- 1Department of Chemistry, Forman Christian College (A Chartered University), Lahore, Pakistan
- 2Department of Chemistry, School of Natural Sciences (SNS), National University of Science and Technology (NUST), Islamabad, Pakistan
- 3Centre of Excellence in Environmental Studies (CEES), King Abdulaziz University, Jeddah, Saudi Arabia
- 4Biology Department, College of Science, Taibah University, Al Madinah Al Munawarah, Saudi Arabia
- 5Department of Chemistry, University of Sahiwal, Sahiwal, Pakistan
- 6Department of Chemistry, Government Islamia College, Civil Lines, Lahore, Pakistan
- 7Department of Chemistry, The Islamia University of Bahawalpur, Bahawalpur, Pakistan
- 8Department of Chemistry, College of Science and Technology, Wenzhou-Kean University, Wenzhou, China
- 9Chemistry Department, Faculty of Science, King Khalid University, Abha, Saudi Arabia
- 10Biology Department, Faculty of Science, King Khalid University, Abha, Saudi Arabia
- 11Department of Semi Pilot Plant, Nuclear Materials Authority, El Maadi, Egypt
- 12Department of Chemistry, College of Science, Princess Nourah Bint Abdulrahman University, Riyadh, Saudi Arabia
- 13Department of Pharmaceutical Sciences, College of Pharmacy, AlMaarefa University, Riyadh, Saudi Arabia
Besides natural sunlight and expensive artificial lights, economical indoor white light can play a significant role in activating a catalyst for photocatalytic removal of organic toxins from contaminated water. In the current effort, CeO2 has been modified with Ni, Cu, and Fe through doping methodology to study the removal of 2-chlorophenol (2-CP) in the illumination of 70 W indoor LED white light. The absence of additional diffractions due to the dopants and few changes such as reduction in peaks’ height, minor peak shift at 2θ (28.525°) and peaks’ broadening in XRD patterns of modified CeO2 verifies the successful doping of CeO2. The solid-state absorption spectra revealed higher absorbance of Cu-doped CeO2 whereas a lower absorption response was observed for Ni-doped CeO2. An interesting observation regarding the lowering of indirect bandgap energy of Fe-doped CeO2 (∼2.7 eV) and an increase in Ni-doped CeO2 (∼3.0 eV) in comparison to pristine CeO2 (∼2.9 eV) was noticed. The process of e-– h+ recombination in the synthesized photocatalysts was also investigated through photoluminescence spectroscopy. The photocatalytic studies revealed the greater photocatalytic activity of Fe-doped CeO2 with a higher rate (∼3.9 × 10−3 min-1) among all other materials. Moreover, kinetic studies also revealed the validation of the Langmuir-Hinshelwood kinetic model (R2 = 0.9839) while removing 2-CP in the exposure of indoor light with a Fe-doped CeO2 photocatalyst. The XPS analysis revealed the existence of Fe3+, Cu2+ and Ni2+ core levels in doped CeO2. Using the agar well-diffusion method, the antifungal activity was assessed against the fungus M. fructicola and F. oxysporum. Compared to CeO2, Ni-doped CeO2, and Cu-doped CeO2 nanoparticles, the Fe-doped CeO2 nanoparticles have outstanding antifungal properties.
Introduction
A major environmental threat that humanity faces today is the contamination of water caused by hazardous chemicals released into the environment by industrial processes. Routine daily activities can cause hazardous materials to be introduced into natural water resources, such as aquifers, lakes, oceans, rivers, and groundwater aquifers. Continually introducing such contaminants into natural water can result in an alteration of the characteristic features of natural water as well as polluted water. This is extremely unsuitable for human health. Chemical pollutants like dyes for textiles, halogenated compounds, substituted phenols, insecticides, pesticides, weed killers, and herbicides are known to be carcinogenic and pose major health risks to humans (Anku et al., 2017; Samanta et al., 2019; Brillas and Garcia-Segura, 2020; Kanan et al., 2020; Rafiq et al., 2021). Among toxic organic pollutants- chlorophenols and nitrophenols, because of their stable structure, toxic and carcinogenic effects on the human being, have been a remaining area under concern (Chiou et al., 2008; Adewuyi et al., 2016; Umukoro et al., 2017; Sharma et al., 2019; Barakat et al., 2020). Therefore, it is highly desirable to remove these toxins effectively from the polluted water.
The structural properties of these phenols and their derivatives have made them difficult to remove from the polluted water by classical methods, i.e., activated carbon adsorption, chemical oxidation, and biological treatment. Because activated carbon adsorption leads to phase separation without degradation of injurious pollutants, the chemical oxidation method is unable to remove pollutants efficiently, and biological treatments are slow and pH and temperature dependent, while the advanced oxidation process seems to be better for effective removal of such sort of destructive pollutants using light (Eryılmaz and Genç, 2021; Ren et al., 2021; Saputera et al., 2021).
Advanced oxidation leads to the formation of highly reactive species which ultimately react with organic pollutants to degrade them successfully (Mazivila et al., 2019; Du and Zhou, 2021; Gallo-Cordova et al., 2021; Huang et al., 2021; Luo et al., 2021). Among advanced oxidation processes, photocatalytic degradation and mineralization are efficient methods of converting highly toxic organic pollutants to harmless species under ambient conditions using light and a photocatalyst through homogeneous or heterogenous photocatalysis. In addition, heterogeneous photocatalysis is considered advantageous over homogenous photocatalysis due to an easy retrievability of a catalyst from the reaction mixture and then the reusability of a catalyst (Gisbertz and Pieber, 2020). Following the concept of the advanced oxidation process, the heterogeneous photocatalytic reaction starts with the generation of H2O2, OH∙, O2∙-, HOO∙ and H+ species by the absorption of a photon having energy equal to or greater than the band gap energy of the photocatalyst which is being used (Loeb et al., 2019; Pandey et al., 2020; Serrà and Philippe, 2020; Danish et al., 2021). In addition, hydroxyl and superoxide anion radicals react with phenols, depending on the position and nature of substituent groups on ph enols, to degrade them into some oxygenate intermediates and consequently, these oxygenates mineralize to their respective harmless species (Qamar et al., 2017a; Qamar et al., 2017b; Alhogbi et al., 2020).
Metal oxide-assisted photocatalytic wastewater treatment is a relatively prospective subject and growing rapidly to remove hazardous pollutants from contaminated water. In this context, metallic oxide semiconductors such as TiO2, ZnO, Fe2O3, SnO2, WO3, Bi2O3, V2O5, Cu2O, NiO, etc. have been studied extensively both in artificial and natural light sources to acquire pollution free water (Ahmed et al., 2010; Oturan and Aaron, 2014; Wang et al., 2014; Qamar et al., 2015; Zangeneh et al., 2015; Aslam et al., 2018). However, exploration of a potential photocatalyst for the effective removal of toxic pollutants from the wastewater has not over yet and researchers are paying attention to study the photocatalytic properties of CeO2-based photocatalysts for the abatement of organic pollutants. Although, bare CeO2 has wide application in electrocatalysis, solar cells, fuel cells and photocatalysis due to its high chemical stability, low toxicity and greater oxygen storage capacity (Ma et al., 2019; Fauzi et al., 2022). However, its performance in photocatalysis is unsatisfactory due to greater photo excitons’ recombination, lower absorption cross-section of light spectrum and higher band gap (2.8–3.1 eV) energy (Ma et al., 2019; Fauzi et al., 2022). Therefore, it is a need to modify CeO2 in order to increase its utilization as a photocatalyst for removal of organic toxins. The unique thing of this study is the introduction of transition metals into CeO2 without significantly changing the cubic structure of CeO2 photocatalyst for the removal of 15 ppm 2-chlorophenol in the illumination of 70 W indoor white light.
Previously, researchers utilized expensive artificial light sources such as solar simulator, Hg and Xe lamps for photocatalytic degradation of various organic toxins using variety of photocatalysts (Tang et al., 2004; Hao et al., 2015; Aslam et al., 2016; Xu et al., 2017). In addition, a few studies are available for the photocatalytic removal of 2-CP using CeO2-based photocatalysts such as CeO2, g-C3N4(0.94)/CeO2(0.05)/Fe3O4(0.01), TiO2-CeO2-ZrO2 (Aslam et al., 2016; García-Hernández et al., 2019; Rashid et al., 2019). However, various combinations of CeO2 with metals, non-metals and photocatalysts such as Ag2CO3, rGO, CdS, AgBr, SrFe12O9, BiOCl, g-C3N4, Co3O4, etc., are reported for the photocatalytic removal of organic dyes, phenols and pharmaceutical ingredients (Ma et al., 2019; Yao et al., 2021; Fauzi et al., 2022). Moreover, literature reveals that a photocatalysis setup while using an economical 70 W indoor white light for the removal of 2-CP over the proposed composition of CeO2-based photocatalysts has not been reported before.
In this study, CeO2 has been synthesized using a co-precipitation methodology and its modification was executed through doping with transition metals, i.e., Fe, Ni and Cu because 3d transition metals are considered capable dopants in tuning the properties of CeO2 for catalysis applications (Yue and Zhang, 2009; Elias et al., 2014; Qi et al., 2019). The spectral response and bandgap of the synthesized materials were evaluated using UV-visible diffuse reflectance spectroscopy (UV-visible DRS) whereas photoluminescence (PL) fluorometer was used to investigate e-–h+ recombination. X-ray diffraction (XRD) and X-ray photoelectron spectroscopy (XPS) were used to evaluate the structural and chemical characterization of synthesized materials. The photocatalytic activity of the synthesized photocatalysts was studied for the removal of 2-chlorophenol (2-CP) the kinetics for the photodegradation of 2-CP were also investigated.
Experimental
Materials
Cerium (III) nitrate hexahydrate [Ce(NO3)3.6H2O, Sigma-Aldrich, ≥99%], iron (III) nitrate non-ahydrate [Fe(NO3)3.9H2O, Sigma-Aldrich, 99.95%], nickel (II) nitrate hexahydrate [Ni(NO3)2.6H2O, Sigma-Aldrich, ≥99%], copper (II) nitrate trihydrate [Cu(NO3)2.3H2O, Sigma-Aldrich, 99.99%], ethanol (C2H5OH, Sigma-Aldrich, ≥99.8%), nitric acid [HNO3, Sigma-Aldrich, 70%], acetone [CH3COCH3, Sigma-Aldrich], potassium hydroxide [KOH, Sigma-Aldrich, 99.5%] and triton X-100 [Tx-100, Sigma-Aldrich] were used without further purification for the synthesis of photocatalysts.
Synthesis of pristine CeO2
In a typical synthesis of CeO2, 30 g Ce(NO3)3.6H2O was dissolved in 100 mL distilled water with continuous stirring until the formation of a clear solution. After complete dissolution, 3 mL Triton X-100 was added to the clear solution under continuous stirring for 30 min at room temperature. To hydrolyze the solution, 0.1M potassium hydroxide (KOH) was added to the solution dropwise till the formation of yellow precipitate near pH 9 at 50°C. The mixture containing the yellow precipitate, surfactant and KOH content was washed several times with distilled water and then with ethanol/water (30:60) mixture to remove surfactant and basic contents present in the mixture until the mixture attained the neutral pH. The precipitate was separated from the reaction mixture through filtration and the obtained yellow precipitate was again washed with ethanol/water (30:60) mixture and dried overnight at 100°C in a vacuum oven and then calcined in a muffle furnace for 4 h at 400°C. The calcined material was ground using mortar and pestle to get fine powder of CeO2 photocatalyst.
Synthesis of modified CeO2
For the synthesis of Cu doped CeO2, 0.381 g Cu(NO3)2.3H2O and 30.681 g Ce(NO3)3.6H2O were dissolved separately in distilled water with continuous stirring at room temperature till the formation of clear solutions and marked solution A and B, respectively. Then, solution A was added slowly into the beaker having solution B with continuous stirring at 50°C for 10 min followed by the addition of 3 mL Triton X-100 under continuous stirring for 30 min. The mixture of both precursors and surfactant was then hydrolyzed with the slow addition of 0.1M KOH till the formation of precipitate at pH 9. The formed precipitate was separated through filtration, washed with water and ethanol/water (30:60) mixture, and dried overnight in a vacuum oven at 100 °C. The dried sample was then subjected to the muffle furnace for calcination at 400°C for 4 h. The calcined material was ground using mortar and pestle to get fine powder of Cu doped CeO2 photocatalyst. The same procedure was applied for the synthesis of Ni-doped CeO2 and Fe-doped CeO2 except for the different amounts of precursors, e.g., 0.496 g Ni(NO3)2.6H2O and 0.723 g Fe(NO3)3.9H2O about the Ni and Fe contents, respectively for 30.681 g of Ce(NO3)3.6H2O. The synthesized photocatalytic materials were characterized by UV- visible diffuse reflectance spectroscopy (UV-Vis DRS), photoluminescence (PL), X-ray Diffraction (XRD), scanning electron microscopy (SEM) and X-ray photoelectron spectroscopy (XPS).
Photocatalytic studies
The photocatalytic performance of transition metal doped CeO2 was studied for the removal of 15 ppm 2-chlorophenol under the illumination of 70 W indoor white light (800 × 102 lx) at room temperature. In this context, the synthetic wastewater containing 15 ppm 2-chlorophenol was prepared in the laboratory, and the dose of the photocatalyst was optimized by exposing the suspension having 100 mL of pollutant with varying amounts of CeO2 photocatalyst (50, 100, 150, and 200 mg) in a glass reactor of 14 cm (diameter) and 2 cm (height) to the indoor white light for 3 h and the samples were collected and filtered using 0.20 µm syringe filter. The filtered samples were then subjected to UV-visible spectrophotometer for the monitoring of photodegradation of 2-CP and 100 mg of the photocatalyst was found to be the optimum dose of catalyst in 100 mL of pollutant. The optimized amount, i.e., 100 mg/100 mL of all four photocatalysts was further used for the evaluation of their photocatalytic degradation efficiency in removing 2-CP under the exposure of 70 W indoor LED white light (800 × 102 lx, 400–800 nm, Opple Lighting Co., Ltd. China) at room temperature and samples were collected after 30, 60, 90, 120, 180 and 240 min and filter using 0.20 µm syringe filter for UV-visible spectrophotometric analysis to evaluate the kinetic of photodegradation.
To study the removal of the target pollutant from the polluted water, the samples were collected using a syringe filter from the suspension exposed to the light after a regular interval and were subjected to UV-visible spectrophotometer for the determination of the concentration of the removed pollutant which ultimately led to the % removal of target pollutant by the photocatalyst under the exposure of light using the following equation.
Here, Co is the pollutant initial concentration whereas Ct is the concentration of the pollutant after exposure time “t.” Moreover, the validity of Langmuir Hinshelwood (L-H) kinetic models was also studied using the following equation to evaluate the kinetics of photocatalytic removal of organic pollutants under exposure to light.
Here, k is the rate constant which was determined from the slope by plotting ln
Results and discussion
The solid-state absorption response of the pristine CeO2 compared to the modified CeO2 is presented in Figure 1A in UV (200–400 nm) as well as visible (400–800 nm) regions of the spectrum. Wherein, Cu-doped CeO2 shows higher absorbance in both UV and visible regions than other synthesized materials. Interestingly, Cu-doped CeO2 and Fe-doped CeO2 showed higher absorbance of visible light than CeO2 and Ni-doped CeO2 whereas in the UV region of the spectrum only Cu-doped CeO2 presented higher absorbance than other photocatalysts. So, the insertion of Cu and Fe content into the structure of CeO2 leads to an increase in the spectral response of CeO2 whereas the same amount of Ni content has a detrimental effect on the spectral response of the pristine CeO2. Moreover, the response of these photocatalysts in reflecting the light spectrum has also been explored in Figure 1B. Wherein, lower reflectance (%) was noticed for CeO2 having copper contents followed by Fe and Ni contents in the lower energy region of the spectrum. This higher absorbance and lower reflectance (%) were attributed to the higher absorbance ability of copper content present in the structure of CeO2 as compared to Fe and Ni.
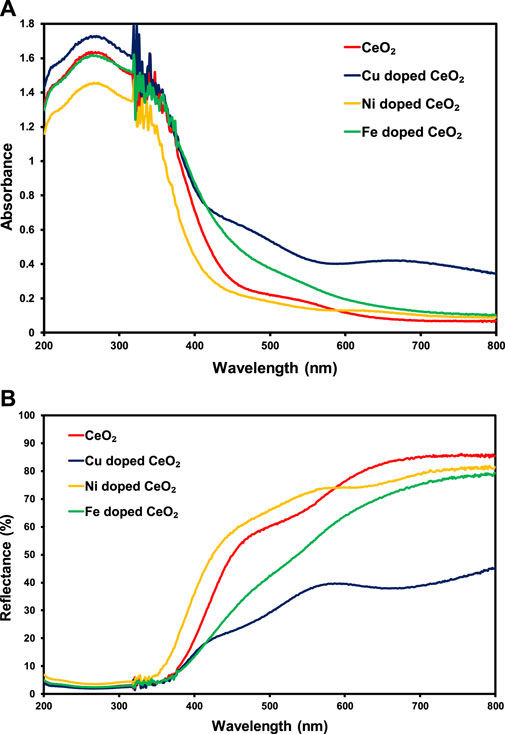
FIGURE 1. The comparison of solid-state (A) absorption and (B) reflectance (%) spectra of pristine CeO2 and doped CeO2.
The direct and indirect band gap evaluations (Figures 2A, B) reveal a lowering in the band gap energy of Cu and Fe-doped CeO2 photocatalysts as compared to pristine CeO2 was noticed which may be attributed to the shifting of conduction band edges to lower energy due to the addition of Cu and Fe contents in the structure of CeO2 (Yue and Zhang, 2009; Qi et al., 2019). Whereas a significant increase in the band gap energy of Ni-doped CeO2 was observed. The direct and indirect band gaps of the pristine CeO2 were noticed at ∼3.0 and ∼2.9 eV, respectively which have good agreement with the literature values (Aslam et al., 2016). Moreover, the direct band gap energies found for Cu, Fe, and Ni-doped CeO2 photocatalysts are ∼2.85, ∼2.8, and ∼3.2 eV whereas evaluated indirect band gap energies are ∼2.78, ∼2.7, and ∼3.0 eV, respectively as shown in Figures 2A, B. A greater decrease in the band gap energy was noticed for Fe doped CeO2 as compared to other photocatalysts which reveal that this photocatalyst requires lower energy as compared to pristine and other modified photocatalysts to make good use of excitons for the removal of organic toxins under the illumination of light.
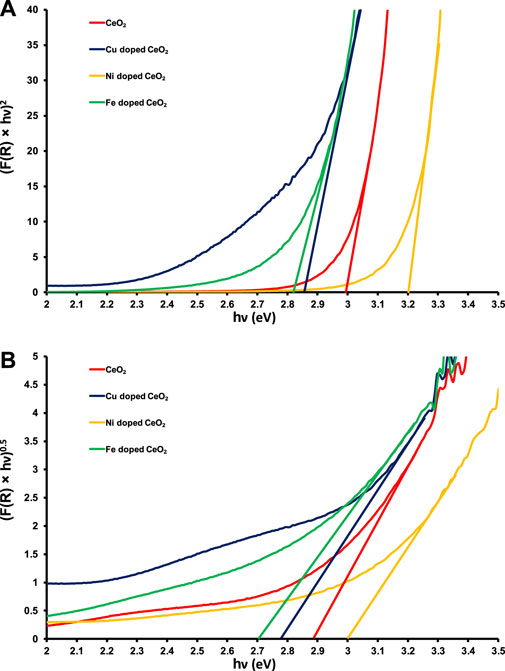
FIGURE 2. The graphical evaluations of (A) direct and (B) indirect band gaps of pristine and doped CeO2.
Photoluminescence analysis is a promising technique to study the emission intensity of the excited electron under the illumination of light which ultimately leads to the study of e-–h+ recombination process. Figure 3 depicts the comparison of photoluminescence spectra of pristine and doped CeO2 photocatalysts, wherein a significantly lower emission intensity was noticed for Cu and Ni-doped CeO2 as compared to unmodified CeO2. The relative decrease in emission intensities of the Cu and Fe doped CeO2 as compared to CeO2 is strong evidence for lowering e-–h+ recombination rate than pristine CeO2 which favors the photocatalytic degradation of organic toxins. Moreover, the decrease in strong emission bands around 470 nm is attributed to the trapping of photoexcitons by the surface defects generated due to the insertion of Cu or Fe into the structure of CeO2 (George et al., 2020).
The comparison of x-ray diffraction patterns of the modified CeO2 with pristine CeO2 is presented in Figure 4 and the cubic phase of the synthesized CeO2 was confirmed by matching diffractions planes (111), (200), (220), (311), (222), (400), (331), and (420) with literature and JCPDS 34-0394 (Aslam et al., 2016). The absence of additional diffraction peaks related to the dopant entities in the respective diffraction patterns verifies the successful doping of CeO2 with Cu, Ni, and Fe. Moreover, few changes such as reduction in few peaks’ height, minor peak shift at 2θ (28.525°) and peaks’ broadening in XRD patterns of modified CeO2 as compared to pristine CeO2 also favour the introduction of dopants in CeO2 (Kumar et al., 2010). Moreover, the successful insertion of dopants into CeO2 without significantly altering its structure were also evident from the micrographs as shown in Figure 5. The average crystallite size of the photocatalytic materials was calculated using a high-intensity diffraction peak at 2θ (28.525°) with the help of the Debye-Scherrer equation. The calculated crystallite sizes were 9.76, 3.46, 4.97, and 8.28 nm for pristine CeO2, Fe doped CeO2, Cu doped CeO2, and Ni doped CeO2, respectively. Moreover, a significant difference in doped CeO2 as compared to unmodified CeO2 were also reported in literature which support the changes in crystallite sizes of Fe and Cu doped CeO2 (Yue and Zhang, 2009; Kumar et al., 2010; Qi et al., 2019).
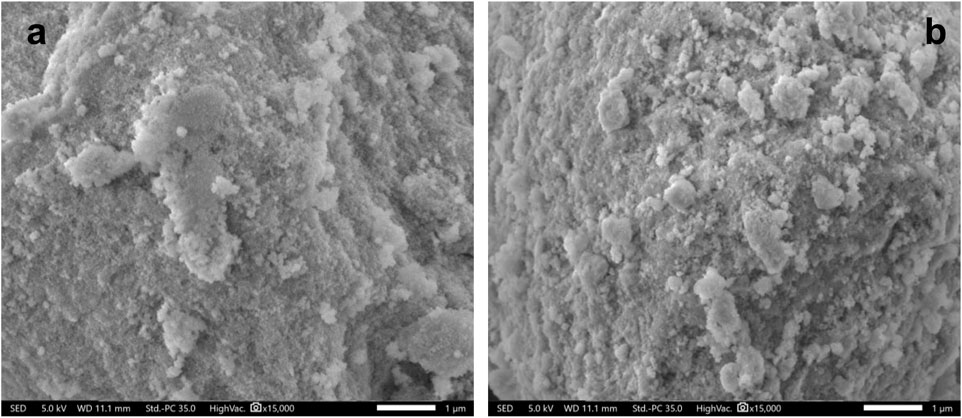
FIGURE 5. The comparison of scanning electron micrographs of (A) pristine CeO2 and (B) Fe doped CeO2.
As this study was designed to investigate the effect of transition metal dopants on the photocatalytic performance of CeO2 under the illumination of indoor white light for the degradation of 15 ppm 2−CP. Before the exposure of suspension containing photocatalyst and 2-CP to light as mentioned in the experimental section, the suspension was kept in dark for 30 min to establish an equilibrium between pollutant and catalyst. The photolysis of 2-CP was also evaluated by recording the absorption spectrum of the substrate after 240 min of light exposure without the presence of a photocatalyst. The amount of photocatalyst was also optimized (100 mg of photocatalyst) while studying the photocatalytic removal of 15 ppm 2-CP with varying doses of CeO2 catalyst under the illumination of indoor white light. The comparison of absorption spectra of photocatalytic removal of 2-CP over pristine CeO2, Fe doped CeO2, Cu doped CeO2, and Ni-doped CeO2 under the illumination of indoor white light (800 × 102 lx) is provided in Figure 6, respectively at different exposure time. Whereas the photocatalytic degradation (%) of 2-CP at different exposure over the pristine and modified CeO2 photocatalysts is given in Figure 7, respectively and highest photodegradation (∼ 65%) was noticed for Fe doped CeO2 followed by Cu doped CeO2 (∼ 60%), pure CeO2 (∼59%) and Ni doped CeO2 (∼45%) under the exposure of white light after 240 min of exposure. The decreasing trend of removal efficiency (%) of the photocatalysts is given below.
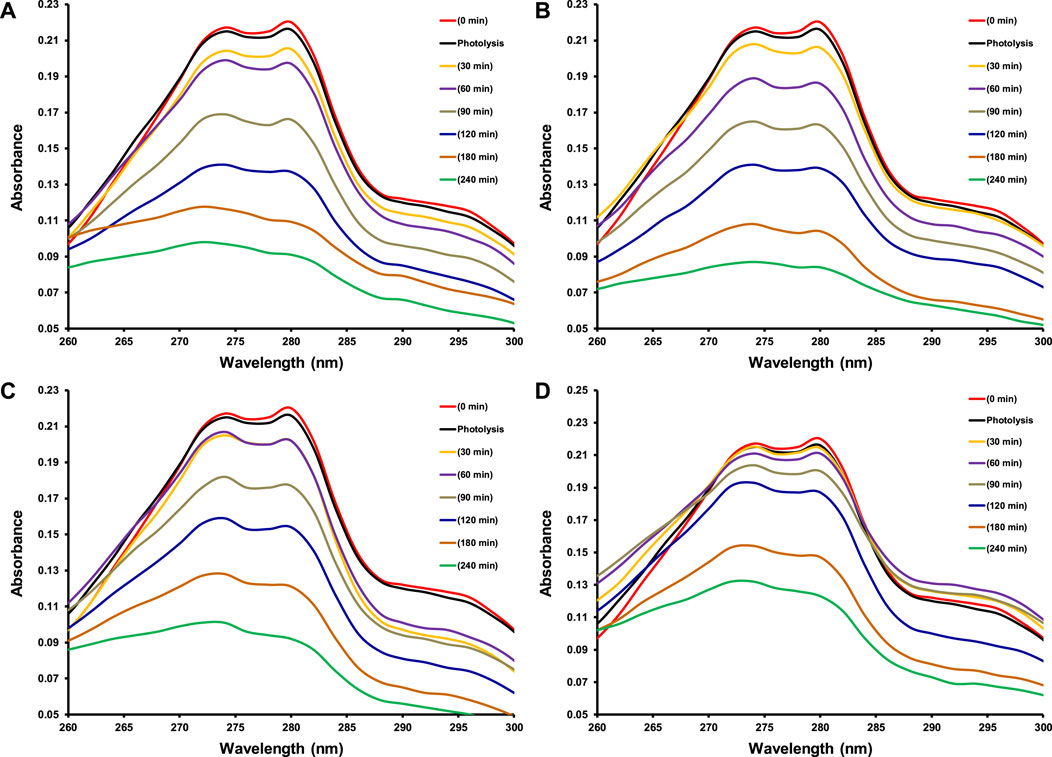
FIGURE 6. The comparison of absorption spectra for photocatalytic degradation of 15 ppm 2-CP over (A) CeO2 (B) Fe doped CeO2 (C) Cu doped CeO2 and (D) Ni doped CeO2 at different intervals of time under the illumination of indoor white light (800 × 102 lx).
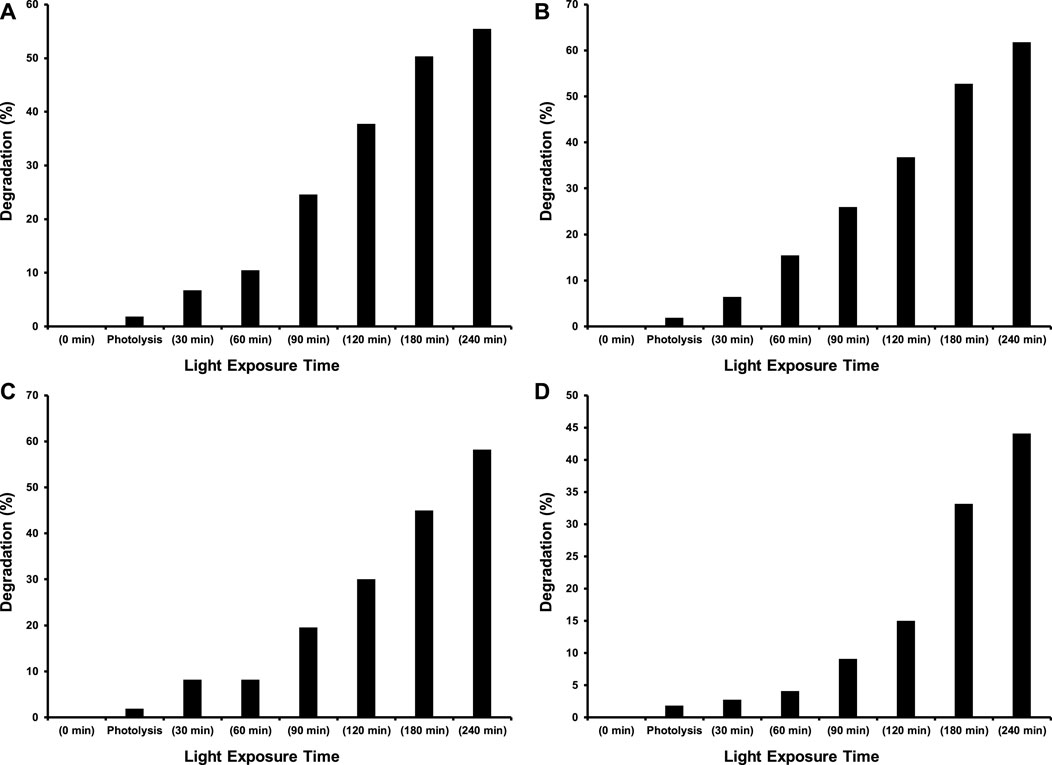
FIGURE 7. The comparison of % degradation of 15 ppm 2-CP over (A) CeO2 (B) Fe doped CeO2 (C) Cu doped CeO2 and (D) Ni doped CeO2 at different intervals of time under the illumination of indoor white light (800 × 102 lx).
Moreover, the rate of photodegradation of 15 ppm 2-CP over synthesized photocatalysts was also investigated and higher photocatalytic removal efficiency with rate constant (k = 3.9 × 10−3 min-1) was observed by Fe doped CeO2 than others as shown in Figure 8. The calculated bandgap energy values as shown in Figure 2 also support the possible higher photodegradation efficiency of Fe-doped CeO2 due to its lower bandgap energy than CeO2. Moreover, a lower photo-excitons’ recombination in PL spectra presented by Fe-doped CeO2 also arguments its higher removal efficiency due to the possible charge transferability for the generation of ROS. The decreasing trend of rate of removal of 2-CP by different photocatalysts is provided below.
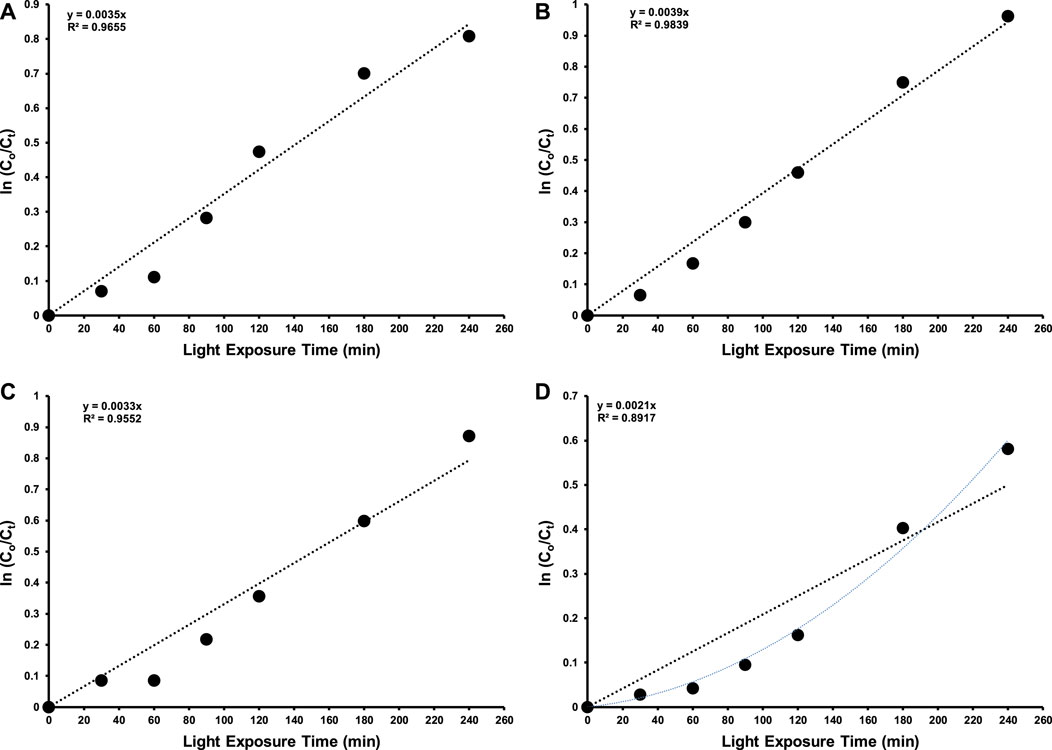
FIGURE 8. Rate of removal of 15 ppm 2-CP over (A) CeO2 (B) Fe doped CeO2 (C) Cu doped CeO2 and (D) Ni doped CeO2 at different intervals of time under the illumination of indoor white light (800 × 102 lx).
The kinetic study also reveals that the photocatalytic removal of 2-CP by Fe doped CeO2, pure CeO2, Cu doped CeO2 followed Langmuir Hinshelwood (L-H) kinetic model whereas the photocatalytic removal of 2-CP by Ni doped CeO2 did not follow Langmuir Hinshelwood (L-H) kinetic model. Previously, Zhang et al. (2022) reported a decrease in degradation rate of 2-CP removal in the illumination of 5W LED white light as compared to 300 W Xe arc lamp. In another study, BiFeO3/Bi2Fe4O9 heterojunctions were able to remove 95% of the 2-CP in the exposure of 150W LED white light (Wang et al., 2022).
In this study, x-ray photoelectron spectroscopy (XPS) was also carried out to investigate the chemical and electronic states of the elements in modified materials as shown in Figure 9. The presence of Ce3d3/2, Ce3d5/2, Fe2p1/2, Fe2p3/2, Cu2p1/2, Cu2p3/2, Ni2p1/2, Ni2p3/2 core levels and O1s levels in the synthesized photocatalysts can be seen in Figure 9, which confirm the existence of dopants (Fe3+, Cu2+ and Ni2+) in doped CeO2 as shown in inset figures. Moreover, the effect of dopants was also verified by the appearance of peak correspond to O1s level at different binding energies which ultimately confirm the different environment of oxygen.
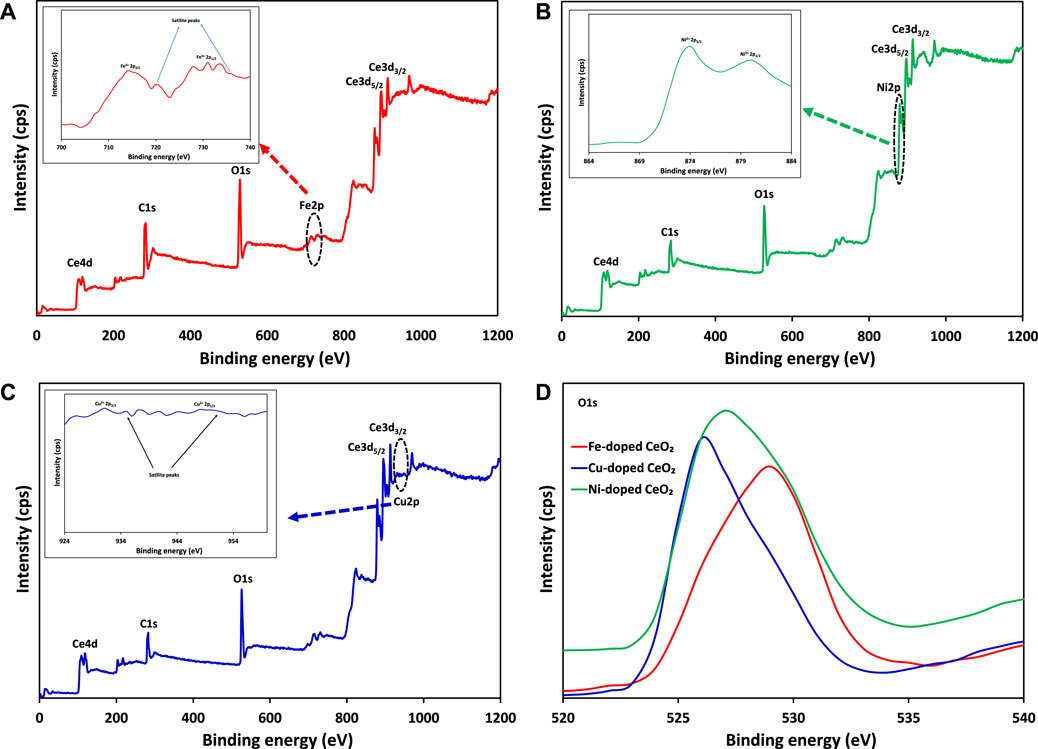
FIGURE 9. The comparison of XPS survey scan of (A) Fe-doped CeO2 (B) Ni-doped CeO2 (C) Cu-doped CeO2 and (D) O1s levels of modified CeO2 photocatalysts.
Antifungal activity
Using the agar well-diffusion method and amphotericin B as a reference, CeO2, Fe-doped CeO2, Ni-doped CeO2, and Cu-doped CeO2 nanoparticles were further assessed for their antifungal activity against M. fructicola and F. oxysporum. Table 1 provides a summary of the outcomes. According to the antifungal activity data (Table 1), Fe-doped CeO2 nanoparticles exhibit greater toxicity when compared to CeO2, Ni-doped CeO2, and Cu-doped CeO2 nanoparticles, with zone inhibition values of 25.3 and 23.1 mm. Due to their small particle size, the Fe-doped CeO2 nanoparticles easily pass through the fungal cell membrane, attach to functional protein groups as well as substances that contain phosphorus and sulphur, including DNA, and ultimately result in fungal cell death. The improved antifungal impact was caused by the synergistic interaction of Fe-doped CeO2 nanoparticles and decreased size.
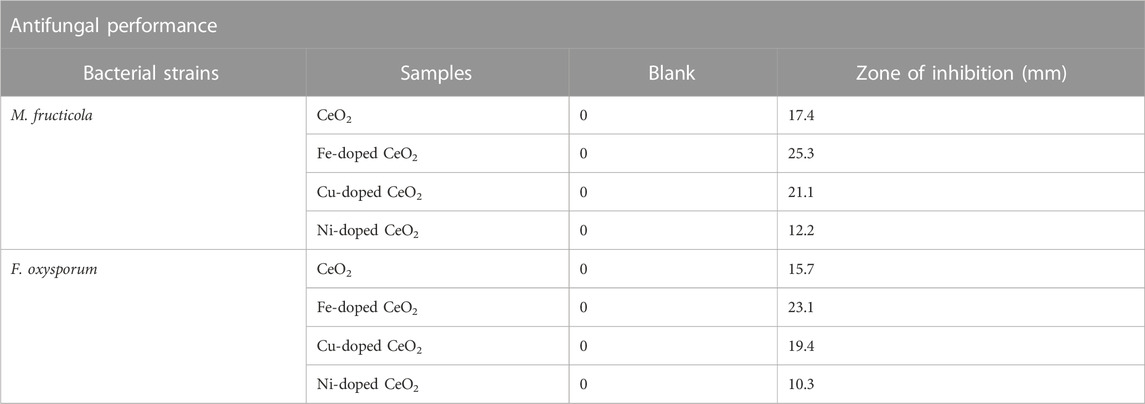
TABLE 1. Agar Well cut diffusion method zone of inhibition for the antifungal action of CeO2, Fe-doped CeO2, Ni-doped CeO2 and Cu-doped CeO2.
Conclusion
In conclusion, cubic CeO2 was doped successfully with Fe, Cu and Ni through co-precipitation method followed by calcination at 400°C for 4 h. The crystallite sizes were reduced from 9.76 to 3.46 nm by incorporating 3d transition metals into CeO2. Solid-state absorption analysis revealed a better spectral response and lower bandgap energy of Fe and Cu-doped CeO2 than unmodified CeO2 whereas increase in bandgap energy was noticed for Ni-doped CeO2. Moreover, the insertion of Cu and Fe into CeO2 suppressed its e-–h+ recombination process by generating trapping sites for photo-excitons. Among the synthesized photocatalysts in this study, Fe-doped CeO2 is excellent for the removal of 2-CP in the illumination of 70 W indoor LED white light.
Data availability statement
The original contributions presented in the study are included in the article/supplementary material, further inquiries can be directed to the corresponding authors.
Author contributions
MQ: Conception, design of study, writing-original draft preparation. SI: Interpret the data, performed major experimental works, writing-original draft preparation and editing. MA: He wrote the antifungal analysis part in the manuscript, interpreted the data and critical revision. AA: He wrote the UV-vis application part in the manuscript, interpreted the data and critical revision. ABi: Conception, performed dye degradation analysis, acquisition of data, interpret the data. KR: She wrote the PL analysis part in the manuscript, interpreted the data and critical revision. HF: Performed dye degradation analysis, reviewed original manuscript and critical revision. TS: Reviewed original manuscript, and critical revision. ABa: He performed synthesis methodology. NA: Visualization of data, XRD analysis, funding acquisition, writing reviewing, and editing. HI: Conception, visualization of data, performed bandgap analysis, funding acquisition. RA: She wrote the SEM analysis part in the manuscript, funding acquisition, interpreted the data and critical revision. EE: Visualization of data, reviewed original manuscript and critical revision.
Funding
The authors extend their appreciation to the Deanship of Scientific Research at King Khalid University for supporting this work through research groups program under grant number RGP.2/334/44. The authors thank the Higher Education Commission, Pakistan and Department of Chemistry, Forman Christian College (A Chartered University) Lahore Pakistan for supporting this work by grant No. 21-1865/SRGP/R&D/HEC/2018. This research was funded by Princess Nourah bint Abdulrahman University Researchers Supporting Project number (PNURSP2023R316), Princess Nourah bint Abdulrahman University, Riyadh, Saudi Arabia. The authors extend their appreciation to the Research Center at AlMaarefa University for funding this work.
Acknowledgments
The authors extend their appreciation to the Deanship of Scientific Research at King Khalid University for supporting this work through research groups program under grant number RGP.2/334/44. The authors thank the Higher Education Commission, Pakistan and Department of Chemistry, Forman Christian College (A Chartered University) Lahore Pakistan for supporting this work by grant No. 21-1865/SRGP/R&D/HEC/2018. This research was funded by Princess Nourah bint Abdulrahman University Researchers Supporting Project number (PNURSP2023R316), Princess Nourah bint Abdulrahman University, Riyadh, Saudi Arabia. The authors extend their appreciation to the Research Center at AlMaarefa University for funding this work.
Conflict of interest
The authors declare that the research was conducted in the absence of any commercial or financial relationships that could be construed as a potential conflict of interest.
Publisher’s note
All claims expressed in this article are solely those of the authors and do not necessarily represent those of their affiliated organizations, or those of the publisher, the editors and the reviewers. Any product that may be evaluated in this article, or claim that may be made by its manufacturer, is not guaranteed or endorsed by the publisher.
References
Adewuyi, A., Göpfert, A., Adewuyi, O. A., and Wolff, T. (2016). Adsorption of 2-chlorophenol onto the surface of underutilized seed of adenopus breviflorus: A potential means of treating waste water. J. Environ. Chem. Eng. 4 (1), 664–672. doi:10.1016/j.jece.2015.12.012
Ahmed, S., Rasul, M., Martens, W. N., Brown, R., and Hashib, M. (2010). Heterogeneous photocatalytic degradation of phenols in wastewater: A review on current status and developments. Desalination 261 (1-2), 3–18. doi:10.1016/j.desal.2010.04.062
Alhogbi, B. G., Aslam, M., Hameed, A., and Qamar, M. T. (2020). The efficacy of Co3O4 loaded WO3 sheets for the enhanced photocatalytic removal of 2, 4, 6-trichlorophenol in natural sunlight exposure. J. Hazard. Mater. 397, 122835–122843. doi:10.1016/j.jhazmat.2020.122835
Anku, W. W., Mamo, M. A., and Govender, P. P. (2017). “Phenolic compounds in water: Sources, reactivity, toxicity and treatment methods,” in Phenolic Compounds - Natural Sources, Importance and Applications, 419–443. doi:10.5772/66927
Aslam, M., Qamar, M., Soomro, M. T., Ismail, I. M., Salah, N., Almeelbi, T., et al. (2016). The effect of sunlight induced surface defects on the photocatalytic activity of nanosized CeO2 for the degradation of phenol and its derivatives. Appl. Catal. B Environ. 180, 391–402. doi:10.1016/j.apcatb.2015.06.050
Aslam, M., Qamar, M. T., Ali, S., Rehman, A. U., Soomro, M., Ahmed, I., et al. (2018). Evaluation of SnO2 for sunlight photocatalytic decontamination of water. J. Environ. Manag. 217, 805–814. doi:10.1016/j.jenvman.2018.04.042
Barakat, M., Kumar, R., Rashid, J., Seliem, M. K., Al-Mur, B., and El-Shishtawy, R. M. (2020). A novel CuO–Cu2O/Ag–Ag3PO4 nanocomposite: Synthesis, characterization, and its application for 2-chlorophenol decontamination under visible light. J. Taiwan Inst. Chem. Eng. 115, 208–217. doi:10.1016/j.jtice.2020.10.030
Brillas, E., and Garcia-Segura, S. (2020). Benchmarking recent advances and innovative technology approaches of fenton, photo-fenton, electro-fenton, and related processes: A review on the relevance of phenol as model molecule. Sep. Purif. Technol. 237, 116337–116367. doi:10.1016/j.seppur.2019.116337
Chiou, C.-H., Wu, C.-Y., and Juang, R.-S. (2008). Photocatalytic degradation of phenol and m-nitrophenol using irradiated TiO2 in aqueous solutions. Sep. Purif. Technol. 62 (3), 559–564. doi:10.1016/j.seppur.2008.03.009
Danish, M. S. S., Estrella, L. L., Alemaida, I. M. A., Lisin, A., Moiseev, N., Ahmadi, M., et al. (2021). Photocatalytic applications of metal oxides for sustainable environmental remediation. Metals 11 (1), 80–104. doi:10.3390/met11010080
Du, X., and Zhou, M. (2021). Strategies to enhance catalytic performance of metal–organic frameworks in sulfate radical-based advanced oxidation processes for organic pollutants removal. Chem. Eng. J. 403, 126346–126361. doi:10.1016/j.cej.2020.126346
Elias, J. S., Risch, M., Giordano, L., Mansour, A. N., and Shao-Horn, Y. (2014). Structure, bonding, and catalytic activity of monodisperse, transition-metal-substituted CeO2 nanoparticles. J. Am. Chem. Soc. 136 (49), 17193–17200. doi:10.1021/ja509214d
Eryılmaz, C., and Genç, A. (2021). Review of treatment technologies for the removal of phenol from wastewaters. J. Water Chem. Technol. 43 (2), 145–154. doi:10.3103/s1063455x21020065
Fauzi, A., Jalil, A., Hassan, N., Aziz, F., Azami, M., Hussain, I., et al. (2022). A critical review on relationship of CeO2-based photocatalyst towards mechanistic degradation of organic pollutant. Chemosphere 286, 131651. doi:10.1016/j.chemosphere.2021.131651
Gallo-Cordova, A., Veintemillas-Verdaguer, S., Tartaj, P., Mazarío, E., Morales, M. d. P., and Ovejero, J. G. (2021). Engineering iron oxide nanocatalysts by a microwave-assisted polyol method for the magnetically induced degradation of organic pollutants. Nanomaterials 11 (4), 1052–1068. doi:10.3390/nano11041052
García-Hernández, L. E., Frías-Márquez, D. M., Pacheco-Sosa, J. G., Cervantes-Uribe, A., Arévalo-Pérez, J. C., Pérez-Vidal, H., et al. (2019). 2-Chlorophenol degradation by catalytic wet air oxidation using copper supported on TiO2-CeO2-ZrO2. Water Sci. Technol. 80 (5), 911–919. doi:10.2166/wst.2019.330
George, S. E., George, M., Alex, J., Joy, L. K., Aravind, A., Sajan, D., et al. (2020). Nonlinear optical and photocatalytic dye degradation of Co doped CeO2 nanostructures synthesized through a modified combustion technique. Ceram. Int. 46 (9), 13932–13940. doi:10.1016/j.ceramint.2020.02.189
Gisbertz, S., and Pieber, B. (2020). Heterogeneous photocatalysis in organic synthesis. ChemPhotoChem 4 (7), 454–475. doi:10.1002/cptc.202000137
Hao, C., Li, J., Zhang, Z., Ji, Y., Zhan, H., Xiao, F., et al. (2015). Enhancement of photocatalytic properties of TiO2 nanoparticles doped with CeO2 and supported on SiO2 for phenol degradation. Appl. Surf. Sci. 331, 17–26. doi:10.1016/j.apsusc.2015.01.069
Huang, B., Wu, Z., Zhou, H., Li, J., Zhou, C., Xiong, Z., et al. (2021). Recent advances in single-atom catalysts for advanced oxidation processes in water purification. J. Hazard. Mater. 412, 125253–125273. doi:10.1016/j.jhazmat.2021.125253
Kanan, S., Moyet, M. A., Arthur, R. B., and Patterson, H. H. (2020). Recent advances on TiO2-based photocatalysts toward the degradation of pesticides and major organic pollutants from water bodies. Catal. Rev. 62 (1), 1–65. doi:10.1080/01614940.2019.1613323
Kumar, S., Kim, Y. J., Koo, B., and Lee, C. G. (2010). Structural and magnetic properties of Ni doped CeO2 nanoparticles. J. Nanosci. Nanotechnol. 10 (11), 7204–7207. doi:10.1166/jnn.2010.2751
Loeb, S. K., Alvarez, P. J., Brame, J. A., Cates, E. L., Choi, W., Crittenden, J., et al. (2019). The technology horizon for photocatalytic water treatment: Sunrise or sunset? Environ. Sci. Technol. 53 (6), 2937–2947. doi:10.1021/acs.est.8b05041
Luo, H., Zeng, Y., He, D., and Pan, X. (2021). Application of iron-based materials in heterogeneous advanced oxidation processes for wastewater treatment: A review. Chem. Eng. J. 407, 127191–127213. doi:10.1016/j.cej.2020.127191
Ma, R., Zhang, S., Wen, T., Gu, P., Li, L., Zhao, G., et al. (2019). A critical review on visible-light-response CeO2-based photocatalysts with enhanced photooxidation of organic pollutants. Catal. today 335, 20–30. doi:10.1016/j.cattod.2018.11.016
Mazivila, S. J., Ricardo, I. A., Leitão, J. M., and da Silva, J. C. E. (2019). A review on advanced oxidation processes: From classical to new perspectives coupled to two-and multi-way calibration strategies to monitor degradation of contaminants in environmental samples. Trends Environ. Anal. Chem. 24, e00072–e00081. doi:10.1016/j.teac.2019.e00072
Oturan, M. A., and Aaron, J.-J. (2014). Advanced oxidation processes in water/wastewater treatment: Principles and applications. A review. Crit. Rev. Environ. Sci. Technol. 44 (23), 2577–2641. doi:10.1080/10643389.2013.829765
Pandey, S., Mandari, K. K., Kim, J., Kang, M., and Fosso-Kankeu, E. (2020). Recent advancement in visible-light-responsive photocatalysts in heterogeneous photocatalytic water treatment technology. Photocatal. Adv. Oxid. Process. wastewater Treat., 167–196. doi:10.1002/9781119631422.ch6
Qamar, M. T., Aslam, M., Ismail, I. M., Salah, N., and Hameed, A. (2015). Synthesis, characterization, and sunlight mediated photocatalytic activity of CuO coated ZnO for the removal of nitrophenols. ACS Appl. Mater. interfaces 7 (16), 8757–8769. doi:10.1021/acsami.5b01273
Qamar, M. T., Aslam, M., Rehan, Z., Soomro, M. T., Basahi, J. M., Ismail, I. M., et al. (2017). The influence of p-type Mn3O4 nanostructures on the photocatalytic activity of ZnO for the removal of bromo and chlorophenol in natural sunlight exposure. Appl. Catal. B Environ. 201, 105–118. doi:10.1016/j.apcatb.2016.08.004
Qamar, M., Aslam, M., Rehan, Z., Soomro, M., Basahi, J. M., Ismail, I. M., et al. (2017). The effect of Fe3+ based visible light receptive interfacial phases on the photocatalytic activity of ZnO for the removal of 2, 4-dichlorophenoxy acetic acid in natural sunlight exposure. Sep. Purif. Technol. 172, 512–528. doi:10.1016/j.seppur.2016.08.030
Qi, Y., Ye, J., Zhang, S., Tian, Q., Xu, N., Tian, P., et al. (2019). Controllable synthesis of transition metal ion-doped CeO2 micro/nanostructures for improving photocatalytic performance. J. Alloys Compd. 782, 780–788. doi:10.1016/j.jallcom.2018.12.111
Rafiq, A., Ikram, M., Ali, S., Niaz, F., Khan, M., Khan, Q., et al. (2021). Photocatalytic degradation of dyes using semiconductor photocatalysts to clean industrial water pollution. J. Indust. Eng. Chem. 2021, 1–18. doi:10.1016/j.jiec.2021.02.017
Rashid, J., Parveen, N., Iqbal, A., Awan, S. U., Iqbal, N., Talib, S. H., et al. (2019). Facile synthesis of g-C3N4 (0.94)/CeO2 (0.05)/Fe3O4 (0.01) nanosheets for DFT supported visible photocatalysis of 2-Chlorophenol. Sci. Rep. 9 (1), 10202. doi:10.1038/s41598-019-46544-7
Ren, G., Han, H., Wang, Y., Liu, S., Zhao, J., Meng, X., et al. (2021). Recent advances of photocatalytic application in water treatment: A review. Nanomaterials 11 (7), 1804–1825. doi:10.3390/nano11071804
Samanta, P., Desai, A. V., Let, S., and Ghosh, S. K. (2019). Advanced porous materials for sensing, capture and detoxification of organic pollutants toward water remediation. ACS Sustain. Chem. Eng. 7 (8), 7456–7478. doi:10.1021/acssuschemeng.9b00155
Saputera, W. H., Putrie, A. S., Esmailpour, A. A., Sasongko, D., Suendo, V., and Mukti, R. R. (2021). Technology advances in phenol removals: Current progress and future perspectives. Catalysts 11 (8), 998–1042. doi:10.3390/catal11080998
Serrà, A., and Philippe, L. (2020). Simple and scalable fabrication of hairy ZnO@ ZnS core@ shell Cu cables for continuous sunlight-driven photocatalytic water remediation. Chem. Eng. J. 401, 126164–126172. doi:10.1016/j.cej.2020.126164
Sharma, G., Kumar, A., Naushad, M., Sharma, S., Ghfar, A. A., Ahamad, T., et al. (2019). Graphene oxide supported La/Co/Ni trimetallic nano-scale systems for photocatalytic remediation of 2-chlorophenol. J. Mol. Liq. 294, 111605–111614. doi:10.1016/j.molliq.2019.111605
Tang, J., Zou, Z., and Ye, J. (2004). Efficient photocatalytic decomposition of organic contaminants over CaBi2O4 under visible-light irradiation. Angew. Chem. 116 (34), 4463–4466. doi:10.1002/anie.200353594
Umukoro, E. H., Peleyeju, M. G., Ngila, J. C., and Arotiba, O. A. (2017). Towards wastewater treatment: Photo-assisted electrochemical degradation of 2-nitrophenol and orange II dye at a tungsten trioxide-exfoliated graphite composite electrode. Chem. Eng. J. 317, 290–301. doi:10.1016/j.cej.2017.02.084
Wang, M., Ioccozia, J., Sun, L., Lin, C., and Lin, Z. (2014). Inorganic-modified semiconductor TiO2 nanotube arrays for photocatalysis. Energy & Environ. Sci. 7 (7), 2182–2202. doi:10.1039/c4ee00147h
Wang, Y., Tang, Y., Sun, J., Wu, X., Liang, H., Qu, Y., et al. (2022). BiFeO3/Bi2Fe4O9 S-scheme heterojunction hollow nanospheres for high-efficiency photocatalytic o-chlorophenol degradation. Appl. Catal. B Environ. 319, 121893. doi:10.1016/j.apcatb.2022.121893
Xu, J., Wang, Z., and Zhu, Y. (2017). Enhanced visible-light-driven photocatalytic disinfection performance and organic pollutant degradation activity of porous g-C3N4 nanosheets. ACS Appl. Mater. interfaces 9 (33), 27727–27735. doi:10.1021/acsami.7b07657
Yao, J., Gao, Z., Meng, Q., He, G., and Chen, H. (2021). One-step synthesis of reduced graphene oxide based ceric dioxide modified with cadmium sulfide (CeO2/CdS/RGO) heterojunction with enhanced sunlight-driven photocatalytic activity. J. Colloid Interface Sci. 594, 621–634. doi:10.1016/j.jcis.2021.03.034
Yue, L., and Zhang, X.-M. (2009). Structural characterization and photocatalytic behaviors of doped CeO2 nanoparticles. J. Alloys Compd. 475 (1-2), 702–705. doi:10.1016/j.jallcom.2008.07.096
Zangeneh, H., Zinatizadeh, A., Habibi, M., Akia, M., and Isa, M. H. (2015). Photocatalytic oxidation of organic dyes and pollutants in wastewater using different modified titanium dioxides: A comparative review. J. Indust. Eng. Chem. 26, 1–36. doi:10.1016/j.jiec.2014.10.043
Keywords: modified CeO2, band gap energy, photocatalytic removal, 2-chlorophenol, nanocompoiste, antifungal activity
Citation: Qamar MT, Iqbal S, Aslam M, Alhujaily A, Bilal A, Rizwan K, Farooq HMU, Sheikh TA, Bahadur A, Awwad NS, Ibrahium HA, Almufarij RS and Elkaeed EB (2023) Transition metal doped CeO2 for photocatalytic removal of 2-chlorophenol in the exposure of indoor white light and antifungal activity. Front. Chem. 11:1126171. doi: 10.3389/fchem.2023.1126171
Received: 17 December 2022; Accepted: 08 March 2023;
Published: 19 April 2023.
Edited by:
Sethumathavan Vadivel, Tokyo Institute of Technology, JapanReviewed by:
Bulelwa Ntsendwana, Mintek, South AfricaZuzanna Bielan, Institute of Fluid Flow Machinery (PAN), Poland
Copyright © 2023 Qamar, Iqbal, Aslam, Alhujaily, Bilal, Rizwan, Farooq, Sheikh, Bahadur, Awwad, Ibrahium, Almufarij and Elkaeed. This is an open-access article distributed under the terms of the Creative Commons Attribution License (CC BY). The use, distribution or reproduction in other forums is permitted, provided the original author(s) and the copyright owner(s) are credited and that the original publication in this journal is cited, in accordance with accepted academic practice. No use, distribution or reproduction is permitted which does not comply with these terms.
*Correspondence: Shahid Iqbal, c2hhaGlkLmkxNEB5YWhvby5jb20=; Ali Bahadur, YWJhaGFkdXJAd2t1LmVkdS5jbg==; Eslam B. Elkaeed, aWthZWVkQG1jc3QuZWR1LnNh