Radioiodine sorbent selection criteria
- 1Pacific Northwest National Laboratory, Richland, WA, United States
- 2University of Nevada Reno, Reno, NV, United States
Methods for preventing radioiodine from entering the environment are needed in processes related to nuclear energy and medical isotope production. The development and performance of many different types of sorbents to capture iodine have been reported on for decades; however, there is yet to be a concise overview on the important parameters that should be considered when selecting a material for chemically capturing radioiodine. This paper summarizes several criteria that should be considered when selecting candidate sorbents for implementation into real-world systems. The list of selection criteria discussed are 1) optimal capture performance, 2) kinetics of adsorption, 3) performance under relevant process conditions, 4) properties of the substrate that supports the getter, and 5) environmental stability and disposition pathways for iodine-loaded materials.
Introduction
Several key parameters need to be considered when selecting a sorbent for capturing radioiodine, which can be relevant for a variety of situations including (a) preventing release of gaseous or ionic iodine species after a nuclear accident into the surrounding environment, (b) capture from the off-gas stream in a molten salt reactor (MSR), (c) capture of gaseous species during reprocessing of used nuclear fuel, and (d) release during medical isotope production. The primary list of sorbent selection criteria includes 1) optimal iodine capture performance of the getter (i.e., loading capacity of chemisorbed iodine under saturated conditions), 2) kinetics of iodine adsorption (i.e., rate of iodine capture), 3) iodine capture performance under relevant process conditions (e.g., pH and temperature of stream, redox sensitivity in oxidizing/reducing environments, iodine selection preference over other components present in the system), 4) properties of the sorbent substrate that supports the getter (e.g., silica aerogel, carbon foam), 5) environmental stability and disposition pathways for iodine-loaded materials. These criteria, graphically shown in Figure 1, are not in any sort of priority order as needs drastically vary with the application. In this paper, these individual parameters will be discussed to provide a perspective on chemisorption-based sorption (compared to physisorption-based sorption) and the areas still in need of study (e.g., radiation stability, capture in a prototypical off-gas stream).
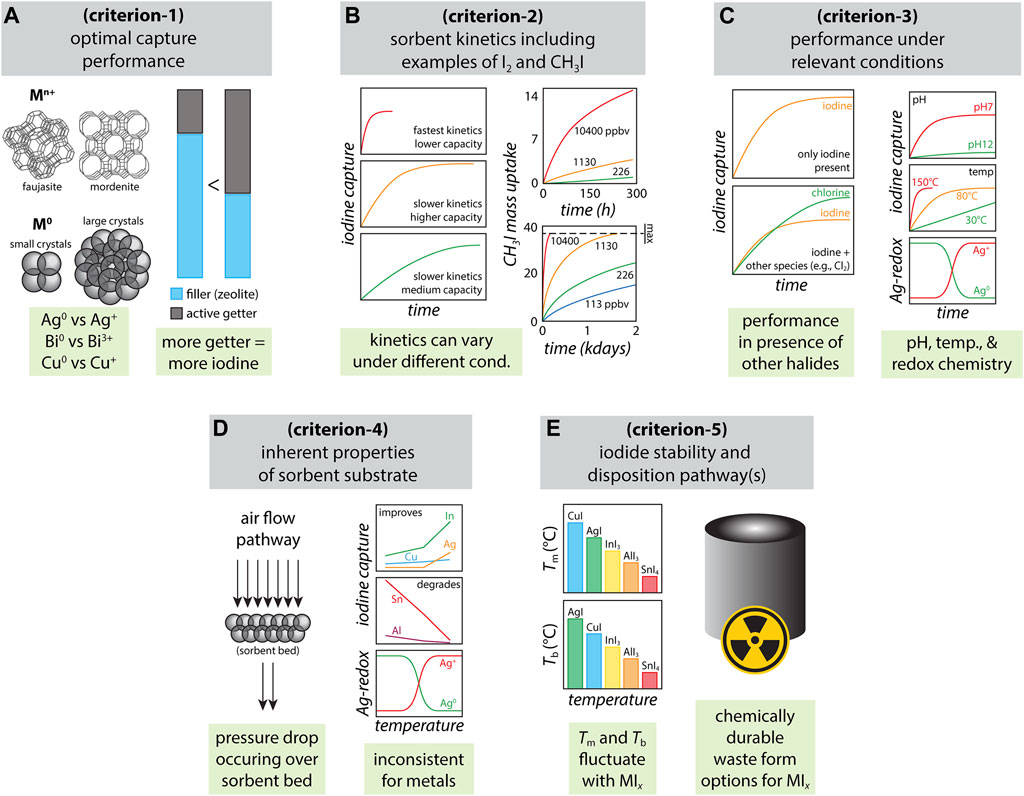
FIGURE 1. Summary of various parameters for consideration when selecting sorbents for radioiodine: (A) optimal capture performance including different types of Ag-zeolites that have different Ag loadings (Riley et al., 2022b) (criterion-1), (B) sorbent kinetics including examples based off actual data for I2 (Riley et al., 2014) for sulfide-based chalcogels and CH3I for Ag0-aerogels (Tang et al., 2021) [in parts per billion by volume (ppbv) CH3I concentration streams] (criterion-2), (C) performance under relevant conditions based off data for I/Cl coadsorption (Matyáš et al., 2021b) and other studies in this area (Riley et al., 2021; Riley et al., 2022a) (criterion-3), (D) inherent properties of sorbent substrate (Riley et al., 2021) (criterion-4), and (E) iodide stability and disposition pathways (Riley et al., 2021; Reiser et al., 2022) (criterion-5).
Optimal iodine capture performance
Stable materials with strong iodine chemisorption are needed for iodine capture to prevent the release of iodine with time or thermal decomposition during heating under prolonged sorbent bed operation as well as during the process to create a waste form (Figure 1A). Once candidate getters have been selected, iodine capture in a saturated environment is performed to understand the maximum loading capacity. Evaluating getter performance in an idealized environment (i.e., no competing or reacting species) is an initial critical assessment to understand the fundamental interactions with iodine before introducing complexity from the process stream.
Figure 2 shows some of the elements that have been explored in various forms for the capture of volatile iodine along with the expected MOyIx compounds based on expected metal oxidation states in the iodine-loaded complexes (Maeck et al., 1968; Maeck and Pence 1970; Pence et al., 1970; Pence et al., 1972; Matyáš et al., 2011; Riley et al., 2017; Riley et al., 2020; Matyáš et al., 2021a; Riley et al., 2021; Tesfay Reda et al., 2021). In practice, several of these base metals do not show iodine capture under saturated conditions so specialized conditions might be needed to realize their potential (Riley et al., 2021). Figure 2B provides an overview of the large range in maximum iodine loadings possible by simply selecting different getter metals.
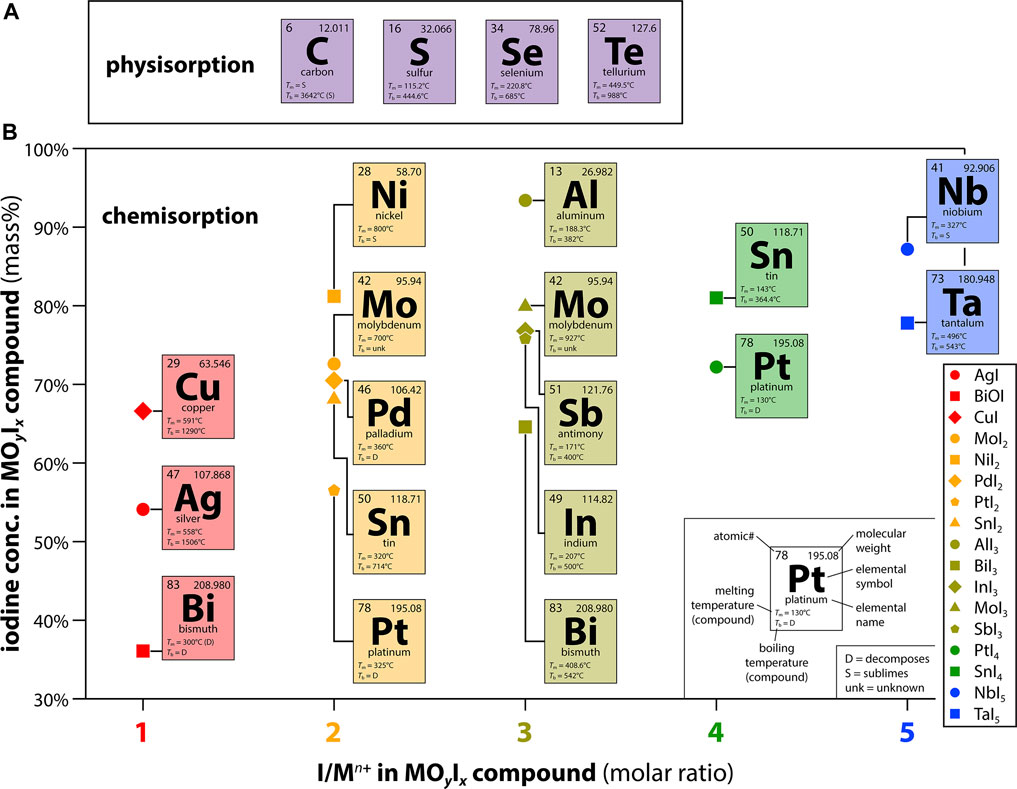
FIGURE 2. Summary of common species demonstrated in the literature for (A) physisorption-based or (B) chemisorption-based iodine capture processes. The data in (B) show the iodine concentration in the MOyIx compound as a function of the I/Mn+ ratio based on the metal oxidation state in the iodine-loaded complex.
Transition metals and post-transition metals have been extensively evaluated, most commonly in the form of metal-exchanged sorbents (Maeck et al., 1968; Maeck and Pence 1970; Pence et al., 1970; Pence et al., 1972; Riley et al., 2017; Riley et al., 2020) or metal-impregnated sorbents (Matyáš et al., 2011; Matyáš et al. 2018; Matyáš et al. 2021b). Although they are often less kinetically favorable than iodine interactions with metals, metal-oxide composites (e.g., single metal oxides, spinel oxides, metal hydroxides) (Muhire et al., 2022) are also of interest as most realistic off-gas environments are highly oxidizing (Jubin et al., 2018). The oxidation state of the getter (e.g., Ag0 vs. Ag+) plays a vital role in the sorption performance due to differences in reactivity with components in the process stream. Metals with the ability to form oxyhalides, especially those with structural analogues of minerals, are favorable as they tend to have prolonged capture performance and stability for long-term storage (Tesfay Reda et al., 2021).
In regard to metals, those where the formation of iodides are thermodynamically favored over oxides are preferred due to the complexity of the process stream, a factor discussed below (Strachan et al., 2011). One such metal is silver, which has been extensively studied for the chemisorption of iodine from gases and aqueous environments for many decades (Jubin 1979). Studies examining alternative metals have typically incorporated them into oxide-based [e.g., zeolites (Maeck and Pence 1970; Pence et al., 1972), silica (Matyáš et al., 2011; Riley et al., 2017; Riley et al., 2020)] or carbon-based (Muhire et al., 2022) substrates. As the choices of substrate and getter loading method can affect iodine capture, a recent study was conducted using pure metallic substrates to eliminate these artifacts (Riley et al., 2021). This study demonstrated that silver, and other pure metals such as Al0, Cu0, In0, and Sn0 can be used to capture large quantities of iodine in saturated conditions at various temperatures. A variety of iodine mass loadings result based on the oxidation state of the metal upon reaction with I2(g) to form the metal-iodide (MIx) complex, which for these metals is M1+ for Ag (AgI) and Cu (CuI), M3+ for Al (AlI3) and In (InI3), and M4+ for Sn (SnI4). The lower the molecular mass of the base metal and the higher the oxidation state of the metal in the MIx complex, the higher the overall iodine mass loading in the MIx complex (see Figure 2B for several examples).
Kinetics of iodine adsorption
Understanding the kinetics of iodine adsorption is essential because the sorbent is required to scavenge maximum amounts of available iodine as fast as possible before it can escape the vicinity of the sorption environment (Figure 1B). In off-gas streams generated during the reprocessing of used nuclear fuel or the processing of legacy waste, this environment would include a large volume of carrier gas with a very low concentration of iodine (Riley et al., 2016). It should also be noted here that the species of iodine can vary from application to application, and could include a mixture of a variety of different iodine species in the same stream [e.g., I2(g), ICl, CH3I] (Riley et al., 2016). Faster kinetics of adsorption means potentially less sorbent required to capture the same quantity of iodine (e.g., shorter bed depth) compared to sorbent with slower kinetics.
A comparison of the iodine sorption kinetics between silver and bismuth was conducted using nickel foam functionalized with either Ag0 (Ag-Ni) or Bi0 (Bi-Ni) (Tian et al., 2021). The study showed that Ag-Ni reached 90% of its saturation capacity in 30 min while 120 min was needed for the Bi-Ni. Although not specifically stated to contribute to the difference in sorption kinetics, significant differences were observed in the particle morphology after reacting with iodine. Iodine reactions with spherical silver particles led to the formation of larger AgI particles, while iodine interactions with rod-like bismuth particles led to the formation of needle-like structures. Changes in crystal structure and ability of iodine to penetrate through the oxide layer(s) present within the sorbents can also add to the time required for full reaction with the getter of choice.
Performance in relevant process conditions
The performance of the getter can change drastically depending on the environment in which it is used to capture radioiodine (Figure 1C). In the reprocessing of used nuclear fuel, extremes in pH are observed depending on the process, such as acidic pH conditions [e.g., HNO3(g), HF(g)] in off-gas streams or highly basic pH conditions in a caustic scrubber solution or a molten hydroxide scrubber (i.e., pH ≥ 12) (Riley et al., 2016). It is also an issue if the stability of the sorbent varies with temperature as some metals behave differently at low temperatures versus high temperatures (Riley et al., 2021). An additional point is that the performance of different getters can vary in terms of their abilities to scavenge low concentrations of the target species from a larger volume of competing species (e.g., Cl2) (Matyáš et al., 2021b). Finally, some of these environments can be highly oxidizing (e.g., NOx) which can cause unwanted reactions between free oxygen and the getter metals to oxidize active metal sites to metal oxides, inhibiting subsequent reactions with iodine (Backx et al., 1981; Campbell 1985; Bukhtiyarov et al., 1999; Matyáš et al., 2018).
Developing an understanding of iodine selection preference over other components present in the off-gas is critical as realistic conditions will contain competing components. The most obvious competitive species are other halogen-based compounds such as ICl and Cl2(g). The chemisorption of Ag-based species for Cl2(g) is not well studied but a recent study by Matyáš et al. (2021b) on the co-adsorption of Cl2(g) and I2(g) shows that the Ag0-functionalized silica aerogel can chemisorb both gases simultaneously and might have a preference of Cl2(g) > I2(g) based on this initial dataset. More work on these types of co-adsorption and competition experiments are needed for new sorbents so that the efficiency in real-world processing conditions can be predicted and evaluated accordingly.
Understanding sorbent performance in oxidizing environments is crucial as it will be the case in most of the known situations in which radioiodine capture is needed. An initial step in assessing oxidation potential is to conduct aging experiments, whereby candidate sorbents are subjected to relevant gas streams expected in the process stream for extended time periods and the sorbent performance is evaluated after these aging experiments. Aging experiments in 2% NO2 in dry air and 1% NO in N2 streams at 150 °C (for up to 6 months) were noted to drastically reduce the iodine capacity of reduced silver mordenite (Ag0Z) in a study by Wiechert et al. (2020), whereby Ag0 is oxidized to form molecular nitrates that are believed to be on the surfaces of the mordenite crystals. In their study, X-ray absorption spectroscopy was used to verify that a majority of the Ag present in the aged samples was in the oxidized (Ag+) state. The reactions shown in Eq. 1 (Backx et al., 1981; Campbell 1985; Bukhtiyarov et al., 1999), Eq. 2 (Bare et al., 1995), Eq. 3 (Outka et al., 1987; Zemlyanov et al., 1998), Eq. 4 (Carley et al., 1998), and Eq. 5 (Bao et al., 1999; Zemlyanov and Schlögl 2000) describe some of the mechanisms that are responsible for the effects observed in aged Ag0Z sorbents (note that “ads” refers to adsorbed species on the mordenite surfaces). Reactions between Ag0 and O2 or NOx species can result in oxidized silver species, such as Ag2O, in the mordenite. Also, Eq. 4 shows how residual Na in the Ag0Z can act as a catalyst for dissociative adsorption of NO to convert Ag0 to Ag2O. Eq. 5 shows how NO can convert Ag2O to AgNO3 and Ag0 so while the oxidation states are shifting around during aging, the types and distribution of oxidized species can also shift. Thus, the surface chemistry of Ag0-containing species under different environmental conditions is complex.
Properties of the sorbent substrate
The physical properties of the substrate supporting the getter play a large role in iodine capture, including specific surface area (SSA), pore structure, and mechanical stability (Figure 1D). From a facility operation standpoint, permeable substrates are important to prevent a significant pressure drop in the sorbent containment chamber. The typical solid sorbent approach for high-flow applications is a packed bed of granular, pelletized, or other forms (e.g., berl saddles) of a moderately high SSA sorbent functionalized with a modest amount of getter. High-SSA substrates with an open cell structure tend to be more permeable and allow carrier gases or solutions to easily flow through the sorbent. Sorbents with low permeability can cause tortuous pathways for gas flow to move through the column and can lead to attrition and particulation of the sorbent bed (generation of fines), reducing the effectiveness of the sorbent. Commonly reported sorbents include porous alumina impregnated with AgNO3 (e.g., AC6120 from Clariant), Ag-KTC/KTB (AgNO3-loaded amorphous silicic acid) (Cheng et al., 2015), zeolites like mordenite (i.e., IONEX Ag-900) and Ag-faujasite (i.e., IONEX Ag-400), Ag0-functionalized silica aerogels (Matyáš et al., 2018), and Ag0-functionalized xerogels (Chong et al., 2021), which all have SSA values of ∼50–250 m2 g−1. Higher SSA values also provide more sites for the getter to enable higher iodine loading. However, the higher the SSA value of the sorbent, the less mechanically stable they tend to be under prototypical conditions (Matyáš et al., 2018). Friable materials have a greater potential to release particulates containing radioiodine into downstream unit operations.
The chemical nature of the substrate can lead to direct reactions with iodine. In a recent report on a carbon foam, both physisorbed I2 and chemisorbed tetraiodoethene (C4I4) were reported after exposure to iodine (Baskaran et al., 2022). These reactions were diminished as the foam was functionalized with increasing amounts of bismuth as chemisorption between the bismuth and iodine dominated. This finding opens the door to tuning the ratio of physisorption to chemisorption for enhanced capture of iodine from dilute gas streams.
Another aspect to consider is when the substrate has another redox-sensitive element present within its matrix. An example of this is the Ag0-functionalized silica aerogel developed by Matyáš et al. (2011; 2018) where the sorbent contains thiol (–SH) groups to help tether the Ag0 crystals to the aerogel surface using (3-mercaptopropyl)trimethoxysilane. The addition of these thiol groups changes the Ag0/Ag+ redox chemistry and drastically reduces the effects of aging compared to Ag0Z sorbents that were treated under the same aging conditions. This is because the thiol groups (S[2-]) oxidize to sulfate (S[6+]O42-) during aging studies based on X-ray photoelectron spectroscopy data thereby reducing the impact on the Ag0 → Ag+ reactions (Matyáš et al., 2018).
Environmental stability and disposition pathways for iodine-loaded materials
The environmental stability of the metal iodide formed after capture considers the chemical surface reactivity and morphological changes that could occur during operation and upon long-term storage after capture (Figure 1E). Some examples of where this is important were documented in a recent paper by Riley et al. (2021) where some of the highest performing pure metals for I2(g) capture were indium (forming InI3) and tin (forming SnI4). While In and Sn metals have a tremendous affinity for I2(g) forming a triiodide and tetraiodide (i.e., 76.8 and 81.0 mass% iodine, shown in Figure 2B), respectively, neither MIx complex is environmentally stable. The SnI4 complex is not a very chemically durable species and InI3 is extremely hygroscopic leading to deliquescence in moisture-containing air (Riley et al., 2021). Oxyhalides, such as BiOI, are attractive from a stability standpoint; however, their formation can be complicated because they form as metastable BiI3 (that converts to BiOI) and reactions with Bi2O3 might not be thermodynamically favored. Therefore, iodine is initially release as BiOI forms, so extra Bi0 would need to be added to a waste form if this phase was formed to capture the evolving I2 during the phase transition—see Eq. 6. These are the types of parameters that need to be considered when designing next-generation sorbents for real-world applications.
The disposition pathway for the iodine-loaded material is very important as it has to meet repository requirements if disposal is the planned pathway. The disposition pathway of the iodine-loaded sorbent is dictated by stability of the iodine-containing compound during transformation of the sorbent into a waste form and the chemical durability of the waste form. Sorbent transformation into a waste form is performed to condense the material into a smaller volume and this prevents potentially friable material from being released into the environment. Volatilization of iodine during the processing to a waste form can be mitigated through low-temperature consolidation (Garino et al., 2011; Sava et al., 2012; Garino et al., 2016), spark plasma sintering (Le Gallet et al., 2010; Yao et al., 2014), or hot-isostatic pressing (Bruffey and Jubin 2015; Maddrell et al., 2015; Maddrell et al., 2019). Once synthesized, the resulting waste form undergoes a variety of testing protocols to determine its resistance to attack by atmospheric agents or aqueous solutions that simulate different potential repository conditions. These tests are often conducted at elevated temperatures and pressures to accelerate the leaching effects and predict material chemical durability over geological timescales.
Other considerations
In addition to the selection criteria provided above, other aspects exist that should be considered. One of the more important ones is the option of recycling the sorbent to enable reuse for future capture. For physisorption-based sorbents, this could involve iodine desorption through an elevated temperature process. For chemisorption-based sorbents, recycling would be a far more involved process whereby the iodine has to be chemically stripped from the getter by means of decomposition (e.g., through high-temperature treatment) or substitution reactions (e.g., through chemical means). For example, iodine from AgI can be driven into solutions using a sodium sulfide reaction to convert Ag in AgI to Ag2S (solid precipitate) whereby I is soluble as I− and Na+ remain in solution (Cao et al., 2017). It is possible that Ag2S could be recycled into future sorbents. Recycling of chemisorption-based sorbents would be very valuable from both economic and environmental perspectives because it would prevent expending limited precious metal resources.
The second aspect mentioned is the development and understanding of adsorption isotherm models. These types of models help researchers understand how and why particular species adsorb to substrates. There are six main types of experimentally observed adsorption isotherms, which can be used to describe both physisorption and chemisorption (Keller 2005). In realistic environments (i.e., mixed gases), no single isotherm can describe all of the adsorption phenomena in these systems. It is especially challenging for iodine as the concentration is extremely low compared to other species in representative off-gas streams. Regardless, adsorption isotherms can provide to guide the design and performance of future sorbents. Empirical models are needed at this time because the complexity of interactions between the adsorbing molecules and the sorbent hinder accurate predictions using theoretical models (e.g., statistical molecular methods).
Future perspectives
The majority of research on sorbents for iodine capture has looked at materials under idealistic and nonrepresentative conditions (Criterion 1 and Criterion 2). This fundamental base understanding of materials performance has provided us with information that was used to down select materials for investigation in environments with oxidants and/or competing species (Criterion 3). Studies on the role of the sorbent will are becoming more dominant as we learn that it can be used to use the properties to “protect” the getter from unwanted interactions or enhance capture performance through physisorption (Criterion 4). The final fate of the iodine-loaded material is of utmost importance, so the chemical durability and environmental stability need to be considered (Criterion 5). Studies that combine all the components of prototypical off-gas streams will be necessary for each application. A major aspect when evaluating stability of the sorbent and the final waste form is radiation stability, as both iodine-129 and iodine-131 decay to xenon. Many of the candidate sorbents in discussions today have not been evaluated under relevant radiological conditions to assess their stabilities within these environments. These unknowns provide a lot of exciting and important areas for future researchers. While this list does provide some of the more important criteria for consideration, it is by no means a comprehensive list and readers are referred to reviews on this topic for more comprehensive criteria and other aspects for sorbent evaluation.
Data availability statement
The original contributions in presented in this study are included in the article, further inquiries can be direct to the corresponding author.
Author contributions
BR and KC participated in the preparation of the original draft. Both authors listed have made a substantial, direct, and intellectual contribution to the work and approved it for publication.
Funding
This work was supported by the United States Department of Energy (DOE) Office of Nuclear Energy’s Nuclear Energy University Program (NEUP) under Contract DENE0008900. Pacific Northwest National Laboratory (PNNL) is operated by Battelle Memorial Institute for the DOE under contract DE-AC05-76RL01830.
Conflict of interest
The authors declare that the research was conducted in the absence of any commercial or financial relationships that could be construed as a potential conflict of interest.
Publisher’s note
All claims expressed in this article are solely those of the authors and do not necessarily represent those of their affiliated organizations, or those of the publisher, the editors and the reviewers. Any product that may be evaluated in this article, or claim that may be made by its manufacturer, is not guaranteed or endorsed by the publisher.
References
Backx, C., De Groot, C. P. M., and Biloen, P. (1981). Adsorption of oxygen on Ag(110) studied by high resolution ELS and TPD. Surf. Sci. 104 (1), 300–317. doi:10.1016/0039-6028(81)90137-0
Bao, X., Wild, U., Muhler, M., Pettinger, B., Schlögl, R., and Ertl, G. (1999). Coadsorption of nitric oxide and oxygen on the Ag(110) surface. Surf. Sci. 425 (2), 224–232. doi:10.1016/S0039-6028(99)00190-9
Bare, S. R., Griffiths, K., Lennard, W. N., and Tang, H. T. (1995). Generation of atomic oxygen on Ag(111) and Ag(110) using NO2: A TPD, LEED, HREELS, XPS and NRA study. Surf. Sci. 342 (1), 185–198. doi:10.1016/0039-6028(95)00670-2
Baskaran, K., Ali, M., Riley, B. J., Zharov, I., and Carlson, K. (2022). Evaluating the physisorption and chemisorption of iodine on bismuth-functionalized carbon foams. ACS Mat. Lett.
Bruffey, S. H., and Jubin, R. T. (2015). Recommend HIP Conditions for AgZ. FCRD-MRWFD-2015-000423, ORNL/SPR-2015/503. Oak Ridge, TN: Oak Ridge National Laboratory.
Bukhtiyarov, V. I., Kaichev, V. V., and Prosvirin, I. P. (1999). Oxygen adsorption on Ag(111): X-Ray photoelectron spectroscopy (XPS), angular dependent x-ray photoelectron spectroscopy (ADXPS) and temperature-programmed desorption (TPD) studies. J. Chem. Phys. 111 (5), 2169–2175. doi:10.1063/1.479488
Campbell, C. T. (1985). Atomic and molecular oxygen adsorption on Ag(111). Surf. Sci. 157 (1), 43–60. doi:10.1016/0039-6028(85)90634-X
Cao, C., Chong, S., Thirion, L., Mauro, J. C., McCloy, J. S., and Goel, A. (2017). Wet chemical synthesis of apatite-based waste forms – a novel room temperature method for the immobilization of radioactive iodine. J. Mat. Chem. A 5 (27), 14331–14342. doi:10.1039/C7TA00230K
Carley, A. F., Davies, P. R., Roberts, M. W., Santra, A. K., and Thomas, K. K. (1998). Coadsorption of carbon monoxide and nitric oxide at Ag(111): Evidence for a CO-NO surface complex. Surf. Sci. 406 (1), L587–L591. doi:10.1016/S0039-6028(98)00141-1
Cheng, Q., Yang, W., Li, Z., Zhu, Q., Chu, T., He, D., et al. (2015). Adsorption of gaseous radioactive iodine by Ag/13X zeolite at high temperatures. J. Radioanal. Nucl. Chem. 303 (3), 1883–1889. doi:10.1007/s10967-014-3736-3
Chong, S., Riley, B. J., Kuang, W., and Olszta, M. J. (2021). Iodine capture with mechanically robust heat-treated Ag-Al-Si-O xerogel sorbents. ACS Omega 6 (17), 11628–11638. doi:10.1021/acsomega.1c00852
Garino, T. J., Nenoff, T. M., Krumhansl, J. L., and Rademacher, D. X. (2011). Low-temperature sintering Bi–Si–Zn-oxide glasses for use in either glass composite materials or core/shell 129I waste forms. J. Am. Ceram. Soc. 94 (8), 2412–2419. doi:10.1111/j.1551-2916.2011.04542.x
Garino, T. J., Nenoff, T. M., and Sava Gallis, D. F. (2016). Densified waste form and method for forming. Albuquerque, NM: Sandia Corporation, USA Patent No. US9343192B2 Application No. US9343192B2.
Jubin, R. T. (1979). A literature survey of methods to remove iodine from off-gas streams using solid sorbents. Oak Ridge, TN: Oak Ridge National Laboratory. ORNL/TM-6607.
Jubin, R. T., Jordan, J. A., and Bruffey, S. H. (2018). Testing of an iodine and tritium removal system for advanced tritium pretreatment off-gas. Oak Ridge, TN: Oak Ridge National Laboratory. NTRD-MRWFD-2018-000199, ORNL/SPR-2018/15.
Keller, J. U. (2005). “Adsorption isotherms,” in Gas adsorption equilibria: Experimental methods and adsorption isotherms. Editors J. Keller, and R. Staudt (New York, NY: Springer), 359–413. ch. 7.
Le Gallet, S., Campayo, L., Courtois, E., Hoffmann, S., Grin, Y., Bernard, F., et al. (2010). Spark plasma sintering of iodine-bearing apatite. J. Nucl. Mater. 400 (3), 251–256. doi:10.1016/j.jnucmat.2010.03.011
Maddrell, E. R., Vance, E. R., Grant, C., Aly, Z., Stopic, A., Palmer, T., et al. (2019). Silver iodide sodalite – wasteform/Hip canister interactions and aqueous durability. J. Nucl. Mater. 517, 71–79. doi:10.1016/j.jnucmat.2019.02.002
Maddrell, E. R., Vance, E. R., and Gregg, D. J. (2015). Capture of iodine from the vapour phase and immobilisation as sodalite. J. Nucl. Mater. 467, 271–279. doi:10.1016/j.jnucmat.2015.09.038
Maeck, W. J., and Pence, D. T. (1970). “Application of the metal zeolites to radioactive air cleaning problems,” in Proceedings of 11th AEC Air Cleaning Conference, 2. CONF-700816.
Maeck, W. J., Pence, D. T., and Keller, J. H. (1968). A highly efficient inorganic adsorber for airborne iodine species (silver zeolite development studies). Idaho Falls, ID: Idaho Nuclear Corporation - National Reactor Testing Station. IN-1224.
Matyáš, J., Fryxell, G. E., Busche, B. J., Wallace, K., and Fifield, L. S. (2011). “Functionalized silica aerogels: Advanced materials to capture and immobilize radioactive iodine,” in Proceedings of Ceram. Eng. Sci.: Ceram. Mater. Energy Appl. Editor H-T. Lin. (Wiley-The American Ceramic Society), 32, 23–33.
Matyáš, J., Ilton, E. S., and Kovařík, L. (2018). Silver-functionalized silica aerogel: Towards an understanding of aging on iodine sorption performance. RSC Adv. 8 (56), 31843–31852. doi:10.1039/C8RA05137B
Matyáš, J., Ilton, E. S., Lahiri, N., Li, X. S., and Silverstein, J. A. (2021a). Bismuth-functionalized silica aerogels for iodine capture. Richland, WA: Pacific Northwest National Laboratory. PNNL-32086.
Matyáš, J., Sannoh, S. E., and Li, X. S. (2021b). A preliminary Investigation of Coadsorption of Halogens on engineered Form of Ag0-aerogel. Richland, WA: Pacific Northwest National Laboratory. PNNL-31222.
Muhire, C., Tesfay Reda, A., Zhang, D., Xu, X., and Cui, C. (2022). An overview on metal oxide-based materials for iodine capture and storage. Chem. Eng. J. 431, 133816. doi:10.1016/j.cej.2021.133816
Outka, D. A., Madix, R. J., Fisher, G. B., and Dimaggio, C. (1987). The adsorption of nitrogen dioxide on the Ag(110) surface and formation of a surface nitrate. Surf. Sci. 179 (1), 1–24. doi:10.1016/0039-6028(87)90117-8
Pence, D. T., Duce, F. A., and Maeck, W. J. (1972). “Developments in the removal of airborne iodine species with metal substituted zeolites,” in Proceedings of 12th AEC Air Cleaning Conference, 720823. CONF.
Pence, D. T., Duce, F. A., and Maeck, W. J. (1970). “Study of the adsorption properties of metal zeolites for airborne iodine species,” in Proceedings of 11th AEC Air Cleaning Conference, 700816. CONF.
Reiser, J. T., Lawter, A. R., Avalos, N. A., Bonnett, J., Riley, B. J., Chong, S., et al. (2022). Review and experimental comparison of the durability of iodine waste forms in semi-dynamic leach testing. Chem. Eng. J. Adv. 11, 100300. doi:10.1016/j.ceja.2022.100300
Riley, B. J., Chong, S., and Beck, C. L. (2021). Iodine vapor reactions with pure metal wires at temperatures of 100-139 °C in air. Ind. Eng. Chem. Res. 60 (47), 17162–17173. doi:10.1021/acs.iecr.1c03902
Riley, B. J., Chong, S., Marcial, J., Lahiri, N., Bera, M. K., Lee, S., et al. (2022a). Silver-loaded xerogel nanostructures for iodine capture: A comparison of thiolated versus unthiolated sorbents. ACS Appl. Nano Mat. 5, 9478–9494. doi:10.1021/acsanm.2c01741
Riley, B. J., Chong, S., Olszta, M. J., and Peterson, J. A. (2020). Evaluation of getter metals in Na-Al-Si-O aerogels and xerogels for the capture of iodine gas. ACS Appl. Mat. Interfaces 12 (17), 19682–19692. doi:10.1021/acsami.0c03155
Riley, B. J., Chong, S., Schmid, J., Marcial, J., Nienhuis, E. T., Bera, M. K., et al. (2022b). Role of zeolite structural properties toward iodine capture: A head-to-head evaluation of framework type and chemical composition. ACS Appl. Mat. Interfaces 14 (16), 18439–18452. doi:10.1021/acsami.2c01179
Riley, B. J., Kroll, J. O., Peterson, J. A., Matyáš, J., Olszta, M. J., Li, X., et al. (2017). Silver-loaded aluminosilicate aerogels as iodine sorbents. ACS Appl. Mat. Interfaces 9 (38), 32907–32919. doi:10.1021/acsami.7b10290
Riley, B. J., Pierce, D., Chun, A, J., Matyáš, J., Lepry, W. C., Garn, T., et al. (2014). Polyacrylonitrile-chalcogel hybrid sorbents for radioiodine capture. Environ. Sci. Technol. 48 (10), 5832–5839. doi:10.1021/es405807w
Riley, B. J., Vienna, J. D., Strachan, D. M., McCloy, J. S., and Jerden, J. L. (2016). Materials and processes for the effective capture and immobilization of radioiodine: A review. J. Nucl. Mater. 470, 307–326. doi:10.1016/j.jnucmat.2015.11.038
Sava, D. F., Garino, T. J., and Nenoff, T. M. (2012). Iodine confinement into metal–organic frameworks (MOFs): Low-temperature sintering glasses to form novel glass composite material (GCM) alternative waste forms. Ind. Eng. Chem. Res. 51 (2), 614–620. doi:10.1021/ie200248g
Strachan, D. M., Chun, J., Matyas, J., Lepry, W., Riley, B. J., Ryan, J. V., et al. (2011). Summary report on the volatile radionuclide and immobilization research for FY2011 at PNNL. Richland, WA: Pacific Northwest National Laboratory. FCRD-SWF-2011-000378, PNNL-20807.
Tang, S., Choi, S., Nan, Y., and Tavlarides, L. L. (2021). Adsorption of methyl iodide on reduced silver-functionalized silica aerogel: Kinetics and modeling. AIChE J. 67 (4), e17137. doi:10.1002/aic.17137
Tesfay Reda, A., Pan, M., Zhang, D., and Xu, X. (2021). Bismuth-based materials for iodine capture and storage: A review. J. Environ. Chem. Eng. 9 (4), 105279. doi:10.1016/j.jece.2021.105279
Tian, Z., Chee, T.-S., Zhu, L., Duan, T., Zhang, X., Lei, L., et al. (2021). Comprehensive comparison of bismuth and silver functionalized nickel foam composites in capturing radioactive gaseous iodine. J. Hazard. Mat. 417, 125978. doi:10.1016/j.jhazmat.2021.125978
Wiechert, A. I., Ladshaw, A. P., Moon, J., Abney, C. W., Nan, Y., Choi, S., et al. (2020). Capture of iodine from nuclear-fuel-reprocessing off-gas: Influence of aging on a reduced silver mordenite adsorbent after exposure to NO/NO2. ACS Appl. Mat. Interfaces 12 (44), 49680–49693. doi:10.1021/acsami.0c15456
Yao, T., Lu, F., Sun, H., Wang, J., Ewing, R. C., and Lian, J. (2014). Bulk iodoapatite ceramic densified by spark plasma sintering with exceptional thermal stability. J. Am. Ceram. Soc. 97 (8), 2409–2412. doi:10.1111/jace.13101
Zemlyanov, D., and Schlögl, R. (2000). Effect of oxygen on NO adsorption on the Ag(111) surface: Evidence for a NO3,ads species. Surf. Sci. 470 (1), L20–L24. doi:10.1016/S0039-6028(00)00840-2
Keywords: iodine capture, iodine immobilization, iodine sorbent selection criteria, getter metal, chemisorption
Citation: Riley BJ and Carlson K (2022) Radioiodine sorbent selection criteria. Front. Chem. 10:969303. doi: 10.3389/fchem.2022.969303
Received: 14 June 2022; Accepted: 27 July 2022;
Published: 31 August 2022.
Edited by:
Luís D. Carlos, University of Aveiro, PortugalReviewed by:
Ali Saad, Shenzhen University, ChinaCopyright © 2022 Riley and Carlson. This is an open-access article distributed under the terms of the Creative Commons Attribution License (CC BY). The use, distribution or reproduction in other forums is permitted, provided the original author(s) and the copyright owner(s) are credited and that the original publication in this journal is cited, in accordance with accepted academic practice. No use, distribution or reproduction is permitted which does not comply with these terms.
*Correspondence: Brian J. Riley, brian.riley@pnnl.gov