co3O4(110) would be CH4 → CH3* → CH3O2c→ CH2O2c → O2cCH2O2c → O2cCHO2c → O2cCHO* →CO2, in which the 2-fold coordinated lattice oxygen (O2c) was the key to the first two C–H bond activations and the C–O bond coupling (Figure 4). According to the figure, H is able to readily transfer swiftly among different surface oxygen species to form adsorbed H2O* for the rapid regeneration of active O2c. The cooperation of multiple active sites not only facilitates the H swift transfer in order to maximally prevent the passivation of the active low-coordinated O2c but also stabilizes surface intermediates during the CMC. Due to the synergistic effect of surface-adsorbed oxygen and lattice oxygen, the reaction pathway belongs to the T-T mechanism, in which the first C–H bond activation step would be the rate-determining step for the CMC on the Co3O4 (110) surface (Hu et al., 2016).
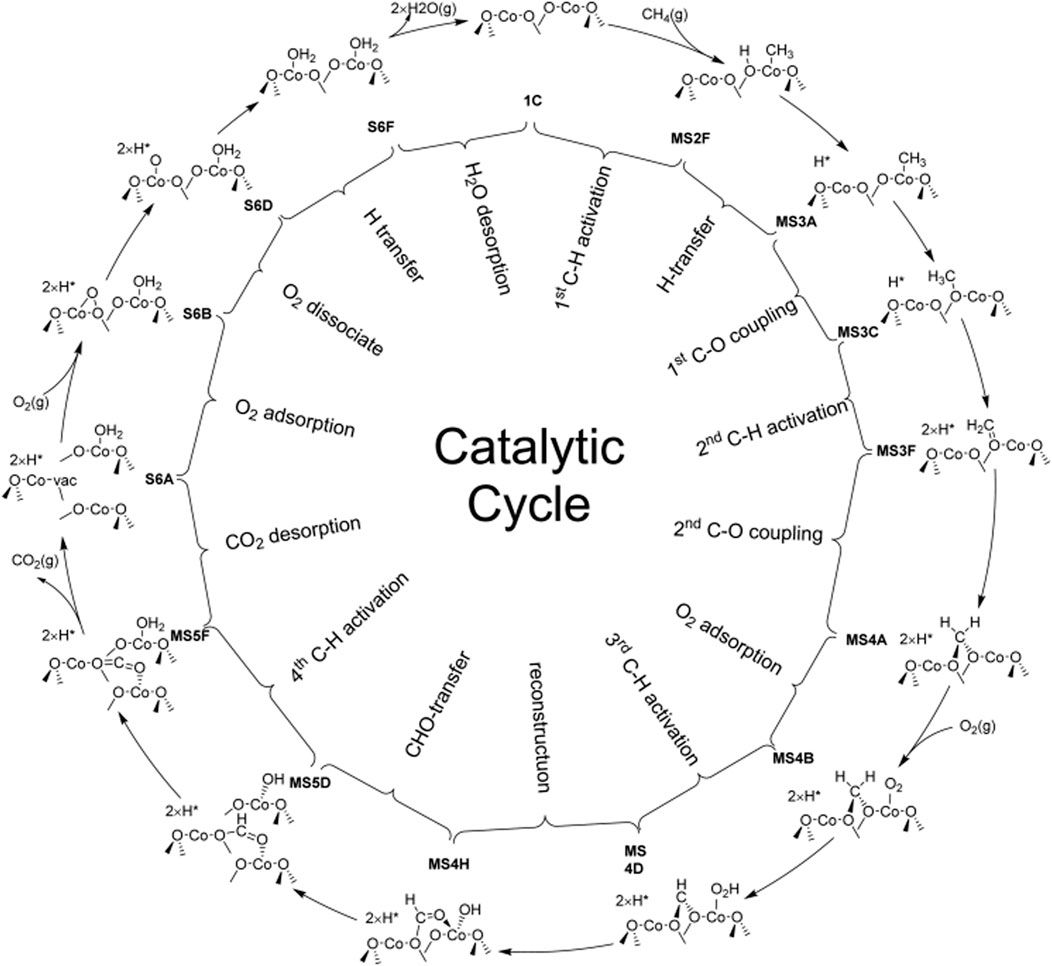
FIGURE 4. Schematic diagram of the whole catalytic cycle of CMC over Co3O4 (110) following the optimal reaction mechanism. Reprinted with permission from Hu et al. (2016). Copyright (2016) American Chemical Society.
However, some researchers have proposed different mechanisms for the CMC over Co3O4. Han et al. prepared a series of Co3O4 nanocrystals with different morphologies via calcining the Co(OH)2 precursor at different temperatures, among which the mesoporous Co3O4 possessed excellent catalytic activity due to the vacancy, antisite, dislocation, and grain boundary defects in the narrow junction regions and the structure of the pore wall. The authors believed that the reaction pathway followed the E–R mechanism, however, without detailed verification (Han et al., 2016).
3.2 NiO-based catalysts
3.2.1 Single NiO
NiO, NaCl-type cubic spar structure, is a typical P-type semiconductor with the intrinsic defects at the Ni2+ metal sites, which give rise to positive holes (p+) to generate Ni3+ (Ni2+ + p+→Ni3+) or O− species (O2− + p+→O−). NiO is extensively used in the field of catalysis, chemical sensors, battery electrodes, and magnetic and electronic devices due to excellent properties such as catalytic activity, thermo-sensitivity, and super-paramagnetic property (Wang et al., 2021). Especially, in the field of catalysis, NiO-based catalysts attract a lot of attention due to the surface electrophilic O2− species being effective for the activation of C–H bonds. NiO have been successfully prepared via a variety of methods, including chemical precipitation, electrode deposition, sol–gel technique, surfactant-template, hydrothermal technique, template-free strategy, microwave-assisted gas/liquid interfacial method, and solvothermal method.
Ye et al. prepared polymorphous NiO with different morphologies, including nanoparticle-based sheets, octahedra, nanosheet-built agglomerates, and nanoparticle-based microsphere, via a simple one-pot thermal decomposition approach. The morphology and crystal properties of NiO can conveniently be achieved by selecting various decomposition temperatures and precursors. The nanoparticle-based sheets and nanosheet-built agglomerates showed a high catalytic performance owing to the small crystal size and large specific surface area by using NiC2O4·2H2O and NiCO3·2Ni(OH)2·4H2O as precursors (Ye et al., 2016).
Chen et al. prepared NiO-NSL nanomaterials with a characteristic nanorod structure through the solid–liquid NH3·H2O precipitation method. The content of Ni2+ on the surface of NiO-NSL was higher than traditional NiO-based catalysts, consistent with DFT calculations in which the energy barrier for the C–H bond activation on Ni2+ was lower than that on Ni3+. However, the authors did not clearly explain the mechanism of the CMC on the NiO-NSL catalyst, but only highlighted the significant impact of Ni2+ on the catalytic performance of the NiO-based catalysts (Chen K. et al., 2021).
3.2.2 Doped NiO
Generally, the synergistic effect induced by the doping can significantly improve catalytic activity. Zhang et al. prepared a series of MnOx–NiO composite oxide catalysts by the co-precipitation method, which exhibits higher catalytic performance compared with the single NiO and MnOx. The characterization results demonstrate that the Ni–Mn–O solid solution formed by the doping of appropriate amount of Mn, showing abundant highly dispersed Mn4+ and higher coordination number as well as certain nickel vacancies, due to the synergy interaction of Ni and Mn (Zhang et al., 2013).
3.2.3 Binary NiO-catalysts
Fan et al. introduced Cu into the NiO lattice to generate the Cu–Ni solid solution with the mesoporous structure by the co-precipitation method. Ni–Cu oxide catalysts manifested superior catalytic activity, moisture tolerance, and durability, owing to more unsaturated Ni atoms and lattice defects. Ni–O–Cu bonds could weaken Ni–O and Cu–O bonds, making the lattice oxygen converted into adsorbed oxygen efficiently and creating more oxygen vacancies to promote the activation and adsorption capacity of Ni–Cu oxide for O2, creating more surface electrophilic species (O22−, O2− and O−). Thus, the reaction pathway was in agreement with the MvK mechanism (Figure 5). The electron densities were redistributed because of the interaction between NiO and CuO, where the surface acid–base properties were adjusted. The higher basicity over Cu–Ni oxide could contribute to the adsorption of CH4 with weak acidity and inhibit the accumulation of surface hydroxyl groups effectively. Meanwhile, the stronger surface acid sites could facilitate the activation of CH4 via heterolytic C–H bond breaking (Fan et al., 2022).
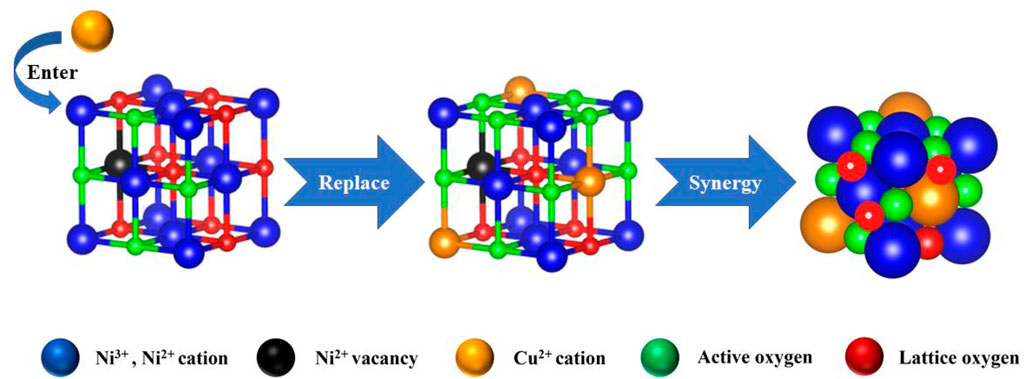
FIGURE 5. Reaction pathway of CMC over Cu–Ni oxide. Cited from Fan et al. (2022).
3.2.4 Deactivation
Through the regulation of preparation method and structure, Ni-based catalysts can obtain excellent resistance to water vapor. Liu et al. prepared the simple metal oxides (Fe2O3, Co3O4, NiO, and CuO) by the thermal decomposition of the corresponding metal nitrates. The NiO possessed larger capability for oxygen adsorption, thus exhibiting the best catalytic activity for CMC as compared to other metal oxides, even superior to the perovskite catalyst LaCoO3. Water vapor promoted CH4 conversion over the NiO catalyst, which could be attributed to H2O could modify NiO surface and promote the activation of O2 and CH4 on the surface (Liu et al., 2017). Xu et al. synthesized NiO catalysts with the mesoporous structure by hydrothermal method with polyethylene glycol (NiO-PEG) and polyvinyl pyrrolidone (NiO-PVP) as soft templates. NiO-PEG and NiO-PVP possessed more mobile-active oxygen species, which was beneficial to the activating of the CH4, due to the mesoporous structure and high surface area. NiO-PEG displayed excellent reaction stability in the presence or absence of water vapor for the reason that its bulk structure possessed certain physical stability (Xu et al., 2017).
3.2.5 Mechanisms
It is generally believed that the pathway of the CMC over nickel-based catalysts is consistent with the MvK mechanism. Shu et al. prepared mesoporous NiO with abundant oxygen defects by a NaCl crystalline scaffold-based method. In kinetic measurements, rCH4 exhibited a near zero-order dependence on O2 partial pressure, indicating that CH4 oxidation was not sensitive to the PO2 partial pressure. Due to the depletion of lattice oxygen, the concentrations of C16O16O and C18O16O were reduced, and the concentration of C18O18O was increased, which further demonstrated that the reaction mechanism was accorded with the MvK mechanism. In this case, the highly abundant lattice oxygen species had great contribution to the excellent performance of NiO (Shu et al., 2022). Wang et al. prepared Zr-promoted NiO nanocatalysts by a designed co-precipitation process. Ni0.89Zr0.11O2-δ solid solution phase exhibited abundant active Ni2+ sites and oxygen vacancies, bringing about the increase in surface acidic–basic sites. The mechanism for CMC could be described, as shown in Figure 6. CH4 was adsorbed on the Ni2+–O2− active site at first and then dissociated to -CH3 and -OH, followed by the formation of formate and carbonate intermediates. Then, the carbonate species converted to CO2. The authors did not mention which mechanism the CMC on Ni0.89Zr0.11O2-δ conforms to, but according to the lattice oxygen and oxygen vacancies involved in the reaction, it is judged that the reaction conforms to the MvK mechanism. Meanwhile, in the stream tests, the steady conversion of CH4 could be well maintained regardless of the presence of H2O due on the doping of Zr (Wang et al., 2021).
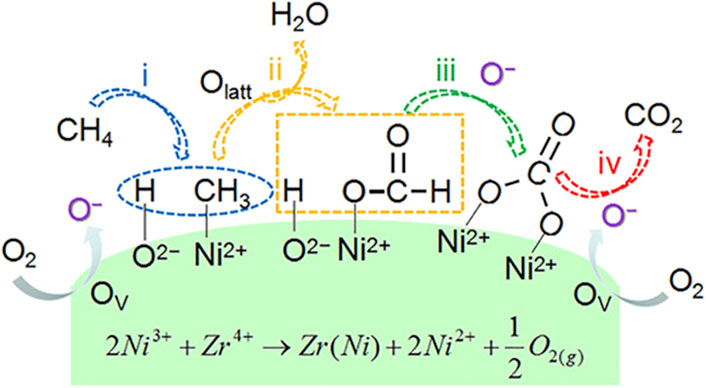
FIGURE 6. Reaction pathway for methane combustion over Ni1−xZrxO2−δ catalysts. Reprinted with permission from Wang et al. (2021). Copyright (2021) American Chemical Society.
3.3 MnO2-based catalysts
3.3.1 Single MnO2
Mn oxides, such as α- and β-MnOx, possess the different polymorphic structures, which exhibit mixed valence state of Mn (Mn2+, Mn3+, and Mn4+), and different ways to link together the basic octahedral [MnO6] units, thereby showing strikingly different and efficient catalytic activities for the CMC (Jia J. B. et al., 2016; Yang et al., 2021). The catalytic activity of the Mn oxides is typically determined by their prepare method, morphology, crystal structure, and degree of oxidation.
Yu et al. prepared γ-MnO2 with abundant surface and lattice defects by a combined ball-milling and selective atom removal method, which showed a high catalytic activity for the CMC owing to the enhanced specific surface area, high Mn4+/Mn3+ ratio, more active oxygen species, and enhanced reducibility (Yu et al., 2019). Wasalathanthri et al. prepared mesoporous amorphous Meso-Mn-A, Meso-Mn2O3, Meso-ε-MnO2 (epsilon phase), and octahedral molecular sieves MnO2 (Meso-OMS-2) via an inverse surfactant micelle method. Meso-OMS-2 showed the highest catalytic activity, attributing to the narrow and monomodal pore size distribution, higher surface area, the oxidation states, and surface oxygen vacancies, promoting the lattice oxygen mobility, following the MvK mechanism (Wasalathanthri et al., 2015).
3.3.2Doped MnO2
In addition, compared to pure MnOx, the doping of other transition metals will also significantly enhance the catalytic performance of Mn-based oxides. Neatu et al. studied CeO2–MnOx catalysts by three methods, illustrating that the CeO2–MnOx catalyst impregnated on the support surface showed superior catalytic activity as compared with the catalyst doped into the bulk. The authors believed that the CMC over the CeO2–MnOx catalyst was in accordance with the MvK mechanism, in which the oxidation of CH4 took place using the lattice oxygen, consecutively with the reduction of Ce4+ and Mn4+ to Ce3+ and Mn3+, respectively. The gaseous O2 was used to reoxidize the surface to assure another catalytic cycle (Neatu et al., 2019).
3.3.3 Deactivation
However, water vapor will affect the catalytic activity of Mn-based catalysts for the CMC to some extent. Akbari et al. prepared nanostructured MnO2 catalysts with various morphologies by the simple hydrothermal method and the solution method, among which the α-MnO2 catalyst with a wire-like morphology exhibited the best performance for CMC. However, the stability of the optimal MnO2 sample reduced to some extent with the water vapor in the reactant feed stream (Figure 7). On one hand, the coverage of the active sites by water vapor inhibited the adsorption of CH4 and O2 on the MnO2 catalyst surface. On the other hand, the -OH group as an inert compound over lattice oxygen could also cause a decline in the catalytic stability (Akbari et al., 2021).
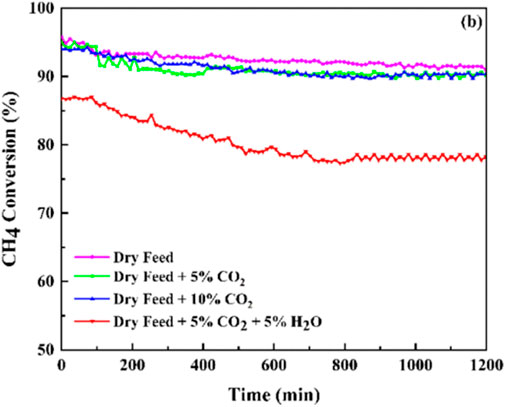
FIGURE 7. Catalytic stability for methane catalytic combustion of the MnO2 catalyst under different feed compositions. Reprinted with permission from Akbari et al. (2021). Copyright (2021) American Chemical Society.
If in an oxidizing atmosphere, SO2 will be oxidized to form sulfate, which will be deposited on the surface of MnO2, hindering the multiple adsorption, activation, and oxidation of CH4 over MnO2, then causing the deterioration of catalytic activity. Zhong et al. synthesized MnCe-RP and MnCe-CP by the redox-precipitation (RP) and co-precipitation (CP) methods, respectively, and studied SO2 resistance of the catalysts (Figure 8). The SO2 would decrease the content of lattice oxygen and Mn4+, so the CH4 conversion of MnCe-CP reduced by 62.45%. On the contrary, the CH4 conversion of MnCe-RP only reduced by 1.08% owing to the excellent morphology and the redox potential of KxMn8O16, absorbing and oxidizing SO2 to sulfides, freeing from the poison of the downstream catalyst (Zhong et al., 2019).
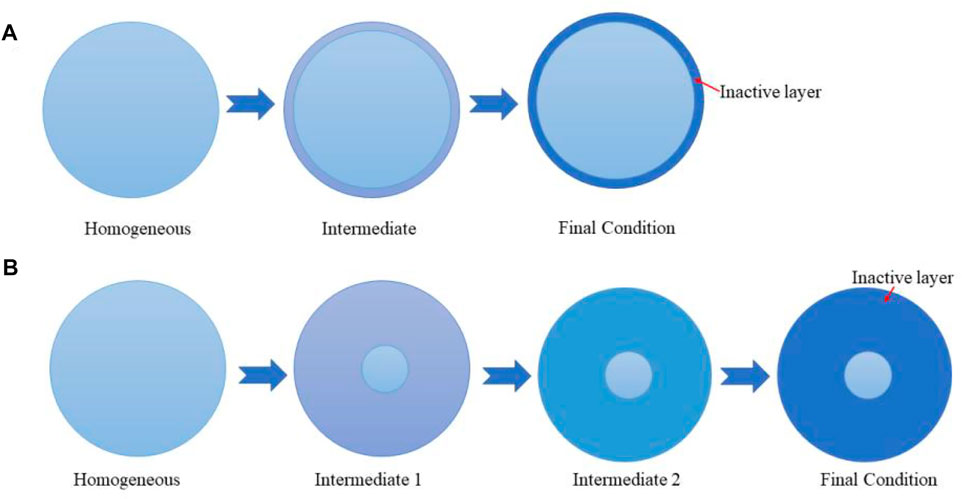
FIGURE 8. SO2-poisoning mechanism over MnCe-CP (A) and MnCe-RP (B). Cited from Zhong et al. (2019).
3.3.4 Mechanisms
There are reported contradictory claims on the CMC over Mn oxides, such as that based on the L–H mechanism involving adsorbed oxygen and that based on the MvK mechanism concerning lattice oxygen.
Zhang et al. synthesized nanocubic MnO2 (MnO2-C) with planes (101) and nanorod-shaped MnO2 (MnO2-R) with planes (110) by a hydrothermal process, illustrating that the morphologies of MnO2 had significant influence on the catalytic performances of CMC. Due to higher BET surface area, smaller crystalline size, more surface oxygen vacancies, and better low-temperature reducibility, MnO2-C displayed higher catalytic performances and good thermal stability compared with MnO2-R. The authors believed that the surface oxygen of MnO2-C could react with the adsorbed C–H to generate the carboxylate species for the reason of more surface oxygen vacancies, followed by the oxidation of carboxylate species to CO2, through the L–H mechanism (Zhang et al., 2019). Jia et al. synthesized two types of single-phase manganese oxides MnO2 with different levels of nonstoichiometric defects, α-MnO2 (Mn1) and γ-MnO2 (Mn2), compared with a stoichiometric Mn2O3 (Mn3) as a reference. Results revealed that both Mn3 and Mn1 exhibited enhanced activities due to more structural defects. It was found that CH4 was directly oxidized to carbonates on the surface of the pre-oxidized Mn1 and subsequently transformed to CO2 in the absence of gaseous O2 by the in situ DRIFTS and CH4-TPD test, which showed that the active oxygen involved in the CH4 oxidation was largely derived from the surface lattice oxygen of the Mn1, in accordance with the MvK mechanism. The O2 on–off profiles further demonstrate reaction mechanism, in which the desorbed CO2 was generated by the reaction between CH4 and lattice oxygen from Mn1 without O2, while the increase in CO2 resulted from the reaction between CH4 and the constantly refilled oxygen at the vacancies in the presence of O2 (Jia et al., 2019).
Wang et al. studied the roles of the crystallographic structure of Mn-based oxides, among which α-MnO2 exhibited the superior catalytic performances, attributing to higher surface Mn concentration and more active oxygen species, more mono-μ-oxo bridged (corner-shared) MnO6 sites, and better reducibility. CMC over the MnO2 catalyst proceeds via both MvK and L–H mechanisms, while the latter was predominant, in which the dehydrogenation of CH* to H* was considered as the rate-determining step and intermediate species -COO and -CH3O were oxidized by active oxygen species to CO2 and H2O (Wang et al., 2018).
3.4 Cr2O3-based catalysts
3.4.1 Single Cr2O3
The Cr2O3, trigonal crystal system belongs to the α-Al2O3 structure, which is composed of oxygen ions as the closest hexagonal packing and Cr3+ filling the octahedral gap formed by these close packings. The coordination number of Cr3+ is 6, and only 2/3 of the octahedral gap is occupied by chromium ions. The activity and selectivity of Cr2O3 are closely related to the change of the valence state of chromium ions. Various techniques have been developed to prepare Cr2O3 nanoparticles such as co-precipitation, incipient wetness impregnation, sol–gel, solvothermal, solid thermal decomposition, and sonication (El-Sheikh et al., 2009).
Jodłowski et al. applied the sonication method to prepare transitional metal oxide catalysts (Co, Cu, Cr), among which the chromium oxide catalyst showed greater catalytic activity as compared to cobalt oxide, copper oxide, and commercial palladium catalysts, attributing to the presence of a high concentration of formate on the surface of chromium oxide (Jodłowski et al., 2016).
3.4.2 Doped Cr2O3
The doping of Cr2O3 enables the catalyst to exhibit excellent catalytic activity due to the synergistic effect between TMOs. Yuan et al. prepared Cr-based catalysts modified by Ce via incipient wetness impregnation method and investigated the effects of the Ce loading amount. It was found that the Cr-based catalysts with 3 wt% Ce showed higher catalytic performance due to an increasing amount of reaction site [CrO4] species, improving the high-temperature-resistant performance and obtaining the synergistic effect between Ce and Cr (Yuan et al., 2013). Dupont et al. synthesized Cu/Cr oxides with high specific surface area by the sol–gel route using propionic acid. The results suggested that Cu/Cr oxides prepared by the special sol–gel process showed better catalytic performances compared with Cu/Cr oxide commercial catalysts, attributing to well-dispersed Cu species and the surface enrichment with Cr6+. In addition, though DFT coupled with periodic slab models, the authors believed that O2 molecules dissociatively adsorbed over the Cu/Cr oxide surface at the first step, along with generating the active oxygen species mainly at the Cr sites (Dupont et al., 2010).
3.4.3 Deactivation
Due to its poor affinity for acid gases, Cr2O3 exhibits excellent resistance to sulfur poisoning. Ordo´n˜ez et al. prepared different bulk metal oxides (Cr2O3, Co3O4, Mn2O3, NiO, and CuO) and investigated their catalytic stability for CMC in the presence of SO2. It was found that Cr2O3 exhibited highly sulfur-tolerant, whereas the other materials were deactivated rapidly, even if Co3O4 and Mn2O3 were more active than Cr2O3 for CMC in the absence of sulfur species. Characterization results illustrated that the excellent stability was caused by the low affinity of Cr2O3 to acid gases (as SO2); thus, sulfates would not form on the surface of the catalyst to occupy the active sites (Ordóñez et al., 2008).
3.4.4 Mechanisms
There is still controversy about the mechanism of CMC on Cr2O3. Huang et al. synthesized a homogeneous ZnCr2O4 oxides by the ethylene glycol-mediated solvothermal method. Cr3+ and Cr6+ coexisted in ZnCr2O4, in which Cr6+ probably caused the presence of interstitial oxygen species in the structure. The authors believed that the reaction pathway of CMC over the ZnCr2O4 catalyst was consistent with the L–H mechanism under low temperature, in which the interstitial oxygen was involved to the methane combustion. However, the mechanism has not been experimentally verified (Huang et al., 2019).
On the contrary, Zhu et al. applied the co-current co-precipitation method to prepare a series of Sn–Cr binary oxide catalysts among which the oxide with a Cr/Sn atomic ratio of 3 : 7 showed excellent catalytic activity due to higher surface areas and high oxidation states of chromium ions. Temperature-programmed 18O isotope-exchange measurements confirmed that CMC over Sn–Cr binary oxide catalysts occurred via a redox cycle with the chromium ion as the active center, following the MvK mechanism (Zhu et al., 2003).
3.5 Fe2O3-based catalysts
3.5.1 Single Fe2O3
Fe2O3 is an earth-abundant and non-toxic material that has been extensively used to catalyze a wide range of reactions including water gas shift reaction, photocatalytic water splitting, looping combustion, etc. There are four polymorphs of Fe2O3, which are Hematite (α-Fe2O3), Maghemite (γ-Fe2O3), β-Fe2O3, and ε-Fe2O3. Among which α-Fe2O3 is thermodynamically stable and has the corundum(α-Al2O3) structure, which is based on a hcp anion packing. γ-Fe2O3 is an inverse spinel structure with cation-deficient sites and can be transformed from α-Fe2O3 at high temperature. β-Fe2O3 andε-Fe2O3 have been synthesized only in the laboratory. The former has been obtained by the de-hydroxylation of β-FOOH under high vacuum at 170°C. The structure of ε-Fe2O3 is intermediate between those of α-Fe2O3 and γ-Fe2O3. It can be prepared in various ways and transforms to α-Fe2O3 at between 500 and 750°C, apparently according to the method of preparation (Cornell and Schwertmann, 2003).
Among the few researches dealing with the use of Fe2O3 in CMC, α-Fe2O3 was the dominant catalytic active phase, and preparation method affects its catalytic performance greatly. Barbosa et al. synthesized bulk α-Fe2O3 by precipitation (α-Fe2O3-p) and the citrate method (α-Fe2O3-c), finding that the preparation method strongly influences both the initial activity of the catalyst and its stability under reaction conditions (Barbosa et al., 2001). The α-Fe2O3-p catalyst presented higher surface areas, in correlation with greater initial activity and lower light-off temperatures than that of the α-Fe2O3-c catalyst. Although all catalysts undergo sintering at the high operation temperature with the loss of active sites, the α-Fe2O3-p catalyst exhibited less sintering and better stability. On this basis, Paredes et al. prepared α-Fe2O3 base catalysts via the acid dissolution-alkaline precipitation method using red mud as a raw material, an aluminum industrial waste formed by α-Fe2O3, Ti, Al, Ca, and Na, and compared the CMC performance and reaction mechanism with unprocessed red mud, and massive α-Fe2O3 synthesized by the precipitation method (α-Fe2O3-c mentioned above) (Paredes et al., 2004). For the combustion of 2000 ppm V CH4 in air, the α-Fe2O3-c exhibits the best activity (T50 = 461°C), and the activity of processed red mud (T50 = 530°C) is much higher than that of unprocessed red mud (T50>650°C) because after treatment the component of Na and Ca, which can hinder the catalyst activity decreases. Moreover, when conversion per Fe content in the catalyst is considered, the difference between α-Fe2O3-c and processed red mud catalysts is much smaller at low conversions. This would indicate that the other constituents of red mud have little or no effect on the catalyst activity. As for the mechanism, the CH4-TPD experiments (100–600°C) suggested that very little CH4 is adsorbed, the reticular oxygen of the catalyst being enough for its complete oxidation.
In addition, researchers have achieved the catalytic conversion of CH4 over α-Fe2O3 catalysts with different morphologies. Dong et al. fabricated 3D urchin-like mesoporous α-Fe2O3 nanoarchitectures with the combination of nonhomogeneous ionic liquid/diphenyl ether solvothermal method and solid-state thermal annealing. They found that CH4 was converted into products containing C–O bonds (CO2) at 230°C, which is 190°C lower than over bulk α-Fe2O3. As for the reason, with the measurement and comparison of adsorbed oxygen on the two material, they inferred that the urchin-like α-Fe2O3 nanoarchitectures have a higher density of surface oxygen vacancies than bulk α-Fe2O3, which can accelerate the dissociation of oxygen molecules at the surface and increase the mobility of lattice oxygen (Dong et al., 2014). It should be noted that this structure does not exhibit good CH4 conversion at high temperatures (700°C, conversion = 16%). He et al. invented a hard-templating synthetic strategy to guide the anisotropic growth of ultrathin α-Fe2O3 nanosheets with a large (110) facet exposure ratio, the catalytic performance of which in low-temperature CH4 combustion is comparable to that of noble metal-based catalysts (He L. et al., 2020). The antiferromagnetic coupled diiron core on the (110) crystallographic plane of α-Fe2O3 is a structurally favorable condition, which resembles the diiron active site in soluble CH4 monooxygenase, an enzyme that converts CH4 to methanol in nature (Ross and Rosenzweig, 2017). Meanwhile, they utilized oxygen isotopic tracing experiments and DFT calculations to confirm that CH4 is primarily activated by lattice oxygen below 400°C, in accordance with the MvK mechanism. They further speculated that at higher temperatures, CH4 would be activated predominantly by molecular oxygen instead, as the accumulation of lattice oxygen vacancy favoring the adsorption of molecular oxygen from the gas phase (Cheng et al., 2016).
3.5.2 Deactivation
CH4 emissions are often accompanied by large quantities of steam and traces of sulfur-containing gases, which are the two most performance hindering species present in typical CH4 after-treatment operating conditions (Raj, 2016). Setiawan et al. confirm that α-Fe2O3 catalysts are significantly lower activity under the mixture conditions of water vapor and CH4 due to the strongly bound between water (hydroxyl) species and Fe2O3, which destruct the active sites irreversibly (Setiawan et al., 2015). Recently, García-Vázquez et al. synthesized iron- and chromium-based oxides by using the citrate sol–gel method and investigated their catalyst performance of CMC in the presence of SO2 and steam. They found that catalyst Fe60Cr40 (molar ration) exhibited the remarkable performance (366 ppm CH4 balanced in air, T = 450°C, conversion = 79%) due to the formation of the FeCr2O4 spinel phase, which is the most active sites in these catalyst for CMC. And because the FeCr2O4 spinel phase can still exits after being aged under wet conditions, the Fe60Cr40 showed the better catalyst conversion of CH4 than α-Fe2O3 catalyst, which can also be found by comparing Figures 9A,B (312 ppm CH4 + 4.6 ppm SO2 10% vol H2O balanced in air, T = 450°C, conversion = 47% vs. 26%). In addition, as has been represented in Figure 9C, the competitive adsorption of hydroxide groups on FeCr2O4 even slows down the rate at which sulfur dioxide poisons the catalyst’s active sites (García-Vázquez et al., 2020).
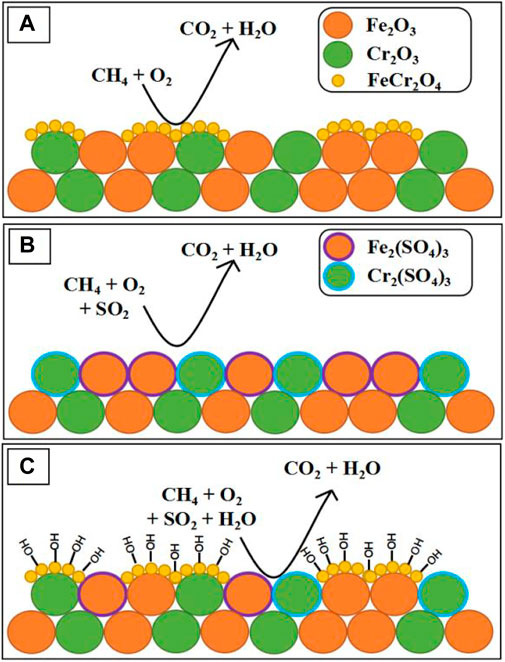
FIGURE 9. Proposed poisoning mechanisms at 450°C under (A) sulfur-free conditions, (B) dry conditions, and (C) wet conditions. Cited from García-Vázquez et al. (2020).
3.5.3 Mechanisms
In conclusion, the reaction of α-Fe2O3 catalyst in CMC involves the MvK mechanism. Recently, Tang et al. adopted the generalized gradient approximation (GGA) + U approach to investigate the reaction pathways of complete and partial oxidations of CH4 on the dominant Fe–O3–Fe termination of thermodynamically stable hematite (α-Fe2O3) (0001) facets. The energy barrier for the first C–H bond activation is 1.04 eV. In the transition state, the dissociating H is in contact with the lattice O and with the dissociating CH3 is in contact with the Fe site. Subsequent decomposition and oxidation of the CHx species (x = 1, 2, 3) exploit the lattice O species according to the MvK mechanism, forming CHxO in more thermodynamically and kinetically favorable pathways. For the two pathways, the overall rate-limiting steps are both the first C–H bond activation. Adsorption of O2 on the VO site is exothermic (-1.42 eV), with one O atom binding at the VO site and the other O binding on the Fe site. In particular, after the dissociation of O2 via the O–O bond cleavage, one O fills the oxygen vacancy, and the other O became the ferryl O (Fe=O). Although the ferryl O is highly active and capable of lowering the energy barrier of the C–H bond activation, the availability of extremely active ferryl O is expected to be too low on the Fe–O3–Fe-terminated surface to critically impact the overall catalyst performance (Tang and Liu, 2016).
3.6CeO2-based catalysts
3.6.1 Single CeO2
CeO2 is the most stable form of cerium oxide, which has a face-centered cubic structure and space group of Fm-3m (Trovarell, 2002). The coordination of cerium is 8 and that of oxygen is 4, which means that there are large vacant octahedral holes in the structure. Because of its structure, CeO2 has an excellent storage-release capacity in a reversible manner. Specifically, Ce4+ can be reduced to Ce3+ under anoxic conditions, accompanied by a rapid release of lattice oxygen from the solid to the gas phase, leaving oxygen vacancy defects. On the other hand, Ce3+ can be re-oxidized into Ce4+ by adsorbing oxygen under oxygen-rich conditions and therefore refills the vacancies. Such unique redox properties render CeO2 a wonderful support for noble metal catalysts in the catalytic oxidation of hydrocarbons, following mostly the MvK mechanism (Aneggi et al., 2016).
3.6.2 Doped CeO2
CeO2 has been actively studied in the past few decades as an alternative to noble metal catalysts for CMC (Mukherjee et al., 2016). While pure CeO2 phase exhibits a poor catalytic activity at low temperatures and a poor thermal stability at high temperatures, which significantly hampers its industrial uses (Kašpar et al., 1999). Efforts have been made to improve the catalytic CH4 combustion performance of CeO2-based catalysts such as compositing with other metal oxides, heteroatom doping, etc. For instance, more oxygen vacancies can be introduced into the CeO2 structure by doping cations with oxidation states below or equivalent to 4+ such as Ca2+, Mn2+, Ni2+, Cu2+, Fe3+, Co3+, La3+, and Y3+ (Palmqvist et al., 1998; Huang et al., 2012; Wu et al., 2015; Zedan and AlJaber, 2019). The presence of abundant oxygen vacancies promotes the mobility of lattice oxygen and subsequently boosts the catalytic activity of CeO2. Meanwhile, there may also exist a synergistic effect between the doped metals and Ce. Palella et al. recently prepared MnCeOx composites via a redox route and demonstrated that Mn2+ ions are the active sites for the formation of active oxygen species arising from the oxygen vacancies in low-temperature oxidation of CH4, while Ce elements can improve the availability of reactant molecules at the active site by introducing oxygen vacancies (Palella et al., 2021). There is a strong synergistic effect between Mn–Ce elements, which stabilizes Mn2+ species and facilitates the dispersion of Mn2+ ions. The dissociation of CH4 into CHn* and H* species was found to be the rate-determining step for the Mn-doped CeO2 composite system. Apart from Mn, other monometallic elements such as Cu (T50 = 350°C), Fe (T50 = 378°C), Ni (T50 = 415°C), and Co (T50 = 380°C) catalysts have also improved catalytic activities and stabilities due to the established synergistic effects that increase the dispersion of active metal oxides, which allow more Mn+/M(n−1)+ redox couples to participate in the redox cycle; more discussions on the doped CeO2 catalysts can be found in a recent review by Stoian et al. in details (Stoian et al., 2021). Due to the absence of active site, which triggered by grain growth, CeO2 is sintering at high temperature. Larrondo et al. tried to increase the thermal stability of CeO2 by the addition of ZrO2 to the structure of CeO2 and found that ZrO2 can also slightly facilitate the reducibility of the solids associated with both surface and bulk Ce sites with the evidence of H2 TPR. And the catalysts with 10% of Zr have higher values of CH4 conversion than the other samples with 30% Zr and 50% Zr (Larrondo et al., 2005). Recently, Toscania et al. used the citrate complexation route to future prepared Ce0.9ScxZr0.1−xO2−δ (x = 0, 0.02, 0.04, and 0.06) by citrate complexation route, with the aim of combining the improved thermal stability provided by the ZrO2 with an increase in vacancy concentration upon Sc doping (Toscani et al., 2019). The doped samples exhibited superior redox behavior because the CeO2 reduction values from TPR experiments and vacancy concentration from Raman tests both increase with increasing Sc content. And in contrast to the binary CeO2–ZrO2 sample, the CeO2–ZrO2–Sc2O3 showed the higher reaction rates and lower apparent activation energies for CMC. In addition, in situ XANES experiments confirm the participation of the lattice in the redox mechanism. Huang et al. prepared a series of NiO/CeO2 by a facile impregnation method, which exhibited high catalytic performance and stability due to synergistic interaction between CeO2 and NiO. The incorporation of Ni2+ into the CeO2 lattice obviously enhanced the concentration of oxygen vacancies and amount of surface oxygen, making the mobility of bulk oxygen in CeO2 increased, along with the reduction of the activation energy of the CMC. On the other hand, CeO2 prevented the aggregation of NiO, further improving the reduction properties of NiO (Huang et al., 2020). Apart from this, CeO2-modified catalytic materials can also be used in the reaction of oxidative coupling of methane (OCM) because of its above redox properties and increased surface basicity (Siakavelas et al., 2021). OCM is an exothermic reaction between CH4 and O2 in the range of 700–900°C, forming C2 hydrocarbons (e.g., C2H4 or C2H6), via CH4–CH3–C2H6–C2H4 progress. Moreover, for OCM, the incorporation of f-block elements such as Pr3+, Sm3+, and La3+ (redox-active basic ions) into the CeO2 could modify the acid–base properties, enhance its thermal stability, and generate additional oxygen vacancy sites (Xu et al., 2019; Zhang et al., 2020; Siakavelas et al., 2022a). Furthermore, Siakavelas et al. (2022b) recently added lithium ions into CeO2- and CeO2-modified materials (Sm-Ce and La-Sm-Ce metal oxides), using the wet impregnation technique. They argued that the addition of lithium species changed the reaction pathway and drastically enhanced the production of ethylene and ethane, mainly for the promoted catalysts (Li/Sm-Ce and Li/La-Sm-Ce).
3.6.3 Binary CeO2 catalysts
The CMC involves both the activation of C–H of CH4 and O–O of O2. CeO2 can activate molecular oxygen via Ce4+ and Ce3+ redox couples; however, it is incapable of activating the C–H of CH4. On the contrary, NiO exhibits high activity in activating C–H, but, does not activate molecular oxygen. Thus, Zhang et al. synthesized NiO/CeO2 through a two-step method, in which nanocomposite consists of CeO2 nanorods with supported NiO nanoclusters, exhibiting notably higher activity due to the lowest apparent activation energy (69.4 ± 4 kJ/mol). The schematic diagram of the reaction mechanism at the interface is shown in Figure 10. The C–H of CH4 was activated on the Ni-O species, forming a H3C–Ni- intermediate on the interface. The formed CH3 could be further activated to form CH2 or even CH species, which could couple with surface lattice oxygen atoms to form CO2 and H2. The process follows the MvK mechanism, in which NiO nanoclusters and CeO2 nanorods show a synergistic effect for CMC (Zhang et al., 2018).
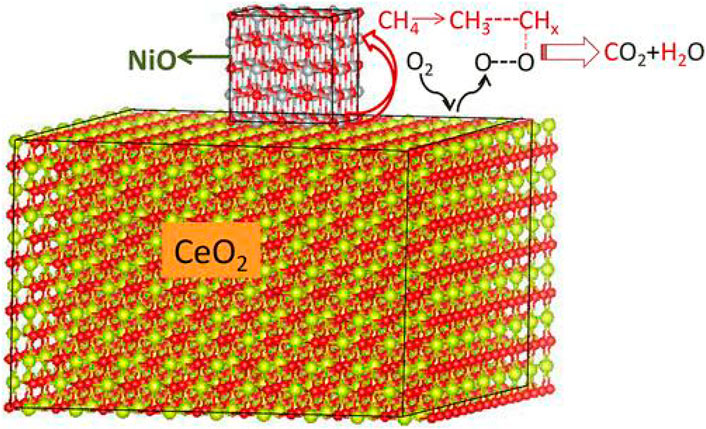
FIGURE 10. Schematic briefly showing activations of C–H of CH4 and O−O of O2 and coupling between CHx species and atomic oxygen at the interface of NiO/CeO2. Reprinted with permission from Zhang et al. (2018). Copyright (2018) American Chemical Society.
3.6.4 Mechanisms
In conclusion, the mechanism of CeO2 in CMC has been reported to follow the MvK mechanism (Knapp and Ziegler, 2008). Results reported in the literature indicate that the first step is hydrogen abstraction from the CH4 molecule over the Ce surface. As the (111) surface of CeO2 is the most stable and predominant, therefore, the exposed oxygen atoms with low coordination on the (111) surface are assumed as the active sites over CeO2-based catalysts in CMC. CH4 dissociation is followed by the formation of a CH3 radical and an adsorbed H* atom. These two species bind to two surface oxygen atoms, leading to the formation of a methyl radical (CH3−) and a hydroxyl radical (HO·). Hydrogen adsorption leads to the reduction of one of the neighboring Ce atoms. Afterward, a series of intermediate steps take place, in which the H atoms are abstracted from CH3* to form CH2* and CH* until CO is formed by further reducing the Ce with the formation of an oxygen vacancy. Finally, the adsorbed CO reduces Ce by binding with another oxygen allowing the formation of CO2. Water is formed by the binding of adjacent OH groups. The breaking of the C–H bond is generally the rate-limiting step in all methane activation processes (Tang et al., 2010).
3.7 CuO-based catalysts
3.7.1 Single CuO
CuO is an important p-type semiconducting material with a cubic rock salt structure (c-CuO) and lower-symmetry monoclinic structure (m-CuO). Analysis of the calculated band structures revealed that c-CuO is an indirect gap semiconductor, while m-CuO has metallic behavior (Cao et al., 2018). CuO has been considered as one of the most effective alternatives to noble metal-based catalysts in CMC because of its Earth abundance, non-toxicity, and good catalytic performance (Liu et al., 2010).
Researchers exhibited that Cu loading and the nature of the carrier exert influence on the Cu species present on the catalyst surface. Park and Ledford tested the catalytic activity of Cu/Al2O3 catalysts with different Cu loadings for CH4 combustion reactions and they found that, with increasing Cu content, both the activity per unit mass of Cu and per mole of Cu on the surface decreased (Park and Ledford, 1998). The active phase for CH4 oxidation is a superficial phase formed by isolated or highly dispersed Cu; as the Cu content increases, the dispersion becomes worse and therefore reduces the total number of active sites. Aguila et al. investigated CuO-loaded catalysts with porous media such as Al2O3, ZrO2, and SiO2 prepared by the impregnation method (Aguila et al., 2008). This work showed that CuO catalysts supported on ZrO2 have higher activity (per unit mass of Cu) for CH4 oxidation than when CuO is supported on alumina or silica, which is related to the ability of ZrO2, stabilizing the highly dispersed Cu species to prevent the formation of bulk CuO. This ability is available when Cu concentrations between 0.25% and 6% for the catalysts are supported on ZrO2. In addition, they also tested the influence of water for the CuO catalysts supported on ZrO2. The addition of water produces a decrease of the CH4 conversion, but, as soon as the water flow is stopped, the catalyst recovers its initial activity, which means that inhibition with water is reversible, at least for the 300 min considered in this experiment.
In addition to dispersion, the acid–base properties of the supports also affect the catalytic performance of Cu-based catalysts. Theoretically, the adsorption and activation of hydrocarbons on oxide-based catalysts and the desorption of reaction products are related not only to the strength and distribution of the Lewis acidic metal cation sites but also to the concentration of lattice oxygen anions as the Lewis base sites (Vedrine et al., 1996). Specifically, the interaction of CH4 with acid–base pairs on the catalyst surface leads to the heterolytic breakage of the C–H bond and the formation of CH3− and H+ species chemisorbed on the acid and base sites, respectively. Stronger acidic sites enhance the interaction with the carbon anion and therefore facilitate surface catalytic combustion (Choudhary and Rane, 1991). By co-precipitation and calcination methods, Popescu et al. synthesized the CuO nanoparticles supported on mixed oxides of Al2O3, MgO, and Mg(Al)O and investigated their catalytic properties in the total oxidation of CH4 (Popescu et al., 2017). Because, on the one hand, CH4 activation involving the heterolytic C–H bond breaking needs acid–base pairs, on the other hand, the total oxidation reaction is favored in the presence of acid sites of high strengths, which strongly adsorb carbanions, thus undergoing surface reaction with oxygen. The CuMgAl(1)O (Mg/Al atomic ratios is 1) showed the highest activity as it not only contained strong acid sites (ca.50%), which was similar with the CuAlO (strong acid species), but also had strong basic sites (ca. 30%).
3.7.2 Doped CuO
Lu et al. prepared CuO–CeO2 hybrid nanoparticle and created substantial amounts of Cu–Ce–O interfaces by gas-phase evaporation-induced self-assembly. CuO–CeO2 exhibited excellent catalytic performances with a low light-off temperature, high activity, selectivity, and operation stability. The two possible mechanisms of CMC over CuO and CuO–CeO2 are shown in Figure 11. The authors conjectured that two routes follow the MvK mechanism. However, the Cu–Ce–O interfacial metal–support interaction promotes the redox cycle of interface, in which CH4 binds to the surface of CuO, while O2 is simultaneously adsorbed on the oxygen vacancy of CeO2, then dissociating to a surface-bound methyl group and oxygen atoms, respectively; after the release of H2O, an oxygen vacancy regenerates, subsequently combining with the oxygen atoms; the dissociated methyl group is further oxidized by the adsorbed oxygen atoms, and then CO2 is released from the surface of CuO. The synergistic effect between CuO and CeO2 increases the rate of CMC, which has shown promise in enhancing the removal rate of hydrocarbon from the catalyst surface (Lu et al., 2016).
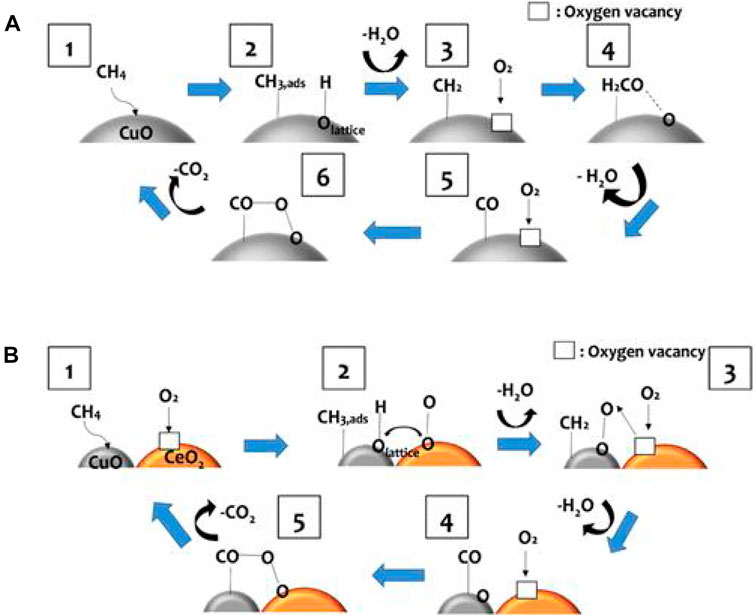
FIGURE 11. Cartoon depiction of methane combustion catalyzed by CuO (A) and Cu/CeO2 (B). Reprinted with permission from Lu et al. (2016). Copyright (2016) American Chemical Society.
3.7.3 Mechanisms
Kong et al. identified CuO (001) as an active surface for CMC over CuO among other surfaces, including (110), (111), (101), (010), and (011) via DFT calculations (Figure 12). It is not surprising because (001) is strongly polarized and shows high reactivity due to the high ratio of lowly coordinated oxygen. In DFT calculations, CH4 is firstly adsorbed with AE = -0.86 eV, followed by a spontaneous dissociation with CH3 and H adsorbed on surface oxygen, as depicted in Figures 12A–C. Surface oxygen is often actively involved and may cleave H from CHx to form -OH and H2O, generating an oxygen vacancy (OV), which can be filled with reactant O2, leading to its dissociation and further oxidizing CHx intermediates, as shown in Figures 12D–F. With oxygen transferring to bond with carbon, the hydrogen in CHx can shift to surface oxygen again with an energy release of 3.66 and 1.46 eV, indicating that such a shift is highly favorable, as shown in Figures 12G–I. Again, an OV is generated and refilled by O2 when H2O is released, and similarly O2 is dissociated to release atomic oxygen after exceeding a small barrier of 0.47 eV, which can oxidize adsorbed CO to form CO2, as outlined in Figures 12I–L. Although the authors did not specify which mechanism the mechanism of CMC over CuO (001) conforms to, given their theoretical calculations, we believe that it is consistent with the MvK mechanism (Kong et al., 2018).
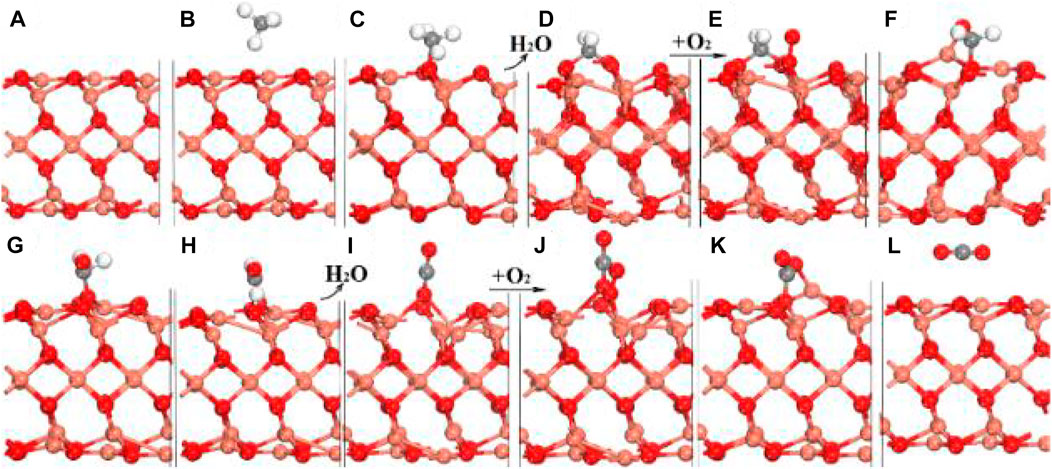
FIGURE 12. (A) Clean (001); (B) CH4 physical adsorption; (C) CH4 dissociative adsorption; (D) CH2* with oxygen vacancy (OV) presented; (E) CH2* with O2 adsorbed; (F) CH2* rotating and interacting with O2*; (G) transition state (TS) state COH2*; (H) CHO* + H*; (I) CO* with OV presented after releasing one H2O; (J) CO* with O2 adsorbed; (K) TS for O transfer to CO*; (L) CO2 physical adsorbed. Cu, O, C, and H are shown as rose-carmine, red, grey and white spheres, respectively. Reprinted with permission from Kong et al. (2018). Copyright (2018) Beilstein-Institut.
4 Perspectives
It has been well acknowledged that TMOs are one of the most promising candidates as alternatives to noble metal catalysts in CMC. Although promises have seen in some TMOs, problems still exist such as the controversial reaction mechanism, low water vapor, and sulfur resistance. Therefore, it is necessary to explore appropriate preparation methods, suitable modified ions, and the optimal ratios to synthesize novel TMOs with excellent resistance to water vapor and sulfur poisoning, guided by the reaction mechanism (Lin et al., 2017; Chen et al., 2022).
Some TMOs with significant water resistance, such as NiO, should be further studied to enhance sulfur resistance. Cr2O3 with excellent sulfur resistance can be selected to prepare binary complexes to enhance its catalytic stability (Ordóñez et al., 2008). Although α-Fe2O3 catalysts, especially with two-dimensional nanostructure, have been proven to have excellent catalytic performance in CMC, their performance is significantly reduced in the presence of steam and SO2. Herein, we can further investigate Fe–Cr mixed catalysts with the emphasis on the creation of spinel structures to increase the sulfur and water resistance of iron-based catalysts (García-Vázquez et al., 2020).
In addition, beyond complete oxidation, TMOs also find great uses in CH4 partial oxidation reactions, such as direct oxidation to methanol, which is known as the “holy grail” in catalysis community to convert CH4 into value-added chemicals with a much reduced cost in reaction steps and separation as compared to the conventional industrial route. (Sushkevich et al., 2017). The TMOs reveal the enormous potentials in the direct oxidation of CH4 to CH3OH owing to the relatively strong ability to activate the C–H bond and avoid CH4 overoxidation.
In nature, CH4 monooxygenase can directly convert CH4 to CH3OH using H2O, O2, and CO2 as reactants at an ambient temperature (Sun et al., 2021). However, such biomimetic strategies are often limited by industrial-scale reactions. Some TMOs can dissociate CH4 at room temperature, which offers the possibility for the direct conversion of CH4 (Huang et al., 2021). Zuo et al. found that an inverse CeO2/Cu2O/Cu (111) catalyst is able to bind and dissociate CH4 at room temperature by mimicking the function of the CH4 monooxygenase. The catalytic system produced only adsorbed CHx fragments in the presence of H2O, along with a high transformation from CH4 to CH3OH. The dissociation of H2O formed OH groups, which occupied the catalyst surface. OH groups removed sites decomposing CHx fragments, generating centers with special electronic properties. On the special active centers, CH4 could directly interact to yield CH3OH (Zuo et al., 2016). Liu et al. carefully studied key roles of H2O for the conversion of CH4 directly into CH3OH on CeO2/Cu2O/Cu (111). H2O preferentially dissociated over the active Ce sites at the CeO2/Cu2O/Cu (111) interface, hindering O2 activation and the overoxidation of CH4. H2O produced active *OH to promote the direct conversion of CH4. O2 dominantly reoxidized the reduced CeOx, and water adsorption also displaced the produced methanol into the gas phase (Liu et al., 2020).
Some researchers have explored bimetallic oxides with dual roles, in which one oxide activates CH4 and the other ensures high selectivity to methanol. Yang et al. synthesized highly mixed hybrid IrO2/CuO via a bottom-up tactic, which exhibited excellent catalytic performance with a methanol yield of 1937 μmol gcat−1 and a methanol selectivity of about 95% through the synergistic effect of IrO2 for CH4 activation and CuO for selective oxidation. In the oxidation process of methane, due to the strong electrophilic property of Ir4+, IrO2 could facilitate the C–H bond cleavage by forming Ir-C σ bond. Then, -CH3 attached by Ir bound to the neighboring Cu-attached O to form -OCH3, subsequently extracted by H2O to accelerate the formation of methanol. At last, the formed O vacancy is replenished by O2 (Yang et al., 2019).
It deserves to explore novel metal oxides with low metal-O bond strength and satisfactory methanol selectivity for facilitating the surface methoxy group formation. The metal oxides can also be employed as a cocatalyst being strong electrophilic metal oxides with extraordinary capacity to promote the C–H bond cleavage of CH4 (Fuller et al., 2016). The synergistic effect of bimetallic oxides offers an alternative route for the design and synthesis of novel catalysts for the direct conversion of CH4 into methanol (Lyu et al., 2021). In addition, the mechanism by which H2O promotes the high selectivity of direct CH4 conversion also needs to be continuously explored.
5 Conclusion
As an important greenhouse gas, the lean emission of CH4 causes a huge environmental crisis. In this article, all the described results certify that both single and binary TMOs show the great potential of being promising alternatives to the expensive noble metal catalysts. Reviewed novel TMO catalysts exhibit appreciable catalytic reactivity associated with preparation methods, structures, morphologies, exposed crystal planes, crystal defects, oxygen vacancies, doping, and supporting. It can be seen that the structure, morphology, and exposed crystal planes determined by the preparation method significantly influence their catalytic activity for the CMC through the variation in morphology, surface area, and surface or lattice defects. In addition, mixed TMO catalysts prepared by doping and supporting exhibit excellent catalytic performance compared with the corresponding single TMOs due to synergistic interactions between the different TMO species. In general, the deactivation of TMO catalysts due to water vapor poisoning is reversible, while the deactivation due to sulfur poisoning is irreversible. The doping and supporting will improve the stability of TMO catalysts. The reaction mechanism of CMC over TMO catalysts is still controversial. Among the discussed possibilities, the MvK mechanism involves the oxidation of methane only by lattice oxygen with molecular oxygen replenishing the lattice oxygen after its consumption, while the L–H and E–R mechanisms only involve surface-adsorbed oxygen from molecular oxygen in the gas phase as the oxidizing power for methane activation. In addition, the T-T mechanism is also proposed for reactions involving both surface-adsorbed oxygen and lattice oxygen. The dominance of surface-adsorbed oxygen and lattice oxygen may change with the catalyst structure and temperature. As the characteristic techniques are under progressive development, it is expected to reveal the precise reaction mechanism of CMC over different TMO catalytic systems and therefore the identification of the true active site structures.
Author contributions
YG and MJ drafted the article, conceived the concept of the review, conducted literature survey, and arranged the figures. LY, ZL, FT, and YH revised the article and provided comments. All authors contributed to the article and approved the submitted version.
Funding
This project was financially supported by the Shanghai Post-Doctoral Excellence Program, 2021232, and the UM-SJTU Joint Institute and Shanghai Science and Technology Development Funds of “Rising Star” Sailing Program (22YF1419400).
Conflict of interest
The authors declare that the research was conducted in the absence of any commercial or financial relationships that could be construed as a potential conflict of interest.
Publisher’s note
All claims expressed in this article are solely those of the authors and do not necessarily represent those of their affiliated organizations, or those of the publisher, the editors, and the reviewers. Any product that may be evaluated in this article, or claim that may be made by its manufacturer, is not guaranteed or endorsed by the publisher.
References
Aguila, G., Gracia, F., Cortés, J., and Araya, P. (2008). Effect of copper species and the presence of reaction products on the activity of methane oxidation on supported CuO catalysts. Appl. Catal. B Environ. 77 (3-4), 325–338. doi:10.1016/j.apcatb.2007.08.002
Akbari, E., Alavi, S. M., Rezaei, M., and Larimi, A. (2021). Catalytic methane combustion on the hydrothermally synthesized MnO2 nanowire catalysts. Ind. Eng. Chem. Res. 60, 7572–7587. doi:10.1021/acs.iecr.1c00881
Aneggi, E., Boaro, M., Colussi, S., de Leitenburg, C., and Trovarelli, A. (2016). Ceria-based materials in catalysis: Historical perspective and future trends. Handb. Phys. Chem. Rare Earths 50, 209–242. doi:10.1016/bs.hpcre.2016.05.002
Arena, F., Di Chio, R., Fazio, B., Espro, C., Spiccia, L., Palella, A., et al. (2017). Probing the functionality of nanostructured MnCeOx catalysts in the carbon monoxide oxidation. Appl. Catal. B Environ. 210, 14–22. doi:10.1016/j.apcatb.2017.03.049
Barbosa, A. L., Herguido, J., and Santamaria, J. (2001). Methane combustion over unsupported iron oxide catalysts. Catal. Today 64 (1-2), 43–50. doi:10.1016/S0920-5861(00)00507-1
Becker, E., Carlsson, P. A., Kylhammar, L., Newton, M. A., and Skoglundh, M. (2011). In situ spectroscopic investigation of low-temperature oxidation of methane over alumina-supported platinum during periodic operation. J. Phys. Chem. C 115, 944–951. doi:10.1021/jp103609n
Belessi, V. C., Ladavos, A. K., Armatas, G. S., and Pomonis, P. J. (2001). Kinetics of methane oxidation over La-Sr-Ce-Fe-O mixed oxide solids. Phys. Chem. Chem. Phys. 3 (17), 3856–3862. doi:10.1039/b103426j
Buchneva, O., Gallo, A., and Rossetti, I. (2012). Effect of nitrogen-containing impurities on the activity of perovskitic catalysts for the catalytic combustion of methane. Inorg. Chem. 51 (21), 11680–11687. doi:10.1021/ic3015892
Cao, H., Zhou, Z., Yu, J., and Zhou, X. (2018). DFT study on structural, electronic, and optical properties of cubic and monoclinic CuO. J. Comput. Electron. 17 (1), 21–28. doi:10.1007/s10825-017-1057-9
Chen, J. H., Arandiyan, H., Gao, X., and Li, J. H. (2015). Recent advances in catalysts for methane combustion. Catal. Surv. Asia 19 (3), 140–171. doi:10.1007/s10563-015-9191-5
Chen, K., Li, W. Z., Li, X. Z., Ogunbiyi, A. T., and Yuan, L. (2021). Irregularly shaped NiO nanostructures for catalytic lean methane combustion. ACS Appl. Nano Mat. 4 (5), 5404–5412. doi:10.1021/acsanm.1c00732
Chen, Y. L., Lin, J., Chen, X. H., Fan, S. Q., and Zheng, Y. (2021). Engineering multicomponent metal-oxide units for efficient methane combustion over palladium-based catalysts. Catal. Sci. Technol. 11 (1), 152–161. doi:10.1039/d0cy01742f
Chen, Y., Yao, K., Zhang, X., Shen, B., Smith, R. L., Guo, H., et al. (2022). Siloxane-modified MnOx catalyst for oxidation of coal-related o-xylene in presence of water vapor. J. Hazard. Mat. 436, 129109. doi:10.1016/j.jhazmat.2022.129109
Chen, Z. P., Wang, S., Liu, W. G., Gao, X. H., Gao, D. N., Wang, M. Z., et al. (2016). Morphology-dependent performance of Co3O4via facile and controllable synthesis for methane combustion. Appl. Catal. A General 525, 94–102. doi:10.1016/j.apcata.2016.07.009
Cheng, Z., Qin, L., Guo, M., Xu, M., Fan, J. A., Fan, L. S., et al. (2016). Oxygen vacancy promoted methane partial oxidation over iron oxide oxygen carriers in the chemical looping process. Phys. Chem. Chem. Phys. 18 (47), 32418–32428. doi:10.1039/C6CP06264D
Choudhary, V., and Rane, V. H. (1991). Acidity/basicity of rare-earth oxides and their catalytic activity in oxidative coupling of methane to C2-hydrocarbons. J. Catal. 130 (2), 411–422. doi:10.1016/0021-9517(91)90124-M
Choya, A., de Rivas, B., González-Velasco, J. R., Gutiérrez-Ortiz, J. I., and López-Fonseca, R. (2018a). Oxidation of residual methane from VNG vehicles over Co3O4-based catalysts: Comparison among bulk, Al2O3-supported and Ce-doped catalysts. Appl. Catal. B Environ. 237, 844–854. doi:10.1016/j.apcatb.2018.06.050
Choya, A., de Rivas, B., Gutiérrez-Ortiz, J. I., and López-Fonseca, R. (2018b). Effect of residual Na+ on the combustion of methane over Co3O4 bulk catalysts prepared by precipitation. Catalysts 8 (10), 427. doi:10.3390/catal8100427
Choya, A., Gudyka, S., de Rivas, B., Gutiérrez-Ortiz, J. I., Kotarba, A., López-Fonseca, R., et al. (2021). Design, characterization and evaluation of Ce-modified cobalt catalysts supported on alpha alumina in the abatement of methane emissions from natural gas engines. Appl. Catal. A General 617, 118105. doi:10.1016/j.apcata.2021.118105
Choya, A., Rivas, B. D., Gutiérrez-Ortiz, J. I., and López-Fonseca, R. (2022). Bulk Co3O4 for methane oxidation: Effect of the synthesis route on physico-chemical properties and catalytic performance. Catalysts 12, 87. doi:10.3390/catal12010087
Cornell, R. M., and Schwertmann, U. (2003). The iron oxides: Structure, properties, reactions, occurrences, and uses, 2. Weinheim: Wiley VCH, 71. doi:10.1515/CORRREV.1997.15.3-4.533
Dong, B., Zhang, H., Kong, A., Kong, Y., Yang, F., Shan, Y., et al. (2014). Synthesis of urchin-Like FeF2 nanoarchitectures and their conversion into three-dimensional urchin-like mesoporous α-Fe2O3 nanoarchitectures for methane activation. Eur. J. Inorg. Chem. 28, 4779–4787. doi:10.1002/ejic.201402152
Dou, J., Tang, Y., Nie, L., Andolina, C. M., Zhang, X., House, S., et al. (2018). Complete oxidation of methane on Co3O4/CeO2 nanocomposite: A synergic effect. Catal. Today 311, 48–55. doi:10.1016/j.cattod.2017.12.027
Dupont, N., Kaddouri, A., and Gélin, P. (2010). Physicochemical and catalytic properties of sol gel-prepared copper-chromium oxides. J. Solgel. Sci. Technol. 58 (1), 302–306. doi:10.1007/s10971-010-2391-6
El-Sheikh, S. M., Mohamed, R. M., and Fouad, O. A. (2009). Synthesis and structure screening of nanostructured chromium oxide powders. J. Alloys Compd. 482 (1-2), 302–307. doi:10.1016/j.jallcom.2009.04.011
Fan, S. Q., Zhang, W., Xu, H., Cai, G. H., Zhan, Y. Y., Chen, X. H., et al. (2022). Tuning lattice defects to facilitate the catalytic performance of Ni-Cu-O hybrid nanoparticles towards methane oxidation. Int. J. Hydrogen Energy 47, 4536–4545. doi:10.1016/j.ijhydene.2021.11.060
Feng, Z. J., Du, C., Chen, Y. J., Lang, Y., Zhao, Y. K., Cho, K., et al. (2018). Improved durability of Co3O4 particles supported on SmMn2O5 for methane combustion. Catal. Sci. Technol. 8 (15), 3785–3794. doi:10.1039/c8cy00897c
Feyel, S., Döbler, J., Schröder, D., Sauer, J., and Schwarz, H. (2006). Thermal activation of methane by tetranuclear [V4O10]+. Angew. Chem. Int. Ed. 45 (28), 4681–4685. doi:10.1002/anie.200600188
Fu, G., Xu, X., and Wan, H. L. (2006). Mechanism of methane oxidation by transition metal oxides: A cluster model study. Catal. Today 117 (1-3), 133–137. doi:10.1016/j.cattod.2006.05.048
Fuller, J. T., Butler, S., Devarajan, D., Jacobs, A., Hashiguchi, B. G., Konnick, M. M., et al. (2016). Catalytic mechanism and efficiency of methane oxidation by Hg (II) in sulfuric acid and comparison to radical initiated conditions. ACS Catal. 6 (7), 4312–4322. doi:10.1021/acscatal.6b00226
García-Vázquez, M., Wang, K., González-Carballo, J. M., Brown, D., Landon, P., Tooze, R., et al. (2020). Iron and chromium-based oxides for residual methane abatement under realistic conditions: A study on sulfur dioxide poisoning and steam-induced inhibition. Appl. Catal. B Environ. 277, 119139. doi:10.1016/j.apcatb.2020.119139
Gong, D., and Zeng, G. F. (2021). Low-temperature combustion of methane over graphene templated Co3O4 defective-nanoplates. Sci. Rep. 11, 12604. doi:10.1038/s41598-021-92165-4
Gremminger, A., Lott, P., Merts, M., Casapu, M., Grunwaldt, J. D., Deutschmann, O., et al. (2017). Sulfur poisoning and regeneration of bimetallic Pd-Pt methane oxidation catalysts. Appl. Catal. B Environ. 218, 833–843. doi:10.1016/j.apcatb.2017.06.048
Han, Y. F., Chen, L. W., Ramesh, K., Widjaja, E., Chilukoti, S., Surjami, I. K., et al. (2008). Kinetic and spectroscopic study of methane combustion over α-Mn2O3 nanocrystal catalysts. J. Catal. 253 (2), 261–268. doi:10.1016/j.jcat.2007.11.010
Han, Z., Zhang, H. Q., Dong, B., Ni, Y. Y., Kong, A. G., Shan, Y. K., et al. (2016). High efficient mesoporous Co3O4 nanocatalysts for methane combustion at low temperature. ChemistrySelect 5, 979–983. doi:10.1002/slct.201600211
He, L., Fan, Y. L., Bellettre, J., Yue, J., and Luo, L. G. (2020). A review on catalytic methane combustion at low temperatures: Catalysts, mechanisms, reaction conditions and reactor designs. Renew. Sustain. Energy Rev. 119, 109589. doi:10.1016/j.rser.2019.109589
He, Y. L., Y. L., Guo, F. C., Yang, K. R., Heinlein, J. A., Bamonte, S. M., Fee, J. J., et al. (2020). In situ identification of reaction intermediates and mechanistic understandings of methane oxidation over hematite: A combined experimental and theoretical study. J. Am. Chem. Soc. 142 (40), 17119–17130. doi:10.1021/jacs.0c07179
Horn, R., and Schlögl, R. (2014). Methane activation by heterogeneous catalysis. Catal. Lett. 145 (1), 23–39. doi:10.1007/s10562-014-1417-z
Hu, L. H., Peng, Q., and Li, Y. D. (2008). Selective synthesis of Co3O4 nanocrystal with different shape and crystal plane effect on catalytic property for methane combustion. J. Am. Chem. Soc. 130 (48), 16136–16137. doi:10.1021/ja806400e
Hu, W. D., Lan, J. G., Guo, Y., Cao, X. M., and Hu, P. (2016). Origin of efficient catalytic combustion of methane over Co3O4(110): Active low-coordination lattice oxygen and cooperation of multiple active sites. ACS Catal. 6 (8), 5508–5519. doi:10.1021/acscatal.6b01080
Huang, E., Orozco, I., Ramírez, P. J., Liu, Z. Y., Zhang, F., Mahapatra, M., et al. (2021). Selective methane oxidation to methanol on ZnO/Cu2O/Cu (111) catalysts: Multiple site-dependent behaviors. J. Am. Chem. Soc. 143, 19018–19032. doi:10.1021/jacs.1c08063
Huang, L., Zhang, X., Lin, C. H. E. N., and Lecheng, L. (2012). Promotional effect of CeO2 and Y2O3 on CuO/ZrO2 catalysts for methane combustion. J. Rare Earths 30 (2), 123–127. doi:10.1016/S1002-0721(12)60007-6
Huang, W., Zha, W. W., Zhao, D. L., and Feng, S. J. (2019). The effect of active oxygen species in nano-ZnCr2O4 spinel oxides for methane catalytic combustion. Solid State Sci. 87, 49–52. doi:10.1016/j.solidstatesciences.2018.11.006
Huang, X., Li, J., Wang, J., Li, Z., and Xu, J. (2020). Catalytic combustion of methane over a highly active and stable NiO/CeO2 catalyst. Front. Chem. Sci. Eng. 14 (4), 534–545. doi:10.1007/s11705-019-1821-4
Jia, J. B., Ran, R., Guo, R. Q., Wu, X. D., and Weng, D. (2018). ZrO2-supported α-MnO2: Improving low-temperature activity and stability for catalytic oxidation of methane. Prog. Nat. Sci. Mater. Int. 28, 296–300. doi:10.1016/j.pnsc.2018.04.005
Jia, J. B., Ran, R., Wu, X. D., Chen, W., Si, Z. C., Weng, D., et al. (2019). Tuning nonstoichiometric defects in single-phase MnOx for methane complete oxidation. Mol. Catal. 467, 120–127. doi:10.1016/j.mcat.2019.01.032
Jia, J. B., Zhang, P. Y., and Chen, L. (2016). Catalytic decomposition of gaseous ozone over manganese dioxides with different crystal structures. Appl. Catal. B Environ. 189, 210–218. doi:10.1016/j.apcatb.2016.02.055
Jia, Y. C., Wang, S. Y., Lu, J. Q., and Luo, M. F. (2016). Effect of structural properties of mesoporous Co3O4 catalysts on methane combustion. Chem. Res. Chin. Univ. 32 (5), 808–811. doi:10.1007/s40242-016-6141-3
Jodłowski, P. J., Chlebda, D., Piwowarczyk, E., Chrzan, M., Jędrzejczyk, R. J., Sitarz, M., et al. (2016). In situ and operando spectroscopic studies of sonically aided catalysts for biogas exhaust abatement. J. Mol. Struct. 1126, 132–140. doi:10.1016/j.molstruc.2016.02.039
Kašpar, J., Fornasiero, P., and Graziani, M. (1999). Use of CeO2-based oxides in the three-way catalysis. Catal. Today 50 (2), 285–298. doi:10.1016/S0920-5861(98)00510-0
Knapp, D., and Ziegler, T. (2008). Methane dissociation on the ceria (111) surface. J. Phys. Chem. C 112 (44), 17311–17318. doi:10.1021/jp8039862
Kong, Q., Yin, Y., Xue, B., Jin, Y., Feng, W., Chen, Z. G., et al. (2018). Improved catalytic combustion of methane using CuO nanobelts with predominantly (001) surfaces. Beilstein J. Nanotechnol. 9 (1), 2526–2532. doi:10.3762/bjnano.9.235
Kuo, C. H., Li, W. K., Song, W. Q., Luo, Z., Poyraz, A. S., Guo, Y., et al. (2014). Facile synthesis of Co3O4@CNT with high catalytic activity for CO oxidation under moisture-rich conditions. ACS Appl. Mat. Interfaces 6, 11311–11317. doi:10.1021/am501815d
Larrondo, S., Vidal, M. A., Irigoyen, B., Craievich, A. F., Lamas, D. G., and Fábregas, I. O. (2005). Preparation and characterization of Ce/Zr mixed oxides and their use as catalysts for the direct oxidation of dry CH4. Catal. Today 53–59. doi:10.1016/j.cattod.2005.07.110
Li, J. A., Li, M. M., Gui, P., Zheng, L. N., Liang, J. S., Xue, G., et al. (2019). Hydrothermal synthesis of sandwich interspersed LaCO3OH/Co3O4/graphene oxide composite and the enhanced catalytic performance for methane combustion. Catal. Today 327, 134–142. doi:10.1016/j.cattod.2018.05.027
Li, J. H., Liang, X., Xu, S. C., and Hao, J. M. (2009). Catalytic performance of manganese cobalt oxides on methane combustion at low temperature. Appl. Catal. B Environ. 90 (1-2), 307–312. doi:10.1016/j.apcatb.2009.03.027
Li, J. L., Zhou, S. D., Zhang, J., Schlangen, M., Usharani, D., Shaik, S., et al. (2016). Mechanistic variants in gas-phase metal-oxide mediated activation of methane at ambient conditions. J. Am. Chem. Soc. 138 (35), 11368–11377. doi:10.1021/jacs.6b07246
Li, K., Liu, K., Xu, D. J., Ni, H., Shen, F. X., Chen, T., et al. (2019). Lean methane oxidation over Co3O4/Ce0.75Zr0.25 catalysts at low-temperature: Synergetic effect of catalysis and electric field. Chem. Eng. J. 369, 660–671. doi:10.1016/j.cej.2019.03.059
Li, L., Chen, H. W., Zhang, C. Y., and Fei, Z. Y. (2019). Ultrafine cobalt oxide nanoparticles embedded in porous SiO2 matrix as efficient and stable catalysts for methane combustion. Mol. Catal. 469, 155–160. doi:10.1016/j.mcat.2018.12.028
Li, M. M., Gui, P., Zheng, L. N., Li, J. A., Xue, G., Liang, J. S., et al. (2018). Active component migration and catalytic properties of nitrogen modified composite catalytic materials. Catalysts 8 (4), 125. doi:10.3390/catal8040125
Li, Y. X., Guo, Y. H., and Xue, B. (2009). Catalytic combustion of methane over M (Ni, Co, Cu) supported on ceria-magnesia. Fuel Process. Technol. 90 (5), 652–656. doi:10.1016/j.fuproc.2008.12.002
Liang, Z., Li, T., Kim, M., Asthagiri, A., and Weaver, J. F. (2017). Low-temperature activation of methane on the IrO2(110) surface. Science 356, 299–303. doi:10.1126/science.aam9147
Lin, F. W., Wang, Z. H., Shao, J. M., Yuan, D. K., He, Y., Zhu, Y. Q., et al. (2017). Catalyst tolerance to SO2 and water vapor of Mn based bimetallic oxides for NO deep oxidation by ozone. RSC Adv. 7 (40), 25132–25143. doi:10.1039/c7ra04010e
Liu, C., Xian, H., Jiang, Z., Wang, L. H., Zhang, J., Zheng, L. R., et al. (2015). Insight into the improvement effect of the Ce doping into the SnO2 catalyst for the catalytic combustion of methane. Appl. Catal. B Environ. 176-177, 542–552. doi:10.1016/j.apcatb.2015.04.042
Liu, F. X., Sang, Y. Y., Ma, H. W., Li, Z. P., and Gao, Z. M. (2017). Nickel oxide as an effective catalyst for catalytic combustion of methane. J. Nat. Gas. Sci. Eng. 41, 1–6. doi:10.1016/j.jngse.2017.02.025
Liu, L., Yao, Z., Liu, B., and Dong, L. (2010). Correlation of structural characteristics with catalytic performance of CuO/CexZr1-xO2 catalysts for NO reduction by CO. J. Catal. 275 (1), 45–60. doi:10.1016/j.jcat.2010.07.024
Liu, Z., Huang, E., Orozco, I., Liao, W. J., Palomino, R. M., Rui, N., et al. (2020). Water-promoted interfacial pathways in methane oxidation to methanol on a CeO2-Cu2O catalyst. Science 368, 513–517. doi:10.1126/science.aba5005
Lu, Y. F., Chou, F. C., Lee, F. C., Lin, C. Y., and Tsai, D. H. (2016). Synergistic catalysis of methane combustion using Cu-Ce-O hybrid nanoparticles with high activity and operation stability. J. Phys. Chem. C 120 (48), 27389–27398. doi:10.1021/acs.jpcc.6b09441
Lyu, Y. M., Jocz, J. N., Xu, R., Williams, O. C., and Sievers, C. (2021). Selective oxidation of methane to methanol over ceria-zirconia supported mono and bimetallic transition metal oxide catalysts. ChemCatChem 13 (12), 2832–2842. doi:10.1002/cctc.202100268
Mukherjee, D., Rao, B. G., and Reddy, B. M. (2016). CO and soot oxidation activity of doped ceria: Influence of dopants. Appl. Catal. B Environ. 197, 105–115. doi:10.1016/j.apcatb.2016.03.042
Neatu, S., Trandafir, M. M., Stănoiu, A., Florea, O. G., Simion, C. E., Leonat, L. N., et al. (2019). Bulk versus surface modification of alumina with Mn and Ce based oxides for CH4 catalytic combustion. Materials 12 (11), 1771. doi:10.3390/ma12111771
Ordóñez, S., Paredes, J. R., and Díez, F. V. (2008). Sulphur poisoning of transition metal oxides used as catalysts for methane combustion. Appl. Catal. A General 341 (1-2), 174–180. doi:10.1016/j.apcata.2008.02.042
Palella, A., Spadaro, L., Di Chio, R., and Arena, F. (2021). Effective low-temperature catalytic methane oxidation over MnCeOx catalytic compositions. Catal. Today 379, 240–249. doi:10.1016/j.cattod.2020.11.010
Palmqvist, A. E. C., Johansson, E. M., Järås, S. G., and Muhammed, M. (1998). Total oxidation of methane over doped nanophase cerium oxides. Catal. Lett. 56 (1), 69–75. doi:10.1023/A:1019032306894
Paredes, J. R., Dı´az, E., Dı´ez, F. V., and Ordo´n˜ez, S. (2009). Combustion of methane in lean mixtures over bulk transition-metal oxides: Evaluation of the activity and self-deactivation. Energy fuels. 23 (1), 86–93. doi:10.1021/ef800704e
Paredes, J. R., Ordóñez, S., Vega, A., and Dıez, F. V. (2004). Catalytic combustion of methane over red mud-based catalysts. Appl. Catal. B Environ. 47 (1), 37–45. doi:10.1016/S0926-3373(03)00325-4
Park, P. W., and Ledford, J. S. (1998). The influence of surface structure on the catalytic activity of alumina supported copper oxide catalysts. Oxidation of carbon monoxide and methane. Appl. Catal. B Environ. 15 (3-4), 221–231. doi:10.1016/S0926-3373(98)80008-8
Pecchi, G., Jiliberto, M. G., Buljan, A., and Delgado, E. J. (2011). Relation between defects and catalytic activity of calcium doped LaFeO3 perovskite. Solid State Ionics 187 (1), 27–32. doi:10.1016/j.ssi.2011.02.014
Pecchi, G., Reyes, P., Zamora, R., López, T., and Gómez, R. (2005). Effect of the promoter and support on the catalytic activity of Pd-CeO2-supported catalysts for CH4 combustion. J. Chem. Technol. Biotechnol. 80 (3), 268–272. doi:10.1002/jctb.1120
Pfefferle, L. D., and Pfefferle, W. C. (1987). Catalysis in combustion. Catal. Rev. 29 (2-3), 219–267. doi:10.1080/01614948708078071
Popescu, I., Tanchoux, N., Tichit, D., and Marcu, I. C. (2017). Total oxidation of methane over supported CuO: Influence of the MgxAlyO support. Appl. Catal. A General 538, 81–90. doi:10.1016/j.apcata.2017.03.012
Pratt, C., and Tate, K. (2018). Mitigating methane: Emerging technologies to combat climate change’s second leading contributor. Environ. Sci. Technol. 52, 6084–6097. doi:10.1021/acs.est.7b04711
Pu, Z. Y., Liu, Y., Zhou, H., Huang, W. Z., Zheng, Y. F., Li, X. N., et al. (2017). Catalytic combustion of lean methane at low temperature over ZrO2-modified Co3O4 catalysts. Appl. Surf. Sci. 422, 85–93. doi:10.1016/j.apsusc.2017.05.231
Raj, B. A. (2016). Methane emission control. Johns. Matthey Technol. Rev. 60 (4), 228–235. doi:10.1595/205651316X692554
Rodríguez-Fernández, J., Sun, Z. Z., Zhang, L., Tan, T., Curto, A., Fester, J., et al. (2019). Structural and electronic properties of Fe dopants in cobalt oxide nanoislands on Au(111). J. Chem. Phys. 150, 041731. doi:10.1063/1.5052336
Ross, M. O., and Rosenzweig, A. C. (2017). A tale of two methane monooxygenases. J. Biol. Inorg. Chem. 22, 307–319. doi:10.1007/s00775-016-1419-y
Sanchis, R., García, A., Ivars-Barceló, F., Taylor, S. H., García, T., Dejoz, A., et al. (2021). Highly active Co3O4-based catalysts for total oxidation of light C1-C3 alkanes prepared by a simple soft chemistry method: Effect of the heat-treatment temperature and mixture of alkanes. Materials 14, 7120. doi:10.3390/ma14237120
Seeburg, D., Liu, D. J., Radnik, J., Atia, H., Pohl, M. M., Schneider, M., et al. (2018). Structural changes of highly active Pd/MeOx (Me = Fe, Co, Ni) during catalytic methane combustion. Catalysts 8 (2), 42. doi:10.3390/catal8020042
Setiawan, A., Kennedy, E. M., Dlugogorski, B. Z., Adesina, A. A., and Stockenhuber, M. (2015). The stability of Co3O4, Fe2O3, Au/Co3O4 and Au/Fe2O3 catalysts in the catalytic combustion of lean methane mixtures in the presence of water. Catal. Today 258, 276–283. doi:10.1016/j.cattod.2014.11.031
Shu, Y., Wang, M. Y., Duan, X. L., Liu, D. D., Yang, S. Z., Zhang, P. F., et al. (2022). Low-temperature total oxidation of methane by pore- and vacancy-engineered NiO catalysts. AIChE J. 68 (6), e17664. doi:10.1002/aic.17664
Siakavelas, G. I., Charisiou, N. D., AlKhoori, A., Gaber, S., Sebastian, V., and Hinder, S. J. (2022b). Oxidative coupling of methane on Li/CeO2 based catalysts: Investigation of the effect of Mg- and La-doping of the CeO2 support. Mol. Catal. 520, 112157. doi:10.1016/j.mcat.2022.112157
Siakavelas, G. I., Charisiou, N. D., AlKhoori, A., Sebastian, V., Hinder, S. J., and Baker, M. (2022a). Cerium oxide catalysts for oxidative coupling of methane reaction: Effect of lithium, samarium and lanthanum dopants. J. Environ. Chem. Eng. 10 (2), 107259. doi:10.1016/j.jece.2022.107259
Siakavelas, G. I., Charisiou, N. D., AlKhoori, S., AlKhoori, A. A., Sebastian, V., and Hinder, S. J. (2021). Highly selective and stable nickel catalysts supported on ceria promoted with Sm2O3, Pr2O3 and MgO for the CO2 methanation reaction. Appl. Catal. B Environ. 282, 119562. doi:10.1016/j.apcatb.2020.119562
Stoian, M., Rogé, V., Lazar, L., Maurer, T., Védrine, J. C., Marcu, I. C., et al. (2021). Total oxidation of methane on oxide and mixed oxide ceria-containing catalysts. Catalysts 11 (4), 427. doi:10.3390/catal11040427
Sun, L. L., Wang, Y., Wang, C. M., Xie, Z. K., Guan, N. J., Li, L. D., et al. (2021). Water-involved methane-selective catalytic oxidation by dioxygen over copper zeolites. Chem 7 (6), 1557–1568. doi:10.1016/j.chempr.2021.02.026
Sun, Y. N., Liu, J. W., Song, J. J., Huang, S. S., Yang, N. T., Zhang, J., et al. (2016). Exploring the effect of Co3O4 nanocatalysts with different dimensional architectures on methane combustion. ChemCatChem 8 (3), 540–545. doi:10.1002/cctc.201501056
Sushkevich, V. L., Palagin, D., Ranocchiari, M., and van Bokhoven, J. A. (2017). Selective anaerobic oxidation of methane enables direct synthesis of methanol. Science 356, 523–527. doi:10.1126/science.aam9035
Tang, J. J., and Liu, B. (2016). Reactivity of the Fe2O3 (0001) surface for methane oxidation: A GGA+ U study. J. Phys. Chem. C 120 (12), 6642–6650. doi:10.1021/acs.jpcc.6b00374
Tang, W., Hu, Z., Wang, M., Stucky, G. D., Metiu, H., McFarland, E. W., et al. (2010). Methane complete and partial oxidation catalyzed by Pt-doped CeO2. J. Catal. 273 (2), 125–137. doi:10.1016/j.jcat.2010.05.005
Tang, X. F., Li, J. H., and Hao, J. M. (2008). Synthesis and characterization of spinel Co3O4 octahedra enclosed by the {111} facets. Mat. Res. Bull. 43 (11), 2912–2918. doi:10.1016/j.materresbull.2007.12.009
Tao, F. F., Shan, J. J., Nguyen, L., Wang, Z. Y., Zhang, S. R., Zhang, L., et al. (2015). Understanding complete oxidation of methane on spinel oxides at a molecular level. Nat. Commun. 6, 7798. doi:10.1038/ncomms8798
Teng, F., Su, X., and Wang, X. (2019). Can China peak its non-CO2 GHG emissions before 2030 by implementing its nationally determined contribution? Environ. Sci. Technol. 53, 12168–12176. doi:10.1021/acs.est.9b04162
Tepamatr, P., Laosiripojana, N., and Charojrochkul, S. (2016). Water gas shift reaction over monometallic and bimetallic catalysts supported by mixed oxide materials. Appl. Catal. A General 523, 255–262. doi:10.1016/j.apcata.2016.06.023
Toscani, L. M., Curyk, P. A., Zimicz, M. G., Halac, E. B., Saleta, M. E., Lamas, D. G., et al. (2019). Methane catalytic combustion over CeO2-ZrO2-Sc2O3 mixed oxides. Appl. Catal. A General 587, 117235. doi:10.1016/j.apcata.2019.117235
Trimm, D. L., and Lam, C. W. (1980). The combustion of methane on platinum-alumina fibre catalysts-I: Kinetics and mechanism. Chem. Eng. Sci. 35 (6), 1405–1413. doi:10.1016/0009-2509(80)85134-7
Vedrine, J. C., Millet, J. M. M., and Volta, J. C. (1996). Molecular description of active sites in oxidation reactions: Acid-base and redox properties, and role of water. Catal. Today 32 (1-4), 115–123. doi:10.1016/S0920-5861(96)00185-X
Veldsink, J. W., Versteeg, G. F., and van Swaaij, W. P. M. (1995). Intrinsic kinetics of the oxidation of methane over an industrial copper(II) oxide catalyst on a γ-alumina support. Chem. Eng. J. Biochem. Eng. J. 57, 273–283. doi:10.1016/0923-0467(94)02872-8
Vickers, S. M., Gholami, R., Smith, K. J., and MacLachlan, M. J. (2015). Mesoporous Mn- and La-doped cerium oxide/cobalt oxide mixed metal catalysts for methane oxidation. ACS Appl. Mat. Interfaces 7 (21), 11460–11466. doi:10.1021/acsami.5b02367
Wang, C. H., and Lin, S. S. (2004). Study on catalytic incineration of methane using Cr2O3/γ-Al2O3 as the catalyst. J. Environ. Sci. Health Part A 39 (6), 1631–1641. doi:10.1081/ese-120037859
Wang, F. G., Zhang, L. J., Xu, L. L., Deng, Z. Y., and Shi, W. D. (2017). Low temperature CO oxidation and CH4 combustion over Co3O4 nanosheets. Fuel 203, 419–429. doi:10.1016/j.fuel.2017.04.140
Wang, H. W., Li, J. L., Liu, W. G., Xu, X., Wei, X. F., Chao, L., et al. (2019). Enhancing catalytic CH4 oxidation over Co3O4/SiO2 core-shell catalyst by substituting Co2+ with Mn2+. J. Dispers. Sci. Technol. 42 (1), 82–92. doi:10.1080/01932691.2019.1661257
Wang, Q., Peng, Y., Fu, J., Kyzas, G. Z., Billah, S. M. R., An, S. Q., et al. (2015). Synthesis, characterization, and catalytic evaluation of Co3O4/γ-Al2O3 as methane combustion catalysts: Significance of Co species and the redox cycle. Appl. Catal. B Environ. 168-169, 42–50. doi:10.1016/j.apcatb.2014.12.016
Wang, T., Wang, J. Y., Sun, Y. M., Duan, Y., Sun, S. N., Hu, X., et al. (2019). Origin of electronic structure dependent activity of spinel ZnNixCo2-xO4 oxides for complete methane oxidation. Appl. Catal. B Environ. 256, 117844. doi:10.1016/j.apcatb.2019.117844
Wang, X. Y., Liu, Y., Zhang, Y. Y., Zhang, T. H., Chang, H. Z., Zhang, Y. F., et al. (2018). Structural requirements of manganese oxides for methane oxidation: XAS spectroscopy and transition-state studies. Appl. Catal. B Environ. 229, 52–62. doi:10.1016/j.apcatb.2018.02.007
Wang, Y. N., Zhang, R. J., and Yan, B. H. (2022). Ni/Ce0.9Eu0.1O1.95 with enhanced coke resistance for dry reforming of methane. J. Catal. 407, 77–89. doi:10.1016/j.jcat.2022.01.020
Wang, Y. Q., Rosowski, F., Schlögl, R., and Trunschke, A. (2022). Oxygen exchange on vanadium pentoxide. J. Phys. Chem. C 126, 3443–3456. doi:10.1021/acs.jpcc.2c00174
Wang, Z. X., Lin, J., Xu, H., Zheng, Y., Xiao, Y. H., Zheng, Y., et al. (2021). Zr-doped NiO nanoparticles for low-temperature methane combustion. ACS Appl. Nano Mat. 4, 11920–11930. doi:10.1021/acsanm.1c02487
Wasalathanthri, N. D., Poyraz, A. S., Biswas, S., Meng, Y., Kuo, C.-H., Kriz, D. A., et al. (2015). High-performance catalytic CH4 oxidation at low temperatures: Inverse micelle synthesis of amorphous mesoporous manganese oxides and mild transformation to K2-xMn8O16 and ϵ-MnO2. J. Phys. Chem. C 119 (3), 1473–1482. doi:10.1021/jp5108558
Wu, H. C., and Ku, Y. (2018). Enhanced performance of chemical looping combustion of methane with Fe2O3/Al2O3/TiO2 oxygen carrier. RSC Adv. 8, 39902–39912. doi:10.1039/c8ra07863g
Wu, H., Pantaleo, G., Di Carlo, G., Guo, S., Marcì, G., Concepción, P., et al. (2015). Co3O4 particles grown over nanocrystalline CeO2: Influence of precipitation agents and calcination temperature on the catalytic activity for methane oxidation. Catal. Sci. Technol. 5 (3), 1888–1901. doi:10.1039/c4cy01158a
Xu, J., Zhang, Y., Liu, Y., Fang, X., Xu, X., and Liu, W. (2019). Optimizing the reaction performance of La2Ce2O7-based catalysts for oxidative coupling of methane (OCM) at lower temperature by lattice doping with Ca cations. Eur. J. Inorg. Chem. 2, 183–194. doi:10.1002/ejic.201801250
Xu, X. L., Li, L., Yu, F., Peng, H. G., Fang, X. Z., Wang, X., et al. (2017). Mesoporous high surface area NiO synthesized with soft templates: Remarkable for catalytic CH4 deep oxidation. Mol. Catal. 441, 81–91. doi:10.1016/j.mcat.2017.08.005
Yang, L., Huang, J. X., Ma, R., You, R., Zeng, H., Rui, Z. B., et al. (2019). Metal-organic framework-derived IrO2/CuO catalyst for selective oxidation of methane to methanol. ACS Energy Lett. 4 (12), 2945–2951. doi:10.1021/acsenergylett.9b01992
Yang, L. Q. Q., Heinlein, J., Hua, C., Gao, R. X., Hu, S., Pfefferle, L. D., et al. (2022). Emerging dual-functional 2D transition metal oxides for carbon capture and utilization: A review. Fuel 324, 124706. doi:10.1016/j.fuel.2022.124706
Yang, R. J., Guo, Z. J., Cai, L. X., Zhu, R. S., Fan, Y. Y., Zhang, Y. F., et al. (2021). Investigation into the phase-activity relationship of MnO2 nanomaterials toward ozone-assisted catalytic oxidation of toluene. Small 17 (50), 2103052. doi:10.1002/smll.202103052
Ye, Y. C., Zhao, Y. T., Ni, L. L., Jiang, K. D., Tong, G. X., Zhao, Y. L., et al. (2016). Facile synthesis of unique NiO nanostructures for efficiently catalytic conversion of CH4 at low temperature. Appl. Surf. Sci. 362, 20–27. doi:10.1016/j.apsusc.2015.11.213
Yu, Q., Liu, C. X., Li, X. Y., Wang, C., Wang, X. X., Cao, H. J., et al. (2020). N-doping activated defective Co3O4 as an efficient catalyst for low-temperature methane oxidation. Appl. Catal. B Environ. 269, 118757. doi:10.1016/j.apcatb.2020.118757
Yu, Q., Wang, C., Li, X. Y., Li, Z., Wang, L., Zhang, Q., et al. (2019). Engineering an effective MnO2 catalyst from LaMnO3 for catalytic methane combustion. Fuel 239, 1240–1245. doi:10.1016/j.fuel.2018.11.094
Yuan, X. Z., Chen, S. Y., Chen, H., and Zhang, Y. C. (2013). Effect of Ce addition on Cr/γ-Al2O3 catalysts for methane catalytic combustion. Catal. Commun. 35, 36–39. doi:10.1016/j.catcom.2013.02.005
Zasada, F., Janas, J., Piskorz, W., Gorczyńska, M., and Sojka, Z. (2017). Total oxidation of lean methane over cobalt spinel nanocubes controlled by the self-adjusted redox state of the catalyst: Experimental and theoretical account for interplay between the Langmuir-hinshelwood and mars-van krevelen mechanisms. ACS Catal. 7 (4), 2853–2867. doi:10.1021/acscatal.6b03139
Zedan, A. F., and AlJaber, A. S. (2019). Combustion synthesis of non-precious CuO-CeO2 nanocrystalline catalysts with enhanced catalytic activity for methane oxidation. Materials 12 (6), 878. doi:10.3390/ma12060878
Zhang, K., Peng, X. B., Cao, Y. N., Yang, H. G., Wang, X. Y., Zhang, Y. Y., et al. (2019). Effect of MnO2 morphology on its catalytic performance in lean methane combustion. Mat. Res. Bull. 111, 338–341. doi:10.1016/j.materresbull.2018.11.023
Zhang, X., Jin, X., Bao, L. R., Zhang, M. C., Song, R. M., Yu, W., et al. (2021). Construction of defective cobalt oxide for methane combustion by oxygen vacancy engineering. New J. Chem. 45 (28), 12655–12660. doi:10.1039/d1nj01296g
Zhang, X. Y., House, S. D., Tang, Y., Nguyen, L., Li, Y. T., Opalade, A. A., et al. (2018). Complete oxidation of methane on NiO nanoclusters supported on CeO2 nanorods through synergistic effect. ACS Sustain. Chem. Eng. 6, 6467–6477. doi:10.1021/acssuschemeng.8b00234
Zhang, Y. G., Qin, Z. F., Wang, G. F., Zhu, H. Q., Dong, M., Li, S. N., et al. (2013). Catalytic performance of MnOx-NiO composite oxide in lean methane combustion at low temperature. Appl. Catal. B Environ. 129, 172–181. doi:10.1016/j.apcatb.2012.09.021
Zhang, Y., Xu, J., Xu, X., Xi, R., Liu, Y., and Fang, X. (2020). Tailoring La2Ce2O7 catalysts for low temperature oxidative coupling of methane by optimizing the preparation methods. Catal. Today 355, 518–528. doi:10.1016/j.cattod.2019.06.060
Zheng, Y. F., Yu, Y. Q., Zhou, H., Huang, W. Z., and Pu, Z. Y. (2020). Combustion of lean methane over Co3O4 catalysts prepared with different cobalt precursors. RSC Adv. 10 (8), 4490–4498. doi:10.1039/c9ra09544f
Zheng, Y., Wang, C., Li, J. J., Zhong, F. L., Xiao, Y. H., Jiang, L. L., et al. (2020). Enhanced methane oxidation over Co3O4-In2O3-x composite oxide nanoparticles via controllable substitution of Co3+/Co2+ by In3+ Ions. ACS Appl. Nano Mat. 3, 9470–9479. doi:10.1021/acsanm.0c02075
Zhong, L., Fang, Q. Y., Li, X., Li, Q., Zhang, C., Chen, G., et al. (2019). SO2 resistance of Mn-Ce catalysts for lean methane combustion: Effect of the preparation method. Catal. Lett. 149 (12), 3268–3278. doi:10.1007/s10562-019-02896-3
Zhu, Y. X., Murwani, I. K., Zhou, C. J., Kemnitz, E., and Xie, Y. C. (2003). Isotopic studies of Sn-Cr binary oxide catalysts for methane total oxidation. Catal. Lett. 85 (3-4), 205–211. doi:10.1023/A:1022197830984
Keywords: methane, catalytic combustion, heterogeneous catalysis, transition metal oxide, reaction mechanism
Citation: Gao Y, Jiang M, Yang L, Li Z, Tian F-X and He Y (2022) Recent progress of catalytic methane combustion over transition metal oxide catalysts. Front. Chem. 10:959422. doi: 10.3389/fchem.2022.959422
Received: 01 June 2022; Accepted: 28 June 2022;
Published: 08 August 2022.
Edited by:
Shanhui Zhu, Institute of Coal Chemistry (CAS), ChinaReviewed by:
Maria A. Goula, University of Western Macedonia, GreeceMiriam Navlani-García, University of Alicante, Spain
Copyright © 2022 Gao, Jiang, Yang, Li, Tian and He. This is an open-access article distributed under the terms of the Creative Commons Attribution License (CC BY). The use, distribution or reproduction in other forums is permitted, provided the original author(s) and the copyright owner(s) are credited and that the original publication in this journal is cited, in accordance with accepted academic practice. No use, distribution or reproduction is permitted which does not comply with these terms.
*Correspondence: Yulian He, eXVsaWFuLmhlQHNqdHUuZWR1LmNu
†These authors share first authorship