- 1College of Pharmacy, Gachon University, Incheon, Republic of Korea
- 2Gachon Pharmaceutical Research Institute, Gachon University, Incheon, Republic of Korea
- 3College of Pharmacy, Kangwon National University, Chuncheon, Gangwon-do, Republic of Korea
Quinazolines are a class of nitrogen-containing heterocyclic compounds with broad-spectrum of pharmacological activities. Transition-metal-catalyzed reactions have emerged as reliable and indispensable tools for the synthesis of pharmaceuticals. These reactions provide new entries into pharmaceutical ingredients of continuously increasing complexity, and catalysis with these metals has streamlined the synthesis of several marketed drugs. The last few decades have witnessed a tremendous outburst of transition-metal-catalyzed reactions for the construction of quinazoline scaffolds. In this review, the progress achieved in the synthesis of quinazolines under transition metal-catalyzed conditions are summarized and reports from 2010 to date are covered. This is presented along with the mechanistic insights of each representative methodology. The advantages, limitations, and future perspectives of synthesis of quinazolines through such reactions are also discussed.
1 Introduction
Nitrogen-containing heteroaromatic compounds are important components of pharmaceuticals, agrochemicals, dyes, and other functional materials (Michael, 2008). Quinazolines comprise a family of nitrogen-based bicyclic heteroarenes that are considered attractive targets by medicinal chemists as these motifs are the constituents of bioactive molecules that show antibacterial (Jafari et al., 2016), antiviral (Wan et al., 2015), anti-inflammatory (Chandrikaa et al., 2008), anticancer (Shagufta, 2017), anticoccidial (Ye et al., 2010), antimutagenic (Cakici et al., 2010), antileishmanial (Van Horn et al., 2014), antimalarial (Bule et al., 2017), antioxidant (Gilson et al., 2017), antiobesity (Sasmal et al., 2012), anti-tubercular (Kunes et al., 2000), neuroprotective (Zhang et al., 2018a), and hypertensive activities (Rahman et al., 2014). In fact, Vandetanib is the first drug approved by FDA for the treatment of medullar thyroid cancer (Chau and Haddad, 2013). Several antitumor drugs, e.g., erlotinib (Higgins et al., 2004) and gefitinib (Sordella et al., 2004) are currently used in the market. Lapatinib is an effective inhibitor for epidermal growth factor and is used in combination therapy for breast cancer (Johnston et al., 2009). Vasicine, a natural quinazoline alkaloid isolated from Adhatoda vasica, displays bronchodilatory activity both in vitro and in vivo (Gupta et al., 1977). Rutaecarpine (Tian et al., 2019) is another alkaloid which has been used to treat gastrointestinal disorders, inflammation, headache, and postpartum hemorrhage, and recently, 2-arylquinazolines were identified as novel inhibitors of HIV-1 capsid assembly (Machara et al., 2016). Prazosin (Rosini et al., 2003) is utilized to treat high blood pressure, anxiety, and panic disorder. Terazosin is another important drug on the market for the treatment of symptomatic benign prostatic hyperplasia (Figure 1) (Wilt et al., 2002; Anwarul Islam et al., 2005).
Additionally, the unique photochromic behavior of quinazoline scaffolds has been exploited to elucidate cellular signaling processes of epidermal growth factor receptor (Liu et al., 2018) (EFGR) and α1-adrenergic receptors (Ma et al., 2016). Furthermore, quinazolines have been identified as light-emitting materials for electronic devices (Lipunova et al., 2018). Moreover, quinazolines also serve as valuable intermediates for preparation of functionalized materials (Zhao et al., 2013). More than 70% of the proprietary drugs are composed of N-heterocycles, as reported in a survey (Based on annual sales of drugs in the year 2009, 2009). Owing to their wide application in the pharmaceutical field, several synthetic methods have been developed over the past few decades. Griess in 1869 reported the first example of the synthesis of quinazolines from cyanogen and anthranilic acid (Griess, 1869). Nevertheless, these classical and general approaches are mostly associated with harsh reaction conditions, limited substrate generality and availability of starting materials, multistep reaction sequences, requirement of pre-functionalized substrates and excess oxidants, and generation of auxiliary waste. Hence, the development of new and alternative methods that involve inexpensive and readily available starting materials is desirable. The transition-metal-catalyzed formation of N-heterocycles continues to be an active area of research because these metals easily lose and gain electrons, and most of them are malleable, ductile, easily available, and act as efficient catalysts. In organic transformations, transition-metal-catalyzed reactions have advantages such as mild reaction conditions and compatibility with an extensive range of functionalities. As a result, transition-metal-catalyzed approaches are deliberated most assiduously by activating the starting materials via coordination, ligand exchange/promotion, elimination, etc. The selectivity of these catalysts can be achieved by ligand alteration.
In recent years, reviews have been presented on the synthetic approaches and biological activity of quinazolines (Wang and Gao, 2013; Srivastava and Srivastava, 2015; Hameed et al., 2018; Auti et al., 2020; Faisal and Saeed, 2021). However, transition-metal-catalyzed synthesis of quinazolines has not been properly highlighted. After a thorough literature study, the transition-metal-catalyzed synthesis of quinazolines was achieved, as illustrated in Figure 2. This review is divided into three categories: A) first-row, B) second-row, and C) third-row transition-metal catalysts. This review highlights this topic by discussing the advantages, limitations, shortcomings, and mechanistic aspects of representative methods over the last decade (2010–2022) in a chronological manner. This is presented alongside the first reported examples of different catalysts or bond-formation reactions involved in the construction of quinazoline scaffolds (Figure 2).
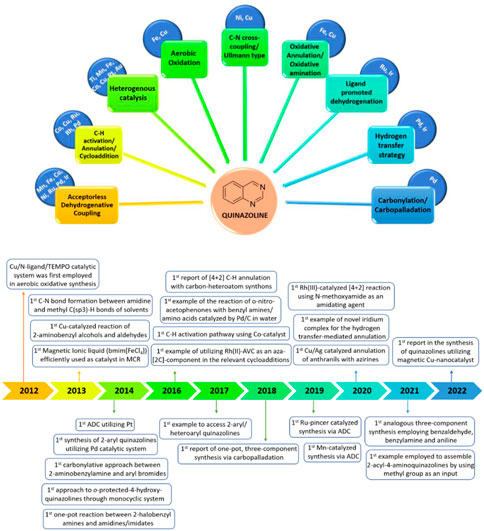
FIGURE 2. First-, second-, and third-row transition-metal-catalyzed synthesis of quinazolines and first reported examples involved in the synthesis of quinazolines (2012–2022).
2 First-row transition metal catalysts
2.1 Titanium
Titanium is the second most abundant transition metal and the ninth most abundant metal in the Earth’s crust. Ti plays a key role in the synthesis of fine chemicals, agrochemicals, and pharmaceuticals, and industrial processes. Ti catalysts are versatile, less toxic, inexpensive, and biocompatible. However, its use as a catalyst has been overlooked because of its oxophilic Lewis acidity, which renders titanium complexes less tolerant to functional groups than their transition-metal counterparts. Ti complexes facilitate excellent transformations such as reductive coupling (McMurry et al., 1978), radical reactions (Nugent and RajanBabu, 1988), hydroaminoalkylation (Luhning et al., 2017), and hydroamination (Janssen et al., 2010).
Shimizu et al. (2019) reported that the synthesis of iodoquinazolines 2 using titanium tetraiodide/trimethylsilyl iodide synergistically induced the iodination–cyclization of benzamides 1. 2-Aryl-4-iodoquinazolines 2 were synthesized from N-(4-chlorobenzyl)-N-(2-cyanoaryl)benzamides 1 (as a nitrogen source) in the presence of titanium tetraiodide and trimethylsilyl iodide in toluene under reflux conditions for 14 h, with 50%–85% yields (Scheme 1). (Hachiya and Shimizu, 2019) The key highlights of this protocol are as follows: benzamide 1 can be prepared from readily available benzonitrile derivatives and the iodine functionality can be applied for further transformations. The only drawback of this procedure is the formation of 1-chloro-4-(iodomethyl)benzene 4 as a by-product in every case.
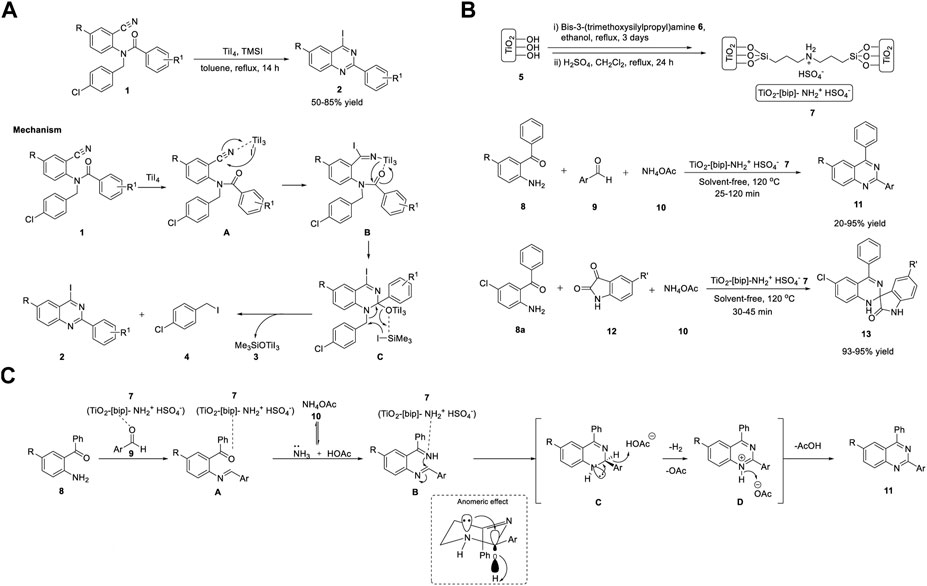
SCHEME 1. (A) Titanium tetraiodide and TMSI synergistically induced synthesis of 2-aryl-4-iodoquinazolines (B) Synthesis of quinazolines and spiro-quinazolines using Ti catalyst (C) Proposed Mechanism for the construction of quinazolines.
The proposed mechanism involves the coordination of titanium tetraiodide with the cyano group of N-(4-chlorobenzyl)- N-(2-cyanoaryl)benzamide 1 to generate Ti intermediate A. Intermediate B is formed by the nucleophilic addition of iodide ions to the cyano group of intermediate A. Subsequent intramolecular cyclization of intermediate B gives titanium alkoxide intermediate C, which then undergoes aromatization promoted by TMSI as an additive via the elimination of [(trimethylsilyl)oxy]titanium(IV) iodide 3 and 1-chloro-4-(iodomethyl)benzene 4 to afford 2-aryl-4-iodoquinazoline 2 (Scheme 1A). The aromatization step is driven by the synergistic effect of TiI4 and TMSI, and elimination is promoted by the increased nucleophilicity of iodide ions, resulting from the coordination of titanium alkoxide with the silyl group.
The combination of solid acids and ionic liquids possesses a wide range of benefits, such as easy handling, separation, and reuse procedures, and minimal usage of ionic liquids in reactions (Wight and Davis, 2002). One such example is nanoporous TiO2 containing an ionic liquid bridge (TiO2-[bip]-NH2+ HSO4−). Shirini et al. (2020) reported the Ti-catalyzed synthesis of quinazolines 11 and spiroquinazolines 13 via the reaction of o-aminobenzophenone 8, benzaldehyde 9, or isatin 12 and ammonium acetate 10 in the presence of catalyst 7 (TiO2-[bip]- NH2+ HSO4−) at 120°C under solvent-free conditions (Scheme 1B). (Mazloumi and Shirini, 2020) Catalyst 7 was prepared in two steps, starting from TiO2 5 and bis-3-(trimethoxysilylpropyl)amine 6 in ethanol (Scheme 1B).
The attractive features of this quinazoline synthesis procedure are high reaction rates, short reaction times, easy preparation and handling of catalysts, green experimental protocols, and inexpensive, reusable catalysts. However, electron-withdrawing and electron-donating groups such as -NO2, -OH, and -Cl in the ortho position of the aromatic aldehyde resulted in lower yields compared to para-substituted aldehydes. This is due to the steric hindrance effect, which constitutes a limitation in the scope of the substrate and requires further development.
Mechanistically, a Brønsted acidic titanium catalyst 7 (TiO2-[bip]-NH2+ HSO4−) activated the carbonyl group, and the reaction occurred in two steps. In the first step, the nucleophilic attack of o-aminobenzophenone 8 on the carbonyl carbon of aldehyde 9 and ammonia from ammonium acetate 10 attacks the carbonyl groups of o-aminobenzophenone 8 to generate intermediate A. In the second step, the ring closure and aromatization of intermediate A afforded quinazoline 11 via anomeric-based oxidation (Scheme 1C).
2.2 Manganese
Manganese is the third most abundant transition-metal in the Earth’s crust. Mn is unique as it is non-toxic and environmentally benign among the 3d-transition-metals. The presence of various catalytically active Mn complexes is due to the variable oxidation states of Mn. Mn catalysts are used in several organic transformations such as C-H activation (Liu and Ackermann, 2016), hydrosilylation (Yang and Wang, 2018), acceptorless dehydrogenative coupling (ADC) (Waiba and Maji, 2020), and hydrogen autotransfer (HA).
Heterogeneous catalysis has emerged as an important approach in cascade organic synthesis (Szollosi et al., 2015). Its advantages include easy catalyst separation, recyclability, and ligand-free conditions (Zhang et al., 2014a). Heterogenous catalysts are employed in the form of metal oxides, metals, and zeolites. Manganese oxide is one such example that is mostly used as a stoichiometric oxidant and rarely as an efficient catalyst (Pal et al., 2013).
Wang et al. (2015) developed a simple and cost-effective protocol for the synthesis of quinazolines using a robust and reusable α-MnO2 heterogeneous catalyst. The reaction of 2-aminobenzylamines 14 with alcohols 15 in the presence of α-MnO2-150, TBHP (tert-Butyl hydrogen peroxide), chlorobenzene at 80°C produced quinazolines 16 in 59%–91% yields (Scheme 2A). (Zhang et al., 2015) The highlights of this cascade synthesis are as follows: α-MnO2 can be separated by centrifugation and reused with no loss in catalytic activity, and various aromatic, aliphatic, and heterocyclic alcohols are well tolerated under the reaction conditions. The authors proposed that the TBHP radicals generated on the defective sites of α-MnO2 probably formed TBHP-MnO2 adducts and carried out the transformation. Usually, catalytic activity can be increased by heteroatom doping and thermal annealing (Tilley, 2008). However, in this case, both operations failed to give the desired results. Heteroatom doping of α-MnO2 (with Zn, Co, Ni, Mg, and Cu) resulted in reduced yields, and annealing at temperatures >150°C induced phase reconstruction and lower activity of the catalyst due to its variable valence and the structure of the manganese oxide catalyst, which limits the use of this catalyst.
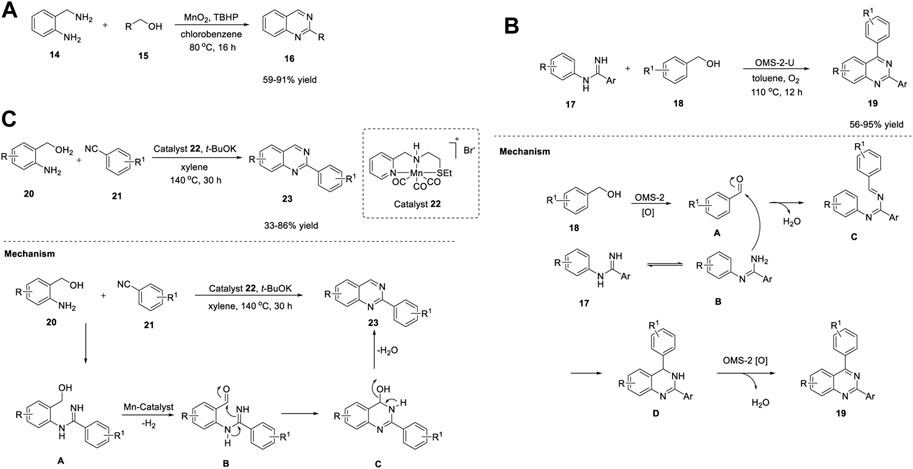
SCHEME 2. (A) Synthesis of quinazolines using MnO2 as catalyst (B) Synthesis of quinazolines using Manganese oxide OMS-2 catalyst (C) Synthesis of quinazolines using Manganese-Pincer complex.
Meng et al. (2018) reported manganese oxide octahedral molecular sieve urea (OMS-2-U)-catalyzed oxidative synthesis of quinazolines under ligand-free conditions. OMS-2-U was prepared through oxidation of Mn2+ or reduction of Mn4+ using urea as the additive. This heterogeneous oxidative synthesis was carried out by reacting N-phenyl amidines 17 with benzyl alcohols 18 using OMS-2-U as a catalyst in toluene at 110°C under O2 (Scheme 2B) (Li et al., 2018a).
A variety of substituted and unsubstituted amidines 17 underwent the reaction smoothly to afford quinazolines 19. Furthermore, benzyl alcohols 18 bearing electron-withdrawing groups gave better yields, presumably due to steric hindrance. However, electron-donating groups on benzyl alcohol 18 failed to form the desired product, which points to a shortcoming of this approach and highlights that further attention needs to be paid to the substrate scope. In addition, the enhanced surface area and crystallinity, easy separation by filtration, and reusability for at least four times makes this catalysis environmentally friendly.
OMS-2-U has excellent redox ability and serves as a catalyst in oxidation in combination with oxygen. The reaction mechanism suggested the oxidation of benzyl alcohol 18 into benzaldehyde A by OMS-2-U and O2. Subsequently, benzaldehyde A reacted with tautomeric amidine B using OMS-2-U to give intermediate C. Cyclization of C generated intermediate D. Finally, OMS-2-U/O2-catalyzed oxidative dehydrogenation of intermediate D led to the desired product 19 (Scheme 2B).
ADC reaction has emerged as a powerful tool for the sustainable synthesis of several organic molecules, since this strategy does not demand pre-functionalized substrates, hydrogen acceptors, or oxidants (Sivakumar et al., 2022). In this endeavor, Srimani et al. (2019) reported the first Mn-catalyzed synthesis of quinazolines 23 through the ADC. The replacement of expensive noble metal catalysts with non-toxic and earth-abundant Mn metal has made this an extremely advantageous strategy. The treatment of various 2-aminobenzyl alcohols 20 with benzonitriles 21 in the presence of Mn-pincer complex 22 bearing the NNS-ligand, potassium tertiary butoxide, in xylene at 140°C generated quinazolines 23 in 33%–86% yields (Scheme 2C) (Das et al., 2019).
The salient features of this strategy include: a) both electron-donating and electron-withdrawing groups in the aromatic nucleus of nitriles are well tolerated, and b) the process is atomically economical and sustainable as H2 and H2O are the only by-products of the reaction. Moreover, the evolved H2 can be used to convert styrene into ethylbenzene via Pd/C hydrogenation. However, the reaction with aliphatic nitriles such as valeronitrile yielded only 10% of the desired quinazoline 23, which is a major limitation of this protocol, and thus the protocol requires further developments in substrate scope. Mechanistically, the reaction proceeded by the dehydrogenation of 2-aminobenzyl alcohols 20 followed by condensation via amidine-type intermediate A. Concomitant C-N bond formation and removal of water resulted in the formation of quinazolines 23 (Scheme 2C).
Similarly, Balaraman et al. (2020) proposed Mn(I)-catalyzed-direct synthesis of quinazolines 26 through an ADC strategy. The reaction of 2-aminobenzyl alcohols 20 with primary amides 24 in the presence of Mn salt and simple phosphine-free NNN-tridentate ligand 25 in toluene at 130°C produced quinazolines 26 in 58%–81% yields (Scheme 7). (Mondal et al., 2020) Notably, most of the catalysts used in ADC reactions possess electron-rich phosphine ligands. However, their preparation is non-trivial and requires multistep procedures and handling in an inert atmosphere. Hence, this phosphine ligand-free protocol has gained attention for sustainable syntheses. The scope of the reaction was established by varying the substitution of the amides 24. Aliphatic, heteroaromatic, and halogen groups on aromatic ring were well tolerated. However, the electron-withdrawing groups on benzamide 24 and trans-cinnamide showed no reactivity under the same reaction conditions. The salient features of this protocol include: the reaction occurs even in the absence of oxidants, requires simple and abundantly available chemicals, and H2 and water are the only by-products of the reaction.
The tandem dehydrogenative annulation proceeded via the coordination of manganese salt Mn(CO)5Br and ligand L12 25 in the presence of potassium tert-butoxide to form the active Mn catalyst A. Subsequently, Mn-complex A activated the O-H bond of 2-aminobenzyl alcohol 24 via metal–ligand cooperation (MLC) and generated intermediate B. Next, intermediate B underwent β-H elimination to produce 2-aminobenzaldehyde 27 and intermediate C. Coupling of 2-aminobenzaldehyde 27 with primary amide 24 led to the formation of quinazoline 26 via the elimination of water molecules. In the last step, H2 gas liberated from intermediate C via metal-ligand cooperation regenerated active Mn catalyst A (Scheme 3A).
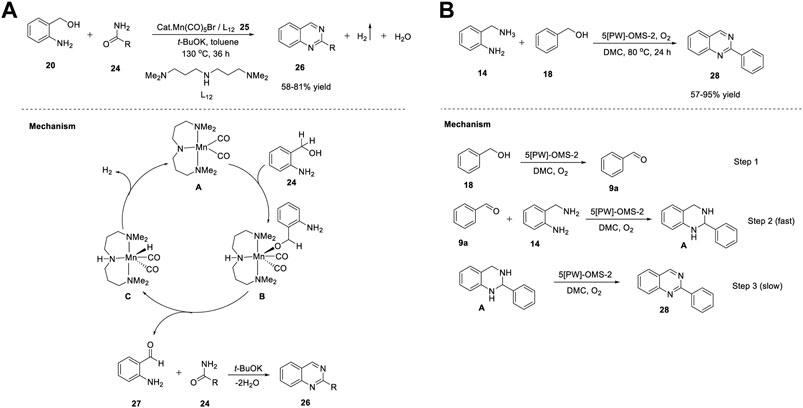
SCHEME 3. (A) Synthesis of quinazolines under Manganese catalysis (B) Synthesis of quinazolines using Manganese based nanocomposites OMS-2.
Recently, Liu et al. (2021) developed a series of sodium phosphotungstate-doped OMS-2 catalysts (5-[PW]-OMS-2) for the synthesis of 2-phenylquinazolines. Heterogeneous catalysts previously reported for the synthesis of quinazolines still have certain disadvantages such as the use of toxic solvents and peroxides as oxidants, difficulty in catalyst preparation, and the need for unstable and expensive aldehydes as starting substrates. To overcome these difficulties, a sodium phosphotungstate-modified manganese oxide catalyst was proposed, providing a new pathway for environmentally-friendly synthesis of quinazolines 28 in a one-pot procedure. The reaction of 2-aminobenzyl amines 14 with benzyl alcohols 18 in the presence of the ([PW]-OMS-2) catalyst and O2 as an oxidant in dimethyl carbonate at 80°C for 24 h afforded the desired products 28 in 57%–95% yields (Scheme 3B). (Yao et al., 2021) With O2 as the oxidant, broad functional group tolerance and easy catalyst preparation (simple wet impregnation method) are some of the advantages of the developed strategy. However, no reaction occurred with aliphatic alcohols and two other alcohols, which leaves a mark on this protocol.
The mechanistic studies depicted in Scheme 3B reveal the formation of quinazolines 28 in three steps. In the first step, benzyl alcohol 18 was converted into benzaldehyde 9a in the presence of (5-[PW]-OMS-2)/O2. Next, benzaldehyde 9a coupled with 2-aminobenzyl amine 14 and generated intermediate A. Finally, the dehydrogenation of intermediate A resulted in 2-phenyl quinazoline 28.
2.3 Iron
The second most abundant metal among all metals is iron, which is widely available in the Earth’s crust. Some superior characteristics of Fe are that it is non-toxic, cost-effective, environmentally benign, has a broad range of redox potentials, and tunes Lewis acidity based on ligands. These properties make Fe a promising catalyst candidate for organic transformations. Fe catalysts have been explored for addition (Li et al., 2018b), cross-coupling (Mako and Bayers, 2016), hydrogenation (Lane et al., 2019), substitution (Watile et al., 2019), and cycloaddition reactions (Hoyt et al., 2015).
In recent years, the multicomponent strategy has gained attention owing to its useful properties, such as that the reaction can be performed in a single step in one pot; use of ecofriendly, non-hazardous ionic liquids as solvents or catalysts; and use of solvent-free conditions sometimes (Hallett and Welton, 2011; Maeda et al., 2011; Choudhury and Singh, 2012). A new class of ionic liquids, namely, magnetic ionic liquids, has emerged as a powerful tool owing to its remarkable catalytic activity, ease of synthesis, and stability (Li et al., 2012). One such example is butylmethylimidazoliumtetrachloroferrate (bmim [FeCl4]) (Hayashi and Hamaguchi, 2004). The paramagnetic FeCl4− anions in the ionic liquid respond in the presence of a magnet and hence they are named as magnetic ionic liquid. Saha et al. (2013) presented an atom-efficient, solvent-free, high yielding, multicomponent green methodology to synthesize quinazolines in a one-pot reaction of 2-aminobenzophenone 8, benzaldehyde 9, and ammonium acetate 10 in the presence of magnetic ionic liquid (bmim[FeCl4]) at 40°C for 2.5 h. Magnetic ionic liquid was efficiently used as a catalyst in multicomponent reaction (MCR) for the first time to achieve quinazolines 29 in 86%–95% yields (Scheme 4A). (Panja and Saha, 2013) Shorter reaction time and unambiguous reusability of catalyst for at least four times highlight the merit of Fe catalyst in this MCR.
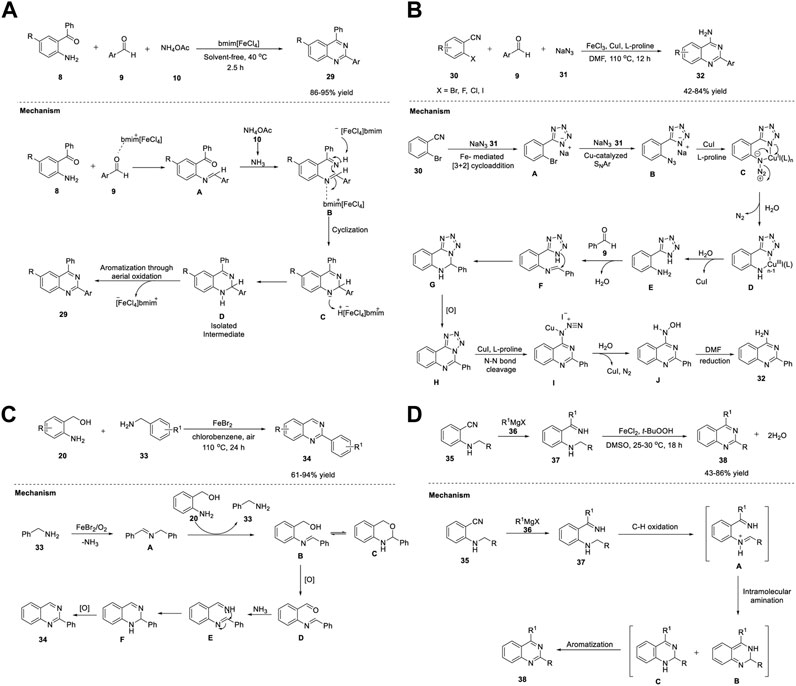
SCHEME 4. (A) Synthesis of quinazolines catalyzed by magnetic ionic liquid bmim [FeCl4] (B) Fe/Cu relay-catalyzed synthesis of quinazolines (C) FeBr2 catalyzed synthesis of quinazolines (D) Synthesis of quinazolines via iron-catalyzed C(sp3)-H oxidation.
Proposed tentative mechanism is shown in Scheme 4A. First, the counter cation bmim+ of the ionic liquid (bmim[FeCl4]) acts as a Lewis acid and catalyzes the condensation of the amino group of 8 with the carbonyl group of aldehyde 9 to generate intermediate A. Likewise, in the second step, condensation of the carbonyl group of intermediate A with ammonia, which is obtained from ammonium acetate 10, results in intermediate B. Subsequently, FeCl4, the counter anionic part of the catalyst, abstracts a proton from ketimine intermediate B to form intermediate C. In the last step, the catalyst is regenerated by proton abstraction from the previous step to produce a stable intermediate D, which then undergoes aerial oxidation to yield the desired quinazoline 29.
A remarkable work was put forth by Wu et al. (2015) on the synthesis of quinazolines via an Fe/Cu relay-catalyzed domino protocol. The reaction of o-halobenzonitriles 30, benzaldehydes 9, and sodium azide 31 (dual nitrogen source) in the presence of FeCl3 and CuI/L-proline in DMF at 110°C for 12 h resulted in 2-phenylquinazolin-4-amines 32 in 42%–84% yields (Scheme 4B) (Jia et al., 2015).
Commercially available starting materials and a wide substrate scope make this strategy advantageous. The reaction proceeds through the Fe-mediated [3 + 2] cycloaddition of o-bromobenzonitrile 30 to sodium azide 31 to generate intermediate A. Subsequent reaction of copper-catalyzed SNAr of intermediate A with sodium azide 31 gave intermediate B, a result of the ortho substituent effect. The coordination of azide 31 with copper, followed by electrocyclization with the release of N2 gave Cu(III) complex D, which then underwent reduction with the help of a water trace in DMF, resulting in intermediate F. The aniline intermediate F underwent condensation with benzaldehyde 9 to form imine intermediate G. Next, intramolecular nucleophilic attack of nitrogen on imine G followed by oxidative dehydrogenation led to intermediate H. Finally, the desired product 32 was obtained via a copper-catalyzed denitrogenation process (Scheme 4B).
Gopalaiah et al. (2017) reported for the first time the formation of 2-aryl/heteroaryl quinazolines 34 in 61%–94% yields from 2-aminobenzyl alcohols 20 and benzylamines 33 in the presence of FeBr2 in chlorobenzene at 110°C under aerobic oxidation for 24 h (Scheme 4C) (Gopalaiah et al., 2017).
The key highlights of this one-pot protocol are as follows: oxygen acts as an oxidant, 2 C=N and one C-N bonds form from two different amine partners, inexpensive Fe salt acts as a catalyst, broad functional group tolerance is observed, and there is a scale-up in gram quantity without affecting yield. However, no reaction occurred with aliphatic amines, indicating a limitation in the substrate scope; otherwise, this is an outstanding work.
A plausible mechanism illustrated in Scheme 4C involves the FeBr2 catalyzed-oxidative self-coupling of benzylamine 33 in the presence of O2, leading to benzylidenebenzylamine A with the simultaneous removal of ammonia. Subsequently, the transamination of imine A with 2-aminobenzyl alcohol 20 results in 2-(N-benzylidene)amino benzyl alcohol B. Intermediate B exists in tautomeric equilibrium with dihydrobenzoxazine C, a ring form. Upon oxidation, imine intermediate B converts into aldehyde D, which then reacts with ammonia generated from benzylamine 33 in the first step to form diamine E. Dihydroquinazoline F is formed by the cyclization of E. Aromatization of F affords quinazoline 34.
Chen et al. (2018) reported an efficient method for forming quinazolines 38 via Fe-catalyzed C (sp3)-H oxidation using t-BuOOH as the terminal oxidant (Scheme 4D) (Chen et al., 2018).
The optimized reaction conditions shown in Scheme 4D involve the treatment of easily available 2-alkylamino benzonitriles 35 with Grignard reagent 36 to form ketimine species 37, which then leads to quinazolines 38 (43%–86% yields) in the presence of FeCl2 and t-BuOOH in DMSO at 25°C for 18 h. Adopting this strategy, heterocycles such as furan, thiophene, and pyridine can be readily installed at the 2- position of quinazoline 38. The synthetic strategy involves the addition of Grignard or Organolithium reagent 36 to o-alkylamino benzonitriles 35 to form o-alkylamino N-H ketimines 37. Subsequently, C(sp3)-H oxidation of the α-proton of the aminoalkyl group generates imine or iminium species A. Ring closure via nucleophilic attack of iminium A then results in dihydroquinolines B and C. Aromatization through oxidation affords the desired quinazoline 38 (Scheme 4D).
Li et al. (2021) demonstrated the efficient synthesis of quinazolines 39 using the ADC strategy. Reaction of (2-aminophenyl)methanols 20 with benzamides 24 in the presence of FeCl2·4H2O, phenanthroline (ligand) in CsOH·H2O, and toluene at 130°C for 24 h delivered quinazolines 39 in 43%–92% yields (Scheme 13). (Zhang et al., 2021) A broad substrate scope and hydrogen and water as the only byproducts of the reaction are the merits of this approach. The proposed mechanism involves the one-pot dehydrogenation of (2-aminophenyl)methanol 20 into aldehyde A, followed by condensation with benzamide 24 in the presence of a base to produce quinazoline 39 (Scheme 5A).
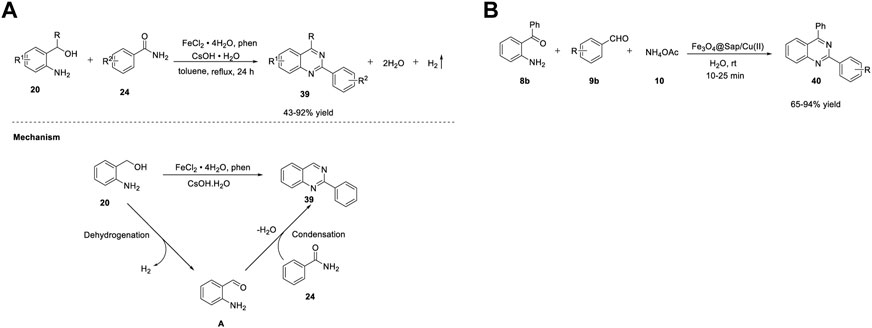
SCHEME 5. (A) Synthesis of quinazolines by Fe-catalyzed acceptorless dehydrogenative coupling (B) Synthesis of quinazolines using Fe3O4@Sap/Cu(II) nanocatalyst.
Heterogeneous catalysis has been used as a complementary protocol to homogenous catalysis. Kazemnejadi et al. (2021) developed a novel magnetically recoverable green nanocatalyst Fe3O4@Sap/Cu(II) for accessing quinazolines 40. Saponin belongs to a group of plant amphipathic glycosides with steroid/triterpenoid aglycones and is soluble in both water and fats; hence, it is a good choice in a phase transfer protocol (Güçlü-Üstündağ and Mazza, 2007). Immobilization of saponin as a green shell on Fe3O4 NPs (nanoparticles) as a magnetic solid support is a promising strategy. Furthermore, water is also a green solvent that has many advantages in organic synthesis such as easy product separation, high reaction rates, and cheap solvent. The one-pot cyclocondensation of aromatic aldehydes 9b with 2-aminobenzophenone 8b and ammonium acetate 10 catalyzed by Fe3O4@Sap/Cu(II) in water at room temperature for 10–25 min afforded quinazolines 40 in 65%–94% yields (Scheme 5B). (Kazemnejadi et al., 2021) High selectivity of substrates, shorter reaction times, and reusability of the catalyst for at least six times are the highlights of this protocol.
2.4 Cobalt
Cobalt is an inexpensive and less toxic metal that exhibits high chemoselectivity and variable oxidation states. Among the first-row transition metals, it is one of the most attractive candidates for catalysis. Hence, it is used to carry out various transformations, such as coupling reactions (Bottaro and Madsen, 2019), hydrofunctionalization (Yang et al., 2019a), hydrogenation (Viereck et al., 2020), and cycloaddition (Ding and Yoshikai, 2019).
Metal-organic frameworks (MOFs) are defined as extended porous materials composed of metal ions or metallic clusters and multifunctional organic linkers (Zhang et al., 2009). They are applied in chemical sensors, biomedical imaging, catalysis, etc. (Getman et al., 2012) Zeolite imidazolate frameworks (ZIFs) have emerged as a new subclass of MOFs. Phan et al. (2015) reported efficient heterogeneous catalysis for the synthesis of quinazolines 41 using a cobalt zeolite imidazolate framework (ZIF-67). The reaction of 2-aminobenzophenone 8c with benzyl amine 33 in the presence of the ZIF-67 catalyst in TBHP at 80°C for 180 min delivered the desired quinazolines 41 in 75%–89% yields (Scheme 6A). (Truong et al., 2015) Low temperature, catalyst reusability, and good functional group tolerance are the key features of this methodology.
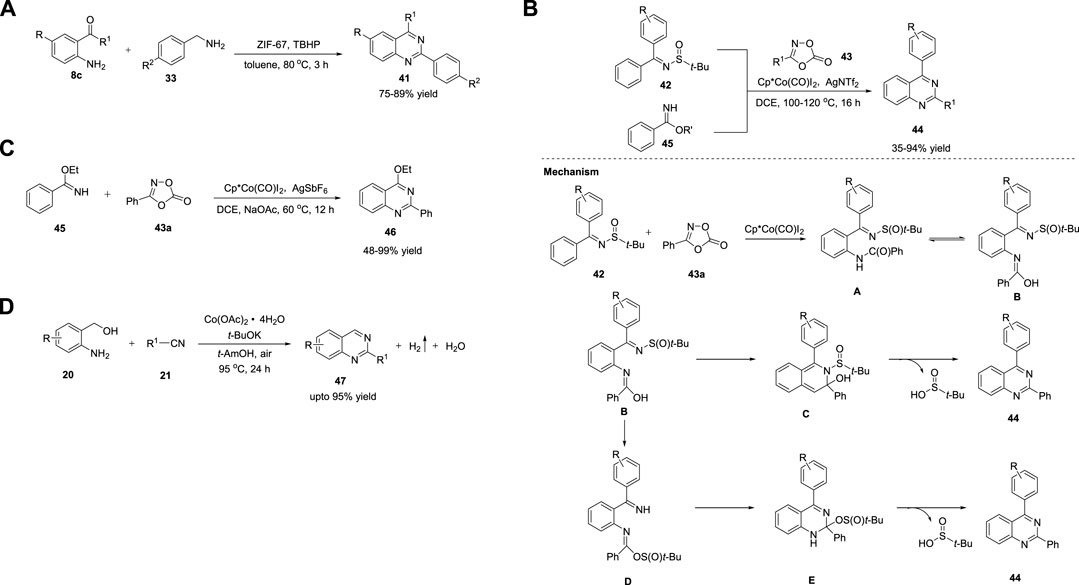
SCHEME 6. (A) Cobalt imidazolate framework ZIF-67 catalyzed synthesis of quinazolines (B) Cp*Co(CO)I2 catalyzed synthesis of quinazolines (C) Synthesis of quinazolines via Co catalyzed [4 + 2] cycloaddition (D) Co-catalyzed ADC coupling for the synthesis of quinazolines.
The C-H functionalization of arenes has been employed as a promising protocol for the synthesis of functional organic molecules and natural products because of the ubiquity of C-H bonds. Direct C-H activation of arenes usually depends on second- and third-row transition metals (McMurray et al., 2011). Li et al. (2016) reported the first synthesis of quinazolines via the C-H activation pathway using a Co catalyst. The optimized conditions involve the reaction of N-sulfinylimines 42 and benzimidates 45 bearing a directing group with dioxazolone 43, a synthon of nitriles, in the presence of Co catalyst Cp*Co(CO)I2 and AgNTf2 (an activator) in DCE at 100°C–120°C for 16 h produced quinazolines 44 in 39%–94% yields (Scheme 6B). (Wang et al., 2016a) Various substituents introduced on the substrates were well tolerated with high regio- and mono/diselectivity. Starting with different arenes, two types of quinazolines 44 were synthesized. In the first approach, C-H activation is aided by an autocleavable N-S bond. In the second approach, the stability of benzimidates 45 is the driving force for the formation of quinazolines 44. The halogen group in quinazoline derivatives 44 should allow further transformations. However, alkyl-substituted dioxazolone 43 reacted with a lower yield.
The mechanism depicted in Scheme 16 for the coupling reaction of N-sulfinylimine 42 and dioxazolone 43a revealed that the initial Co(III)-catalyzed C−H activation followed by amidation generates amide intermediate A, which undergoes 6π-electrocyclization via imidic acid tautomer B to deliver dearomatized intermediate C. Quinazoline 44 is formed via the elimination of tert-butanesulfinic acid. Simultaneously, B undergoes nucleophilic attack at the sulfinyl group to produce an ester intermediate D, which upon intramolecular nucleophilic addition forms E, and subsequent elimination of tert-butanesulfinic acid affords product 44. In both cases, the post-amidation process was uncatalyzed.
In the same year, Glorius et al. (2016) documented Co-catalyzed formal [4 + 2] cycloaddition of arenes with rarely explored free imines and dioxazolones for facile access to quinazolines 46. The reaction of ethyl benzimidate 45 with dioxazolone 43 in the presence of Cp*Co(CO)I2 and AgSbF6 in NaOAc and DCE at 60°C for 12 h produced quinazolines 46 in 48%–99% yields (Scheme 6C) (Wang et al., 2016b). This tandem C-H amidation and cyclization is uniquely catalyzed by the Co complex and represents a complementary process to multi-substituted quinazoline synthesis. The excellent substrate scope and reactivity are attributed to the strong Lewis acidity and high sensitivity to the steric hindrance of the Co(III) catalyst. Easily available substrates and high yields are other advantages of this method.
Recently, Lin et al. (2022) reported an efficient one-pot strategy for Co-catalyzed formation of quinazolines 47 via ADC. The dehydrogenative cyclization of 2-aminoaryl alcohols 20 and nitriles 21 in the presence of Co(OAc)2·4H2O and t-BuOK in tert-AmOH in air at 95°C for 24 h produced quinazolines 47 in up to 95% yields (Scheme 6D). (Hao et al., 2022) The salient features of this protocol are as follows: mild reaction conditions, simple operation, ligand-free, wide substrate compatibility, and cost-effective catalyst.
2.5 Nickel
Ni belongs to the precious metals group, accompanied by Pt and Pd. Ni complexes are highly reactive organometallic species that exhibit multiple oxidation states, are less expensive than other precious metals, and have a strong affinity for unsaturated systems. Numerous Ni-catalyzed reactions have been reported, such as cyclization (Fujimoto et al., 2019), oligomerization (Li et al., 2017), cross-coupling reactions (Muto et al., 2015), and cascade reactions (Chen et al., 2019). Paul et al. (2018) reported an environmentally benign protocol for the production of quinazolines 48 with 25%–85% yields through ADC of a) 2-aminobenzylamine 14a with benzyl alcohol 18 and b) 2-aminobenzylalcohol 20 with benzonitrile 21 in the presence of a Ni catalyst [Ni(MeTAA)] with a macrocyclic ligand (tetramethyltetraaza[14]annulene (MeTAA)) in t-BuOK and xylene at 100°C–140°C for 24 h (Scheme 7A) (Parua et al., 2018).
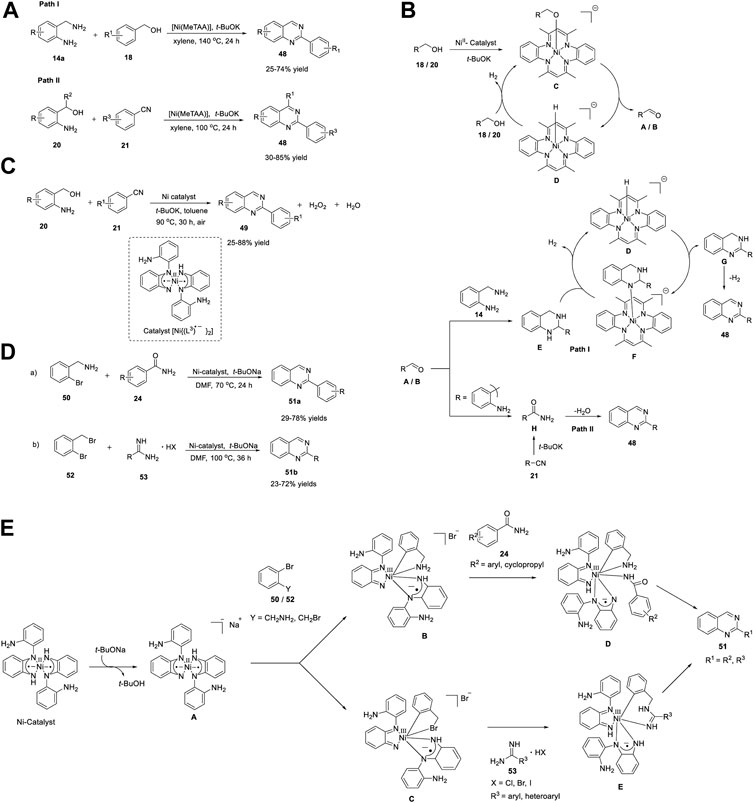
SCHEME 7. (A) Synthesis of quinazolines via Ni-catalyzed acceptorless dehydrogenative coupling (B) Plausible mechanism for the construction of quinazolines through ADC reaction (C) Synthesis of quinazolines via Ni catalyzed dehydrogenative coupling (D) Synthesis of quinazolines via Ni-catalyzed C-N cross coupling (E) Proposed mechanism for Ni-catalyzed synthesis of quinazolines.
The salient features of this strategy include easily available starting precursors, use of an inexpensive, Earth-abundant, easily prepared Ni catalyst, high atom efficiency, non-toxic byproducts such as H2 and water, and broad functional group tolerance in both pathways, including hetero substituents. Notably, addition of styrene as a sacrificial hydrogen acceptor via path I increased the product yield, whereas path II did not require any sacrificial hydrogen acceptor.
A stepwise reaction mechanism has been proposed by the authors (Scheme 7B). The first step is the dehydrogenation of alcohols 18 and 20 to the corresponding aldehydes A and B, in both pathways. This proceeds via the following sub-steps: initial deprotonation of alcohols (benzyl alcohol 18 in path I and 2-aminobenzylalcohol 20 in path II), formation of Ni-alkoxy intermediate C, and finally dehydrogenation. In the second step, benzaldehyde A (Path I) undergoes cyclocondensation with 2-aminobenzylamine 14 to generate intermediate E. In the last step, [Ni(MeTAA)]-catalyzed dehydrogenation of E affords the desired product 48. Likewise, the base-promoted cyclocondensation of 2-aminobenzaldehyde B with benzamide (generated in situ from benzonitrile 21) affords quinazoline 48.
In this endeavor, Paul et al. (2019) developed a new bio-mimetic method for the production of poly-substituted quinazolines 49 through dehydrogenative condensation. Coupling of 2-aminobenzylalcohols 20 with nitriles 21 catalyzed by diradical nickel (II)-complex [NiII{(L3) •−}2] having two diamine ligands in the presence of t-BuOK and toluene at 90°C for 30 h gave the desired quinazolines 49 in 25%–88% yields (Scheme 7C). (Chakraborty et al., 2019) In the Ni catalyst, two one-electron oxidized-tridentate N-(2-aminophenyl)benzene-1,2-diamine ligands (L3) were coordinated with the Ni(II) center in a bidentate manner, where all or a part of the redox process takes place at the ligands.
In the same year, Paul et al. (2019) demonstrated the C-N cross coupling reaction of a) 2-bromobenzylamine 50 with benzamides 24 and b) 2-bromobenzylbromide 52 with amidines 53 to form the corresponding multi-substituted quinazolines 51 in 23%–78% yields, catalyzed by singlet diradical Ni(II)-catalyzed [NiII{(L3) •−}2] in the presence of NaOtBu and DMF at 70°C–100°C for 24–36 h, respectively (Scheme 7D). (Sikari et al., 2019) Under the optimized conditions, different electron-donating and electron-withdrawing groups on benzamide 24 produced significant yields of desired product 51a. The low yields (29%–38% yield) obtained with heteroarylamides 24 might be due to their ability to coordinate with the catalyst, thereby inhibiting the catalytic cycle. Furthermore, electron-donating- and electron-withdrawing-substituted benzamidines 53 were suitable candidates for C-N cross-coupling, providing better yields. However, cyclopropyl amidine 53 afforded quinazoline 51b in a lower yield (23% yield), indicating a limitation in the substrate scope of both approaches. Easy preparation, cost-effectiveness, and air stability are promising features of the Ni catalyst used in this straightforward strategy, which offers an elegant alternative to expensive transition metal catalysts. Furthermore, during catalytic turnover, the cooperative involvement of Ni and ligand-centered redox processes provides an alternative approach for energetically demanding Ni-centered redox processes.
Insight into cross-coupling was obtained by performing mechanistic studies, as shown in Scheme 7E. The reaction mechanism begins with the formation of active catalyst A via deprotonation of the complex. Anionic -NH deprotonated species A facilitates the oxidative addition of aryl halides viz. 2-bromobenzylamine 50 and 2-bromobenzylbromide 52 in a synergistic fashion via Ni(II)/Ni(III) and {(L3)•2−}/{(L3)−} redox couples to form intermediates B and C, respectively. Consequently, N-arylation followed by intramolecular cyclization of intermediates D and E affords quinazolines 51.
2.6 Copper
Copper is an inexpensive, non-toxic, and Earth-abundant metal that catalyzes reactions involving one- and two-electron mechanisms. Cu can activate terminal alkynes. Cu complexes/salts undergo several transformations such as cycloaddition (Brittain et al., 2016), oxidation (Kathiravan et al., 2019), radical (Gu et al., 2020), and coupling reactions (Li et al., 2020).
Fu et al. (2010) reported Ullmann-type coupling for the Cu-catalyzed synthesis of quinazolines 54 in 37%–87% yields from readily available 2-bromophenyl methylamines 50a and amides 24 in the presence of Cu(I) and K2CO3 in i-PrOH in air at 110°C for 24 h (Scheme 8A). (Wang et al., 2010) The ortho-substituent effect of the amino group in 50 was the driving force for the absence of the ligand in this cascade reaction. All substrates were well tolerated. However, aliphatic amides failed to yield any product. Easily available substrates, ligand- or additive-free reaction, and air as green oxidants are some of the key advantages of this protocol. Sequential Ullmann-type coupling begins with the coordination of (2-bromophenyl)methylamine 50a with Cu(I) ions, resulting in intermediate A, which upon oxidative addition gives intermediate B. Intermediate C is formed by the reaction of B with amide 24 in the presence of K2CO3. Subsequently, reductive elimination of C leads to N-arylated species D, leaving the copper catalyst. Intramolecular dehydrative cyclization of D followed by air-promoted aromatization provides quinazoline 54 (Scheme 8A).
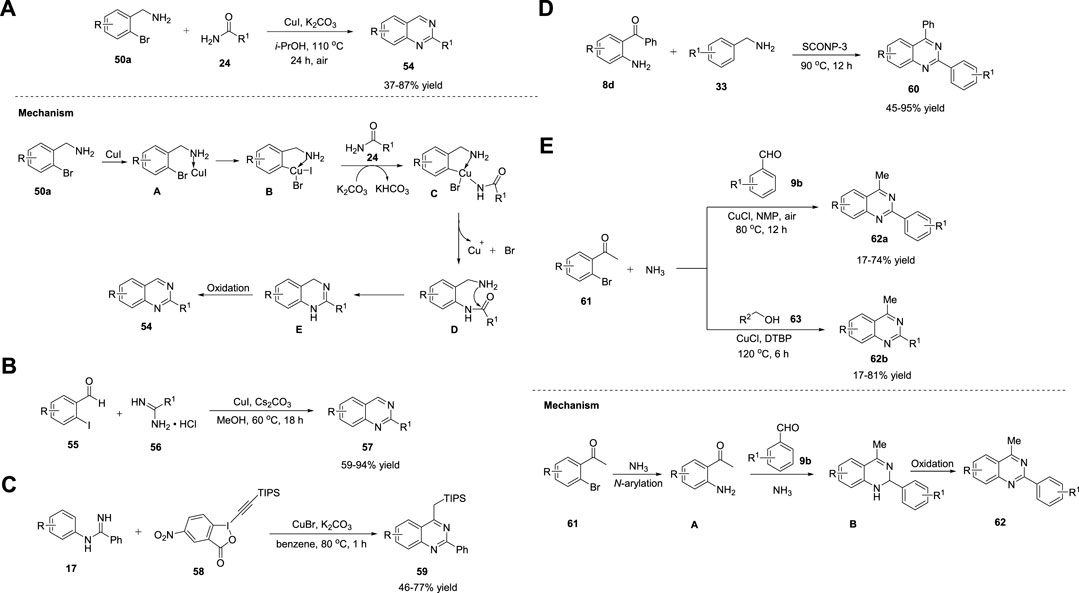
SCHEME 8. (A) Proposed mechanism for Ni-catalyzed synthesis of quinazolines (B) Proposed mechanism for Ni-catalyzed synthesis of quinazolines (C) CuBr catalyzed synthesis of quinazolines via C-H alkynylation (D) CuO NPs catalyzed synthesis of quinazolines (E) CuCl catalyzed synthesis of quinazolines.
Likewise, Truong et al. (2010) proposed a ligand-free Cu-catalyzed Ullmann condensation for highly functionalized quinazolines 57. The reaction of o-iodobenzaldehydes 55 and amidine hydrochlorides 56 in the presence of Cs2CO3 in methanol at 60°C for 18 h afforded the desired product 57 in 59%–94% yields (Scheme 8B), (Truong and Morrow, 2010) while using o-bromobenzaldehyde gave only 5% yield, which is a limitation to the substrate scope. Mild reaction conditions, one-pot methodology, and ligand-free conditions are the striking features of this protocol.
In the same year, Ohno et al. (2010) demonstrated the Cu-catalyzed synthesis of quinazolines 58 from ortho-unfunctionalized aniline derivatives 17 via C-H alkynylation and cyclization. The reaction of N-phenylbenzamidines 17 with 5-nitro-1-[(triisopropylsilyl)ethynyl]-1,2-benziodoxol-3(1H)-one 58 (alkyne source) in the presence of a catalytic amount of CuBr in K2CO3 and benzene at 80°C for 1 h gave quinazolines 59 in 46%–77% yields (Scheme 8C). (Ohta et al., 2010) Furthermore, TIPS could be cleaved by the treatment of 59 with TBAF in THF-AcOH (20:1) at room temperature to yield quinazolines. Direct synthesis and commercially available starting materials are the notable features of this protocol. The formation of highly bipolar byproducts is the reason for the moderate product yields.
Metal NPs have gained attention as semi-heterogeneous catalysts (Shiju and Guliants, 2009). Copper oxide NPs (CuO NPs) belong to this class. Wang et al. (2010) disclosed a novel protocol for the production of a series of quinazolines 60 in 45%–95% yields via the reaction of 2-aminobenzophenones 8d and benzylic amines 33 catalyzed by CuO NPs supported on kaolin (SCONP-3) at 90°C for 12 h (Scheme 8D). (Zhang et al., 2010) Both electron withdrawing and electron donating groups favored the reaction with excellent yields. Inexpensive, stable, and reusable for at least four times are the benefits of this heterogeneous catalyst.
Hua et al. (2012) developed two efficient approaches to produce multi-substituted quinazolines 62a/b: a three-component one-pot reaction of I) o-bromo aromatic ketones/aldehydes 61, aromatic aldehydes 9b, and ammonia water, and II) o-bromo aromatic ketones/aldehydes 61, primary alcohols 63, and ammonia water in the presence of CuCl, NMP, and air/DTBP, respectively, at 80°C–120°C for 6–12 h (Scheme 8E). (Ju et al., 2012) Under optimized conditions, quinazolines 62a/b were obtained in 17%–81% yields using ammonia water as the nitrogen source and air/DTBP as the oxidant. A variety of substituents, including electron-donating and electron-withdrawing groups, were well tolerated. The advantages of this approach include easy availability of precursors and use of air as oxidant. Mechanistically, the Ullmann-type amination reaction of o-haloacetophenones 61 with ammonia generated intermediate A, which upon cyclocondensation with aldehyde 9b and ammonia followed by aerobic oxidation gave desired product 62a/b (Scheme 8E).
Yu et al. (2012) explored aerobic oxidative synthesis using the Cu/N-ligand/TEMPO catalytic system, the first example of its kind, in the synthesis of heterocycles and quinazolines in particular. The reaction of 2-aminobenzylamines 14 with aldehyde 64 in the presence of CuCl/DABCO/4-HO-TEMPO in acetonitrile and oxygen as the terminal oxidant at 80°C for 6 h afforded quinazolines 65 in 40%–98% yields (Scheme 9A). (Han et al., 2012) The aerobic oxidative reaction could be scaled up to one g without any difficulty. Heterocyclic aldehydes 64 such as 3-picolylaldehyde and 2-furylaldehyde also afforded corresponding quinazolines 65 in good yields. However, alkyl aldehydes 64 gave lower yields of the product, which was the only limitation in the substrate scope; otherwise, this is an outstanding work.
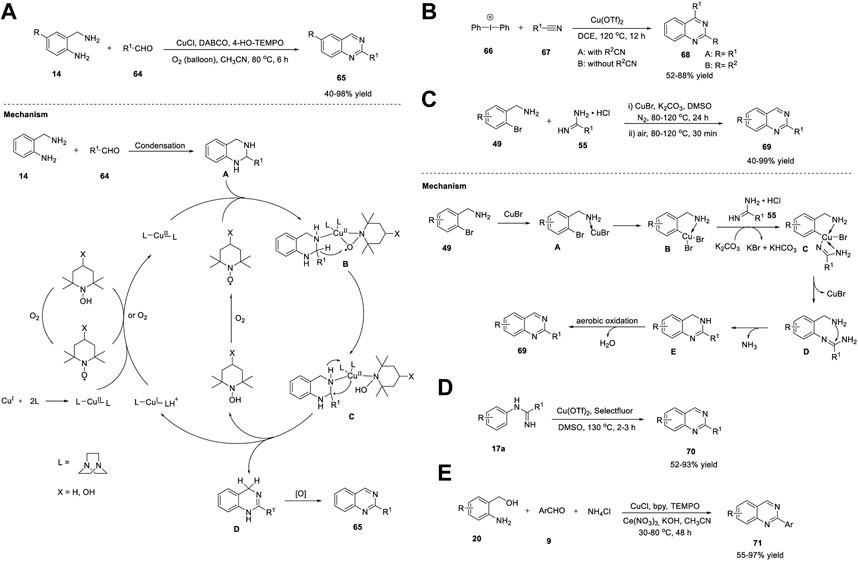
SCHEME 9. (A) CuCl/DABCO/TEMPO- catalyzed synthesis of quinazolines (B) Cu(OTf)2 catalyzed synthesis of quinazolines via [2 + 2+2] annulation (C) CuBr catalyzed synthesis of quinazolines (D) Cu-catalyzed annulation of amidines for the synthesis of quinazolines (E) CuCl catalyzed cascade synthesis of quinazolines.
Oxidative dehydrogenation proceeded via the formation of the CuII-(DABCO)2 complex through the oxidation of CuI-(DABCO)2 by oxygen or TEMPO. Further coordination of the CuII-(DABCO)2 complex with the N-atom of tetrahydroquinazoline A and TEMPO resulted in η-2 manner intermediate B (Caneschi et al., 1988). The transfer of the benzylic hydrogen atom in B to TEMPO via a hydrogen abstraction step resulted in radical-TEMPOH Cu species C. The benzyl radical then underwent oxidation via Cu(II)-mediated inner-sphere electron transfer, resulting in the corresponding carbocation, which then underwent deprotonation to give dihydroquinazoline D, with the simultaneous formation of Cu(I) species and TEMPOH. Subsequently, TEMPOH was autoxidized into TEMPO, which then reoxidized the Cu(I) species into CuII-(DABCO)2. In the last step, intermediate D was oxidized again to form desired product 65 (Scheme 9A).
Chen et al. (2013) presented an efficient approach for the one-pot synthesis of multisubstituted quinazolines 68 via [2 + 2 + 2] cascade annulation with diaryliodonium salts 66 and nitriles 67 in the presence of Cu(OTf)2 in DCE at 120°C for 12 h (Scheme 9B). (Su et al., 2013) Various aliphatic and aromatic nitriles 67 worked well and produced quinazolines 68 in 52%–88% yields. Electrophilic annulation involves the use of readily available starting materials and encompasses a wide range of functional groups. The reaction proceeded with the addition of two different nitriles in sequence to give regioselective products. However, ethyl cyanoformate and diethyl cyanophosphate did not undergo annulation owing to electron deficiency.
Cheng et al. (2013) reported a novel Cu-catalyzed cascade method for the production of quinazolines. The optimized reaction conditions included (2-bromophenyl)methylamines 49 and amidine hydrochlorides 55 as substrates, CuBr as the catalyst, K2CO3 as the base, and DMSO as the solvent at 100°C in N2 for 24 h and then in air for 30 min to furnish quinazolines 69 in 40%–99% yields (Scheme 9C). (Liu et al., 2013) The salient features of this protocol are as follows: inexpensive copper catalyst, air as a green oxidant, and broad functional group tolerance. The proposed mechanism begins with the coordination of (2-bromophenyl)methylamine 49 with CuBr to generate complex A, which upon oxidative addition provides B. Base-mediated complexation of B with amidine gives C. Reductive elimination of C affords N-arylation species D, leaving the catalyst. Subsequently, intramolecular nucleophilic attack of the amino groups on the carbon of the amidine group generates E, resulting in the loss of NH3. Finally, the aerobic oxidation of E results in the desired quinazoline 69 (Scheme 9C).
In this study, Zhang et al. (2013) illustrated a facile Cu-catalyzed synthesis of quinazolines 70 from ortho-unfunctionalized aniline viz. amidines 17a and DMSO, DMA, DMF, TEMDA, or NMP via direct oxidative amination of N-H bonds and C (sp3)-H bonds, followed by intramolecular C-C bond formation reactions. Quinazolines were obtained in 52%–93% yields by employing amidine as a nitrogen source, sp3 carbon in DMSO as a one-carbon synthon, Cu(OTf)2 as the catalyst, and Selectfluor as an oxidant at 130°C for 2–3 h (Scheme 9D). (Lv et al., 2013) This is the first example of a C-N bond formation reaction between the N-H bond of amidine and the methyl C (sp3)-H bonds of solvents. The broad substrate scope and high selectivity of the annulation reaction towards different C(sp3)-H bonds and easy preparation of amidines make this an attractive method. However, cyclic amidines failed to yield the desired product, which is a limitation of this approach.
Wu et al. (2013) reported the first example of the Cu-catalyzed reaction of (2-aminophenyl)methanols 20 with aldehydes 9 in the presence of CuCl, bipyridine, TEMPO, and cerium nitrate hexahydrate–ammonium chloride in KOH and acetonitrile at 30°C–80°C for 48 h, leading to the formation of quinazolines 71 in 55%–97% yields (Scheme 9E). (Chen et al., 2013) The reaction proceeded smoothly and tolerated a wide variety of functional groups. Furthermore, the reaction could be easily scaled up to gram quantities, which is a noteworthy advantage.
Similarly, Fan et al. (2013) developed a simple and economical synthesis method of quinazolines 72 through the Cu-catalyzed cascade reaction of 2-bromobenzylbromides 51, aldehydes 9c, and aqueous ammonia in the presence of Cu(OAc)2, DMAP, and DMSO at 80°C in air for 24 h (Scheme 10A). (Fan et al., 2014) Sequential cupric acetate-catalyzed amination, condensation, intramolecular nucleophilic cyclization, and aromatization led to the roduction of quinazoline 72 in 27%–75% yields. The structural diversity of the products and easy availability of the starting materials are the benefits of this approach.
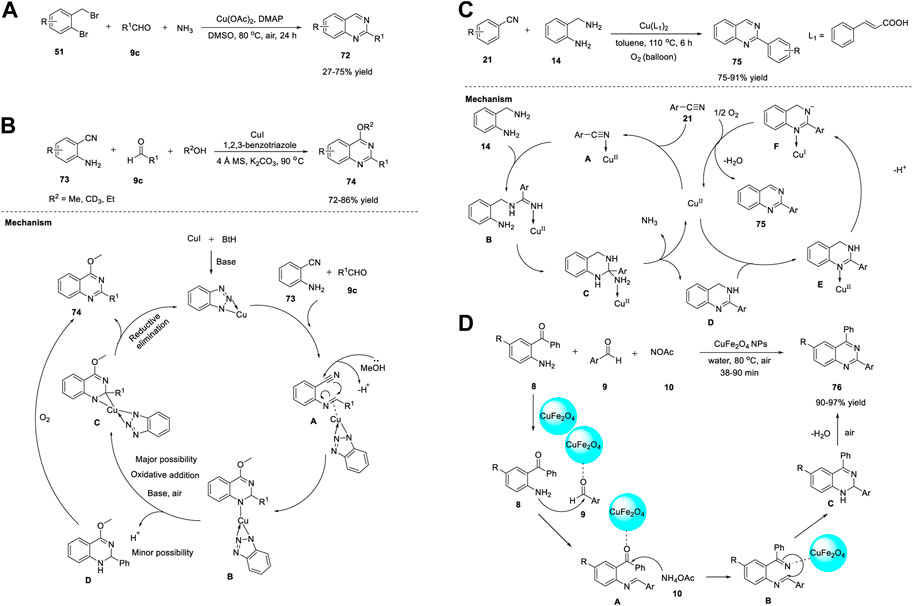
SCHEME 10. (A) Cu(OAc)2 catalyzed synthesis of quinazolines (B) Cu-benzotriazole catalyzed synthesis of quinazolines (C) Cu-catalyzed cascade coupling and aerobic oxidation for the synthesis of quinazolines (D) CuFe2O4 NPs catalyzed synthesis of quinazolines.
In a study, Ahmed et al. (2014) reported an efficient approach to produce o-protected-4-hydroxyquinazolines 74 via copper-benzotriazole (Cu-BtH)-catalyzed intramolecular electrophilic cyclization. The reaction proceeded via the treatment of 2-aminobenzonitriles 73 with aldehydes 9 in the presence of Cu(I)-benzotriazole, K2CO3, and 4 Å molecular sieves in alcohol at 90°C, providing quinazolines 74 in 72%–86% yields (Scheme 10B). (Battula et al., 2014) Use of Cu in catalytic amounts is the benefit of this protocol despite long reaction time and relatively high temperature. In addition, this is the first approach for synthesis of o-protected-4-hydroxyquinazolines 75 through a monocyclic system via a bicyclic intermediate. All types of aldehydes 9c, such as aryl, alkyl, and heteroaryl aldehydes, were converted into the corresponding quinazolines 74 in excellent yields.
A plausible reaction mechanism proposed by the authors is shown in Scheme 10B. Initially, 2-aminobenzonitrile 73 forms an N-arylimine with aldehyde 9c. Complex A is formed by the coordination of the imine with the BtH-ligated Cu. Simultaneous intramolecular electrophilic cyclization and oxidative addition of alcohols gives complex B, which is converted to complex C through oxidative addition in the presence of a base and air. In the last step, the reductive elimination of C leads to the formation of quinazoline 74. An additional pathway is presumed to explain the presence of compound D, wherein the complex in the H+ environment forms dihydroquinazoline, which then undergoes oxidation to afford quinazoline 74.
Li et al. (2014) reported a green method for the synthesis of quinazolines 75 through Cu-catalyzed cascade coupling and aerobic oxidation without the need for additives or bases. The optimized reaction conditions involve the coupling of benzonitrile 21 with 2-aminobenzylamine 14 in the presence of CuII(L1)2 and cinnamic acid as the ligand, under air in toluene at 110°C for 6 h, affording quinazolines 75 in 75%–91% yields (Scheme 10C). (Li et al., 2014) The key features of this strategy include: a) Cu acts as a dual catalyst for both cascade coupling and aerobic oxidation, b) O2 acts as a terminal oxidant, c) base- or additive-free reaction, d) good substrate specificity, and e) mild reaction conditions in a one-pot fashion.
A tentative mechanism as depicted in Scheme 10C begins with the activation of nitrile 21 by Cu(II) to provide an intermediate A. Nucleophilic addition of A with 2-aminobenzylamine 14 gives B. Intermediate C is formed by the intramolecular cycloaddition of B followed by removal of CuII(L1)2 and NH3 furnished the compound D. Then, the nitrogen atom of tertiary amine D coordinates to CuII(L1)2 to form E via a ligand-exchange reaction. Subsequently, intermediate F is formed by the deprotonation of E, with simultaneous liberation of the desired product 75 and reoxidation of the Cu(I) complex to the Cu(II) complex.
Magnetic NPs have gained considerable attention owing to their unique features such as a) ease of preparation from non-toxic substances, b) simple separation by means of an external magnet, c) cost-effective and controllable fabrication, d) low catalyst leaching compared to that of other material-supported catalysts, and e) use of eco-friendly solvents. Baghbanian et al. (2014) reported a one-pot method for the synthesis of quinazoline derivatives 75 in the presence of CuFe2O4 NPs as reusable catalysts in water (Scheme 10D). The tandem cyclization reaction between 2-aminoketone 8 with aldehyde 9 and ammonium acetate 10 in the presence of CuFe2O4 NPs in water at 80°C for 38–90 min produced quinazolines 76 in 90%–97% yields (Scheme 10D). (Baghbanian and Farhang, 2014) The major benefits of this technique are the simplicity of product/substrate extraction from the catalyst, reusability of the catalyst for at least five times, and chemoselectivity. The reaction mechanism is initiated by the coordination of the clean catalyst, CuFe2O4 NPs, with the carbonyl groups of 2-aminoketone 8 and aldehyde 9. This coordination results in increased electrophilicity of the carbonyl carbons and thus promotes the nucleophilic attack of the amine group in 8 and ammonium acetate 10. Subsequently, the condensation of aldehyde 9 with amine 8 generates aldimine A. The attack of ammonium acetate 10 on the keto group of benzophenone leads to the formation of ketimine B. Subsequent ring closure followed by aromatization through dehydration in conjunction with O2 from the air gives desired product 76 (Scheme 10D).
Likewise, Zhang et al. (2014) identified CuO NPs as inexpensive catalysts for producing quinazolines 77 via aerobic oxidative coupling (Scheme 11A). The treatment of N-arylamidines 17b with benzaldehyde 9b or benzyl alcohol 18 in the presence of CuO NPs and 1,10-phenanthroline in toluene in air at 110°C for 24 h led to the formation of quinazolines 77 in 54%–98% yields (Scheme 11A). (Zhang et al., 2014b) CuO NPs have proven to be more effective heterogeneous catalysts than the other CuO nanocatalysts and commercial CuO, exhibiting remarkable efficiency. The straightforward synthetic protocol, inexpensiveness, recyclability of the Cu catalyst, high generality, and good functional group tolerance are the benefits of this oxidative coupling approach.
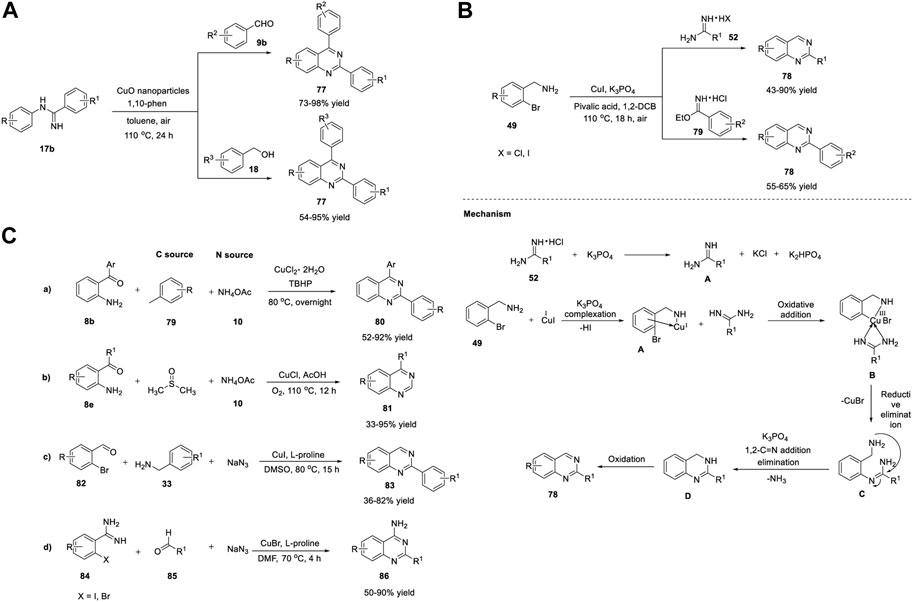
SCHEME 11. (A) Synthesis of quinazolines via CuO NPs catalyzed oxidative coupling (B) Cu(I)-catalyzed N-arylation for the synthesis of quinazolines (C) Cu-catalyzed amination/one-pot method for the synthesis of quinazolines.
Beifuss et al. (2014) demonstrated two efficient methods for the synthesis of 2-substituted quinazolines 78 via Cu-catalyzed domino intermolecular N-arylation/intramolecular nucleophilic substitution (Scheme 11B).
The first method involved one-pot reaction between 1-(2-bromophenyl)methanamines 49 and amidines 52/imidates 79 using Cu(I) as catalyst, K3PO4 as base, and pivalic acid as an additive in 1,2-dichlorobenzene at 110°C for 18 h under aerial O2 as an oxidant to produce quinazolines 78 in 43%–90% yields (Scheme 11B). (Omar et al., 2014) External oxidant-free, ligand-free, broad substrate scope of both 1-(2-bromophenyl)methanamines 49 and amidines 52, and inexpensive Cu catalyst are the promising features of this protocol. Mechanistically, coordination of Cu(I) with 1-(2-bromophenyl)methanamine 49 in the presence of a base gives intermediate A. Oxidative addition of A, followed by complex formation with the amidine, generates B, which then undergoes reductive elimination to produce C and CuBr. Furthermore, intramolecular 1,2-addition of an amine group to the CN group of the amidine species in the presence of a base delivers the cyclized species D, with loss of ammonia. Finally, Cu-catalyzed oxidation of D with O2 in air results in quinazoline 78 (Scheme 11B).
Wang et al. (2015) reported a novel Cu-catalyzed double oxidative C-H amination of methylarenes 79 for the formation of 2-arylquinazolines 80. The optimized reaction conditions involved the treatment of 2-aminobenzoketones 8b with ammonium acetate 10 (N source) and toluene 79 as the solvent and a reagent in the presence of CuCl2·2H2O as the catalyst and TBHP as an oxidant at 80°C to generate quinazolines 80 in 52%–92% yields (Scheme 11Ca) (Liu et al., 2015).
In this reaction, a variety of electron-donating and electron-withdrawing groups are well tolerated, and, surprisingly, aliphatic substituents of 2-aminobenzoketones also give products in excellent yields. Cu acts as a dual catalyst in the construction of one C=N bond and one C-N bond in a single step. A plausible mechanism proposed by the authors suggests that the coordination of 2-aminobenzoketone 8c with the Cu(II) species produces complex A via a ligand exchange reaction. Then, benzyl/Cu(II) species B is formed via benzylic C-H activation of complex A. Complex B then undergoes oxidation with another Cu(II) to give benzyl/Cu(III) complex C and one Cu(I) species. Next, reductive elimination of complex C generates intermediate D and another Cu(I) species. Simultaneously, D is converted into F via β-H elimination. In the last step, intermediates F and NH3 are converted to G via a similar catalytic cycle, which then forms 80 through condensation and oxidation. The Cu(I) species are then oxidized to the corresponding Cu(II) species in the presence of TBHP (Scheme 12A).
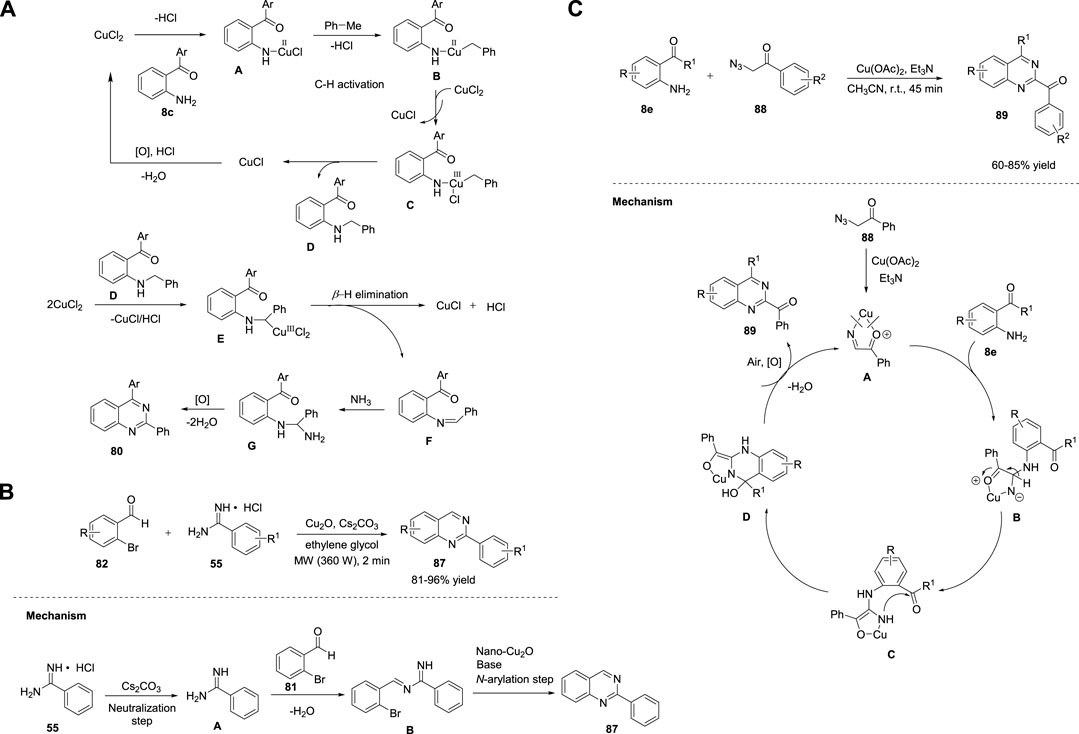
SCHEME 12. (A) Cu-catalyzed dual oxidative benzylic C-H amination for the synthesis of quinazolines (B) Cu2O nanocubes-catalyzed synthesis of quinazolines (C) Synthesis of quinazolines via Cu-catalyzed transimination.
Ma et al. (2016) reported a novel three-component reaction for the synthesis of quinazolines 81 via Cu-catalyzed oxidative amination. Quinazolines were obtained in 33%–95% yields by employing N-alkylamide viz. 2-aminobenzophenone 8e and DMSO as the sp3 carbon source, ammonium acetate 10 as the nitrogen source, O2 as the green oxidant, and CuCl as the catalyst in acetic acid at 110°C in air for 12 h (Scheme 11Cb) (Duan et al., 2016). Moreover, aromatic aldehydes and primary alcohols served as carbon sources to afford the corresponding 2-aryl and 2-alkyl quinazolines 81. The approach is simple, atom-economical, and external oxidant-free, and DMSO acts as both a solvent and reagent.
Similarly, Wu et al. (2016) reported an efficient three-component reaction of 2-bromobenzaldehydes 82, benzyl amines 33, and sodium azide (N source) for assembling quinazolines 83 in 36%–82% yields via the Cu(I)/L-proline catalytic system in DMSO at 80°C for 15 h (Scheme 11Cc) (Xu et al., 2016). Except for the steric effect of 2-bromobenzaldehydes 82 on electron-deficient substrates, the remaining heteroaryl and electron-rich benzylamines 33 produced quinazolines 83 in good yields. In situ-generated aryl azides, readily available substrates, and dual activity of Cu(I) catalysts to promote SNAr and denitrogenation/cyclization are remarkable features of this approach.
Adopting the same strategy, Chen et al. (2017) developed a Cu-catalyzed one-pot methodology for the synthesis of 4-aminoquinazolines 86. The CuBr/L-proline-catalyzed reaction proceeded by the treatment of 2-iodo/2-bromobenzimidamides 84, aldehydes 85, and sodium azide in DMF at 70°C for 4 h to afford quinazolines 86 in 50%–90% yields (Scheme 11Cd) (Yang et al., 2017). The reaction mechanism involved consecutive SNAr substitution, reduction, cyclization, oxidation, and tautomerization. Simple operation conditions, low temperatures, base-free reaction, and easy availability of starting precursors are the notable advantages of this approach.
In recent years, sonochemical techniques have received considerable attention for the preparation of inorganic nanomaterials such as monometallic, bimetallic, carbide, and oxide (Li et al., 2021). As an alternative to conventional methods, ultrasonication is considered favorable, cost-effective, powerful, eco-friendly, and employs easy reaction parameters that will increase the selectivity and yield of the product. Bhanage et al. (2017) reported the facile ultrasonic-assisted green synthesis of Cu2O-nanocubes. Furthermore, Cu2O nanocubes were used as heterogeneous nanocatalysts in the microwave-assisted preparation of quinazolines 87 at 360 W for 2 min via tandem cyclization of 2-bromobenzaldehydes 82 with amidines 55 in the presence of Cs2CO3 in ethylene glycol (Scheme 12B) (Raut et al., 2017).
A variety of electron-donating and electron-withdrawing substrates underwent the reaction smoothly and produced quinazolines 87 in 81%–96% yields. Ligand-free reaction, shorter reaction times, and recyclability of the catalyst at least four times are the merits of this green approach. The proposed mechanism begins with the neutralization of benzamidine hydrochloric salt 55 to give the amidine species A, which then react with 2-bromobenzaldehyde 82 to form imine intermediate B. Imine B undergoes Ullmann type N-arylation, generating the desired product, quinazoline 87 (Scheme 12B).
Kamal et al. (2017) developed an operationally simple approach for quick production of quinazoline derivatives via a Cu-catalyzed base-mediated system. The method involved the transimination of o-aminoketones 8 with in situ-generated imine, obtained from the decomposition of phenacyl azides 88, in the presence of Cu(OAc)2 in Et3N as the base and acetonitrile as the solvent, at room temperature for 45 min, enabling the formation of substituted quinazolines 89 in 60%–85% yields (Scheme 12C). (Sastry et al., 2017) The reaction proceeded smoothly with a wide range of substituents on the aromatic ring. However, alkyl acyl azide instead of 88 failed to form the desired product. Mechanistically, the Cu(OAc)2/Et3N catalytic system initiates the loss of nitrogen from phenacyl azide 88 and generates Cu-chelated imine A. Transimination between o-carbonyl aniline 8e and imine results in intermediate B. Deprotonation of B provided intermediate C. Nucleophilic attack of the amine group of intermediate C on the carbonyl group gives D. Finally D, undergoes aromatization in the presence of atmospheric oxygen to afford quinazoline 89 (Scheme 12C).
Jiang et al. (2018) demonstrated the concise construction of substituted quinazolines 91–92 via Cu(OAc)2-catalyzed cyclization of 2-ethynylanilines 90 with benzonitriles 21 in the presence of t-BuOK as a base, toluene/DMSO, and molecular O2 at 100°C–120°C for 12–24 h (Scheme 13A). (Wang et al., 2018) This strategy enabled the effective assembly of C-N and C-C bonds and aerobic C-C triple bond cleavage. The major advantages of this strategy are readily available starting materials and catalysts, broad functional group tolerance with 41%–88% yields, and no external oxidant. However, heterocyclic substituted alkynes fail to afford the desired product. Furthermore, a new solid-state blue-emitting organic molecule was identified during the procedure, which has drawn attention for further development of a new variety of aggregation-induced emission (AIE) luminescent materials.
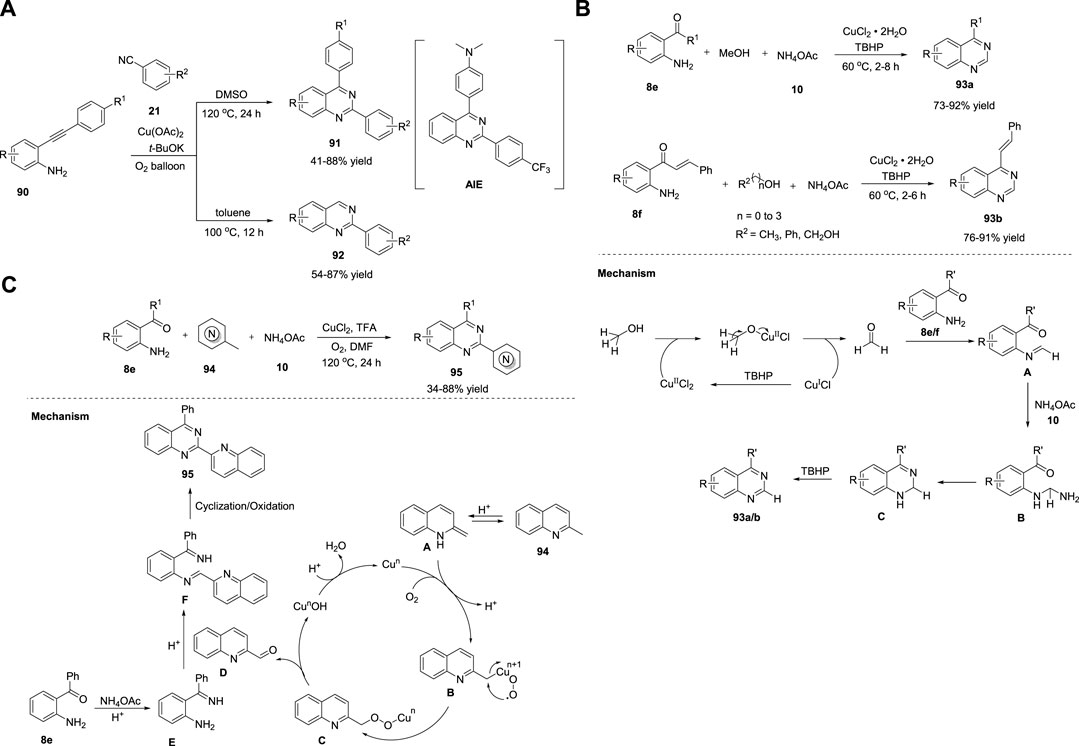
SCHEME 13. (A) Cu(OAc)2 catalyzed C-C bond cleavage for the synthesis of quinazolines (B) Cu-catalyzed oxidative amination of methanol for the synthesis of quinazolines (C) Cu-catalyzed aerobic oxidative cyclization for the synthesis of quinazolines.
Ilangovan et al. (2019) illustrated a straightforward method for the assembly of quinazolines 93 using naturally abundant methanol as the C1 carbon source. The one-pot reaction of 2-aminoarylketones 8e or 2-amino chalcone 8f, alcohols, and ammonium acetate 10 in the presence of CuCl2·2H2O in TBHP at 60°C for 2–8 h led to the formation of quinazolines and styryl quinazolines 93a/b in 73%–92% yields (Scheme 13B). (Satish et al., 2019) Atom-conomy, easy scale-up to gram quantities, methanol as a carbon source, and high yields are the benefits of this protocol. The plausible mechanism depicted in Scheme 13B begins with the dehydrogenation of methanol to produce formaldehyde in the presence of CuCl2·2H2O/TBHP. Subsequently, the in situ-generated formaldehyde is condensed with the aromatic primary amine of 8e/f to produce imine species A. Intermediate A reacts with ammonium acetate 10 to produce aminal B, which then undergoes intramolecular cyclization and oxidation to produce the desired quinazoline 93a/b.
As an improvement, Tang et al. (2019) reported a one-pot method to produce 2-azaaryl quinazolines 95 from 2-aminophenyl ketones 8e, methylazaarenes 94, and ammonium acetate 10 using CuCl2 as the catalyst and trifluoroacetic acid in DMF at 120°C for 24 h (Scheme 13C). (Liang et al., 2019) This aerobic oxidative cyclization provided several substituted quinazolines in 34%–88% yields. The features of this method include easily available starting precursors, mild reaction conditions, green oxidants, and good substrate applicability. The tentative mechanism proposed by the authors suggests the isomerization of 94 with acid-generated enamine intermediate A. Next, A is combined with Cu salts and O2 to give intermediate B, which then undergoes intramolecular rearrangement to form intermediate C, leaving behind quinoline-2-carbaldehyde D. Intermediate E formed from 8e reacts with quinoline-2-carbaldehyde D to produce imine intermediate F through acid-catalyzed dehydration. In the last step, imine intermediate F undergoes cyclization and oxidation to afford desired product 95 (Scheme 13C).
Xie et al. (2020) reported an unprecedented approach for the synthesis of quinazoline derivatives 98 by employing Cu/Ag-catalyzed annulation of anthranils 96 with azirines 97. This novel strategy involving the cleavage of the C-N bonds of 2H-azirines attacked by anthranils as nucleophiles has not been explored before. The fact that 2H-azirines can act as nucleophiles to cleave the N-O bond of anthranils may be the reason. The optimized conditions included the reaction of anthranils 96 with phenyl-2H-azirine 97 in the presence of Cu(OAc)2 as the catalyst, AgSbF6 as the additive, acetic acid in DCE, and O2 at 100°C for 12 h to produce (quinazolinyl-2-yl)methanones 98 in 25%–72% yields via an unexplored 1,3-hydroxyl migration and β-N elimination (Scheme 14A) (Sun et al., 2020).
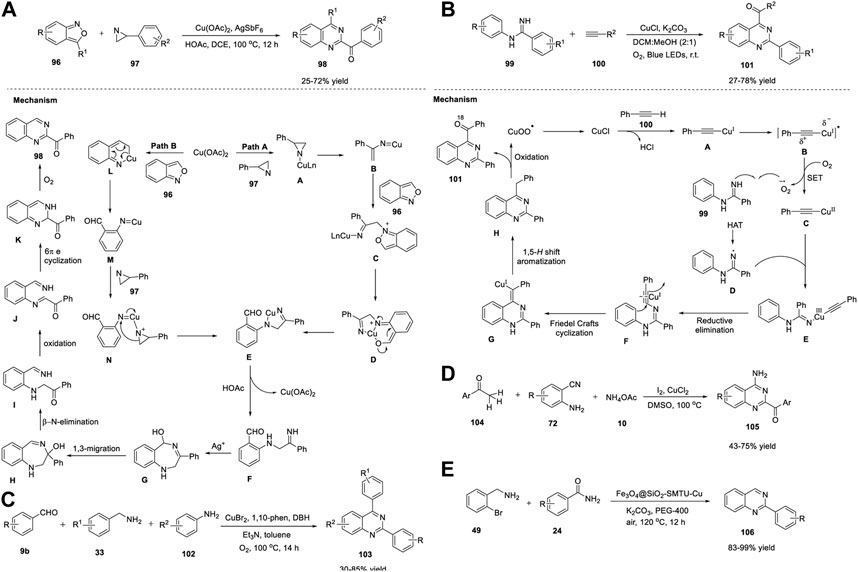
SCHEME 14. (A) Cu/Ag-catalyzed synthesis of quinazolines (B) Cu-catalyzed photoredox synthesis of quinazolines (C) Cu-catalyzed [3 + 2 + 1] annulation for the synthesis of quinazolines (D) I2/CuCl2-copromted [4 + 1 + 1] cyclization for the synthesis of quinazolines (E) Fe3O4@SiO2-SMTU-Cu nanocomposite catalyzed synthesis of quinazolines.
Steric hindrance did not influence the reaction by accommodating a variety of electronically and sterically variable functional groups, and the product was produced in good yields. However, 3-(thiophene-2-yl)-2H-azirine 97 did not afford the desired product, which is a minor limitation in the substrate scope. Atom-economy, easy scale-up with 70% yield, cheap and abundant catalysts, and good substrate compatibility are the benefits of this protocol. Mechanistically, azirine 97 coordinates with the Cu catalyst to generate complex A. Azirine complex A then transforms into the corresponding nitrene–Cu complex B via reversible N-C (Jafari et al., 2016) bond cleavage. Anthranil 96 attacks complex B to give intermediate C. Cu is then inserted into the adjacent N-O bond of C to produce intermediate D, which then undergoes isomerization to form complex E (path A). Alternatively, a Cu salt can be inserted into the cleavable N−O bond of anthranil 96, resulting in Cu–nitrenoid species M, which further coordinates with 2H-azirine 97 to afford intermediate N. Subsequently, migratory insertion of nitrenoid species into the N-C (Jafari et al., 2016) bond in N provides complex E (path B). E is protonated by HOAc and generates intermediate F, leaving behind Cu(II) acetate by closing the catalytic cycle. AgSbF6 promotes the cyclization of F to deliver intermediate G. Subsequently, 1,3 migration of the hydroxy group and β-N elimination gives α-aminocarbonyl intermediate I, which then undergoes coordination with Cu(II) and oxidizes to form intermediate J. Intermediate J then transforms into 1,2-dihydroquinazoline product K via 6π electrocyclization. In the last step, autoxidation of K affords quinazoline 98 (Scheme 14A).
Traditional photocatalytic reactions mostly demand expensive transition metals, specially designed ligands, organic oxidants, and reaction waste. Visible-light photoredox catalysis (PRC) has emerged as a complementary approach to traditional photocatalysis. Hwang et al. (2021) achieved visible-light-induced photoredox Cu-catalyzed oxidative Csp (Jafari et al., 2016)-H annulation (Friedel–Crafts-type cyclization) of amidines 99 with terminal alkynes 100 to assemble functionalized quinazolines 101. The oxidative Csp (Jafari et al., 2016)-H annulation of N-phenylbenzimidamide 99 with phenylacetylene 100 catalyzed by CuCl, by employing K2CO3 as a base, in DCM:MeOH (2:1) in presence of O2 at room temperature for 22–24 h produced quinazolines 101 in 27%–78% yields (Scheme 14B). (Charpe et al., 2021) The promising features of this approach include: a) the construction of 2,4-disubstituted quinazolines at RT through simultaneous C-C and C-N bond formation; b) use of cost-effective CuCl catalyst and O2 as an oxidant under mild reaction conditions; c) ligand-free; d) ease of scale-up; e) water as the only by-product; f) broad substrate scope; and g) green metrics and Eco-Scale evaluations proved that this photochemical process is simple and environmentally benign. The annulation mechanism begins with the absorption of blue light by the in situ-generated Cu(I)-phenylacetylide A (λmax = 472 nm) with visible-light irradiation to form the photochemically excited triplet state B (long-lived triplet lifetime, τ = 15.95 μs). B then donates an electron to molecular O2 and generates Cu(II) complex C and superoxide anion radical via the SET process. Subsequently, owing to the basic nature of the copper superoxo radical anion, it abstracts acidic NH protons to generate nitrogen-centered radical D, which then reacts with Cu(II)–phenylacetylide complex C and delivers Cu(III)-complex species E. Upon reductive elimination, intermediate E gives the Cu(I)-coordinated ynamine intermediate F. Furthermore, intermediate F undergoes Friedel–Crafts-type cyclization (6-exo-dig cyclization) to form cyclized intermediate G and subsequent aromatization to form compound H, which then undergoes photo-oxidation by Cu(II) superoxo (λabs = 486 nm) to yield the desired product 101 (Scheme 14B).
Recently, Liu et al. (2021) reported a Cu-catalyzed multicomponent [3 + 2+1] annulation reaction of benzaldehyde 9b, benzylamine 33, and aniline 102 for the facile access to quinazolines 103. The cascade reaction proceeded with the treatment of benzaldehyde 9, benzylamine 33, and aniline 102 in the presence of CuBr2 and 1,10-phenanthroline in dibromohydantoin (DBH) with triethylamine and toluene in O2 at 100°C for 14 h to produce quinazolines 103 in 30%–85% yields (Scheme 14C). (Wang et al., 2021) The broad substrate scope, ease of scale-up to 1.72 g with 68% yield, and oxygen as a green oxidant are the advantages of this protocol. This is the first analogous three-component synthesis that employs benzaldehyde, benzylamine, and aniline for the assembly of quinazoline derivatives.
In the same year, Wu et al. (2021) demonstrated that I2/CuCl2-copromoted diamination of C(sp3)-H bonds for the facile synthesis of quinazolines 105. The [4 + 1+1] annulation reaction between methyl ketones 104, 2-aminobenzonitriles 72, and ammonium acetate 10 in the presence of I2/CuCl2 in DMSO at 100°C resulted in the formation of 2-acyl-4-aminoquinazolines 105 in good yield (Scheme 14D). (Huang et al., 2021) This became the first example of the assembly of 2-acyl-4-aminoquinazolines using a methyl group as an input. Multiple C-N bond formation, dual C(sp3)-H amination, broad functional group compatibility, and multicomponent cyclization are the features of this approach.
Recently, Sajjadi et al. (2022) reported that the Fe3O4@SiO2–SMTU–Cu nanocomposite catalyzed the synthesis of quinazolines 106 from 2-bromobenzylamine 49 and benzamides 24 in K2CO3 and PEG-400 in air at 120°C for 12 h (Scheme 14E). (Riadi et al., 2022) The reaction proceeded smoothly with a variety of functional groups and delivered product 106 in 83%–99% yields. This is the first report of the synthesis of quinazolines using a magnetic copper nanocatalyst. The catalyst was fabricated through immobilization of Cu(NO3)2 on the surface of silica-coated magnetic Fe3O4 NPs (Fe3O4@SiO2) functionalized with the S-benzylisothiourea ligand. The notable merits of this strategy include excellent yields, easy catalyst separation using an external magnet, shorter reaction times, non-toxic Cu catalyst, and simple operation procedure.
3 Second-row transition metal catalysts
3.1 Ruthenium
Ruthenium, a rare transition metal, has multiple oxidation states. As compared to other precious transition metals, it is less expensive and acts as an important catalyst for synthetic transformations, such as hydrogenation reactions (Sayed et al., 2020), oxidative transformations (Dutta et al., 2019), and coupling reactions (Duarah et al., 2018).
Zhang et al. (2014) reported a Ru3(CO)12/Xantphos/t-BuOK catalyst system for the ADC reaction that yields 2-arylquinazolines 107. Treatment of 2-aminoaryl methanols 20 with benzonitriles 21 in the presence of Ru3(CO)12 as the catalyst, Xantphos as the ligand, and t-BuOK as the base in t-AmOH in N2 at 130°C for 16 h produced 2-arylquinazolines 107 in 43%–76% yields (Scheme 15A) (Chen et al., 2014a). Moreover, aliphatic nitriles 21 afforded the expected product in a lower yield (18% yield). Advantages of this protocol are its simple operation, broad substrate scope, high atom efficiency, and commercially available catalyst system. The ADC proceeds in the following sequence: 1) nucleophilic addition of the amino group of 20 to benzonitrile 21 generates an amidine intermediate A. 2) Next, the ruthenium-catalyzed dehydrogenation of the alcohol group of A yields o-carbonyl amidine B. 3) In the last step, intramolecular condensation of B produces quinazoline 107 (Scheme 15A).
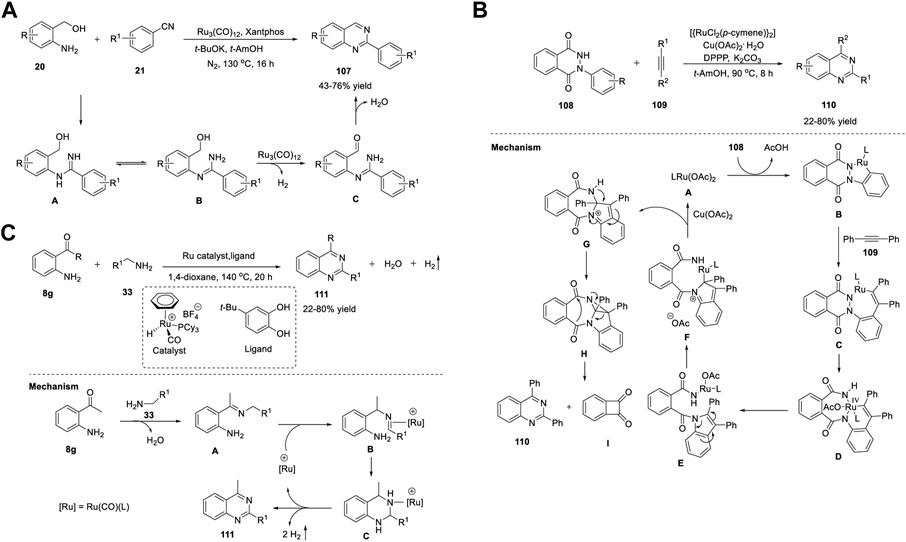
SCHEME 15. (A) Ru3(CO)12 catalyzed ADC coupling for the synthesis of quinazolines (B) Ru-catalyzed C-H activation and annulation for the synthesis of quinazolines (C) Ligand-promoted Ru-catalyzed synthesis of quinazolines.
Gogoi et al. (2018) developed an unprecedented Ru(II)-catalyzed C-H activation and annulation approach for the facile access to quinazolines 110 via C-C triple bond cleavage. The reaction of 2-phenyldihydrophthalazinedione 108 with alkyne 109 using [{RuCl2(p-cymene)}2] as the catalyst and Cu(OAc)2·H2O as the additive, 1,3-bis-(diphenylphosphino)propane (DPPP) as the ligand, and K2CO3 as the base in t-AmOH at 90°C for 8 h delivered quinazolines 110 in 22%–80% yields (Scheme 15B). (Prakash et al., 2018) In this cleavage reaction, both fragments of the alkyne remain in the product. Both the partners viz. N-arylpyrazol-5-ones and diaryl-/arylalkyl-substituted alkynes are well tolerated with good yields. However, dialkyl-, terminal-, silyl-, and bromo-substituted alkynes did not favor the reaction. The reaction mechanism is initiated by the active Ru(II) catalyst A generated from [RuCl2(p-cymene)2] and Cu(OAc)2. The active catalyst irreversibly reacts with 108 to produce complex B through the activation of the C-H bond. The next step is the insertion of alkyne 109 into the C-Ru bond to deliver a seven-membered Ru(II) complex C. Oxidation of C leads to the formation of Ru(IV) complex D via cleavage of the N-N bond. Subsequent reductive elimination–activation at C-2 reductive elimination–intramolecular cyclization–intramolecular rearrangement and elimination generates the indole derivative E, Ru complex F, seven-membered amide G, diazacyclopropane azulene H, quinazoline derivative 110, and diketo compound I in a stepwise manner (Scheme 15B).
Yi et al. (2019) reported a novel ligand-promoted Ru-catalyzed dehydrogenative coupling reaction for the facile access to quinazolines 111. The coupling reaction between 2-aminophenyl ketones 8 and amines 33 in the presence of an in situ-generated catalytic system, Ru–hydride complex [(C6H6)(PCy3)(CO)RuH]+ BF4−, and 4-(1,1-dimethylethyl)-1,2- benzenediol (L1) as the ligand in 1,4-dioxane at 140°C for 20 h generated quinazoline derivatives 109 in 45%–87% yields (Scheme 15C). (Arachchige and Yi, 2019) The prominent advantages of this method are as follows: a) readily available substrates, b) wide substrate scope accommodating common functional groups with good yields, c) no need for any reactive reagents, and d) water and hydrogen are the only byproducts of the reaction. A plausible mechanism proposed by the authors suggests that the initial dehydrative coupling of amino ketone 8g and amine 33 generates imine intermediate A. Then, the Ru catalyst facilitates imine A isomerization to give imine-coordinated species B, which upon cyclization and dehydrogenation furnishes the desired product 111. The authors believe that the redox-active catechol ligand may facilitate the dehydrogenation step in catalysis (Scheme 15C).
Hao et al. (2019) first reported the synthesis of quinazolines 112 catalyzed by the NNN pincer Ru(II) complex via the ADC reaction of o-aminobenzyl alcohols 20 and (hetero)aryl or alkyl nitriles 21 in the presence of t-BuOK as the optimal base in tert-AmOH in air at 130°C for 2 h (Scheme 16A) (Wan et al., 2019). This strategy has gained attention because it overcomes the limitations of previously reported Ru-catalyzed syntheses and exhibits advantages such as shorter reaction time (2 h) compared with previous synthesis (16 h), compatibility with aliphatic nitriles, affording up to 87% yield (18%), and environmental benignity.
Mechanistically, in the presence of t-BuOK, o-aminobenzyl alcohol 20 reacts with the Ru catalyst to generate Ru-alkoxide A, which then undergoes β-elimination to produce Ru-H species B and carbonyl intermediate C. The reaction of Ru-H species B with 20 regenerates Ru-alkoxide intermediate A by evolving hydrogen gas. Simultaneously, the base promotes the hydration of benzonitrile 21, followed by cyclocondensation to afford the desired quinazoline 112 (Scheme 16A).
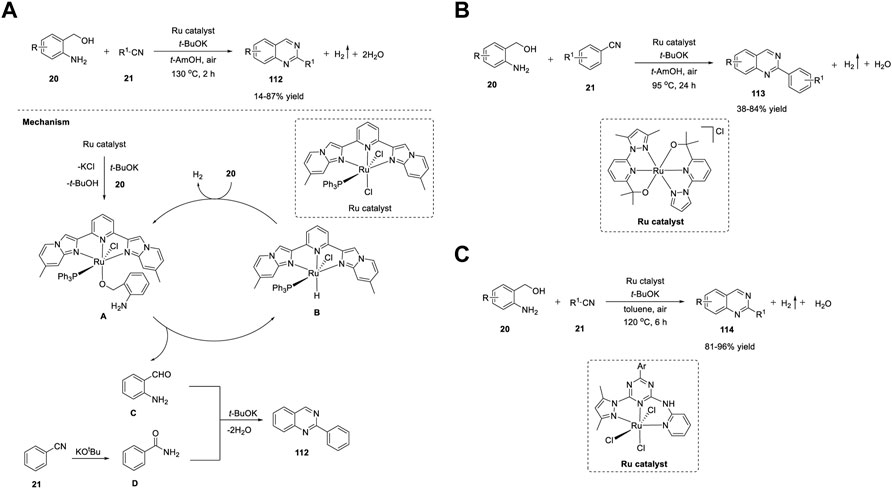
SCHEME 16. (A) Ru(II) NNN pincer catalyzed synthesis of quinazolines (B) Ru(III)-catalyzed ADC synthesis of quinazolines (C) Ru NNN pincer catalyzed acceptorless dehydrogenative synthesis of quinazolines.
Hao et al. (2021) developed an N,N,O-tridentate pyrazolyl-pyridinyl-alcohol ligand-supported Ru(III) complex for the facile synthesis of quinazolines 113. The dehydrogenative coupling of 2-aminoaryl methanols 20 with nitriles 21 in the presence of Ru(III) complex L2RuCl and t-BuOK in tert-AmOH at 95°C for 24 h delivered product 113 in 38%–84% yields (Scheme 16B). (Huo et al., 2021) The easy preparation, air/moisture stable catalyst, atom economy, phosphine ligand-free, sustainable strategy, and broad functional group tolerance are noteworthy merits of this approach.
Recently, Das et al. (2022) demonstrated an efficient method for the Ru NNN-pincer complex-catalyzed synthesis of quinazoline derivatives 114 via ADC of 2-amino benzyl alcohols 20 and nitriles 21 in the presence of t-BuOK in toluene at 120°C for 6 h. The products were obtained in 81%–96% yields (Scheme 16C). (Bhattacharyya et al., 2022) Remarkably, this catalytic system displayed a promising high TON of 290000 for 2-phenylquinazoline 114, which is the highest value reported so far for transition metal-based catalysts.
3.2 Rhodium
Rhodium is one of the rarest and most expensive metals. This noble metal favors a wide range of chemical transformations such as hydrogenation (Schiwek et al., 2020), hydroformylation (Alsalahi and Trzeciak, 2021), and C-H activation (Zhou et al., 2016), in the form of Rh(II) and Rh(III) complexes.
Transition-metal-catalyzed C-H functionalization has emerged as a shortened synthetic sequence in recent years for the construction of C-C/C-hetero bonds (Ye and Cramer, 2015). However, the presence of a nearby directing group is generally needed to direct the position of a metal catalyst for specific C-H bond activation. Zhu et al. (2016) reported a rarely explored double C−N bond formation strategy to construct highly substituted quinazolines 115 using benzimidates 45 and dioxazolones 43 in the presence of a redox-neutral [Cp*RhCl2]2/AgBF4 catalytic system with DCE as a solvent at 50°C for 5 h (Scheme 17A). (Wang et al., 2016c) A wide range of electron-donating and electron-withdrawing substrates perform well to afford the corresponding products in 66%–96% yields. Ideally, dioxazolone acts as an internal oxidant to maintain the catalytic cycle and a coupling partner to yield quinazolines 115. The mechanism is similar to that presented for Co-catalyzed synthesis proposed by Li et al. (2016).
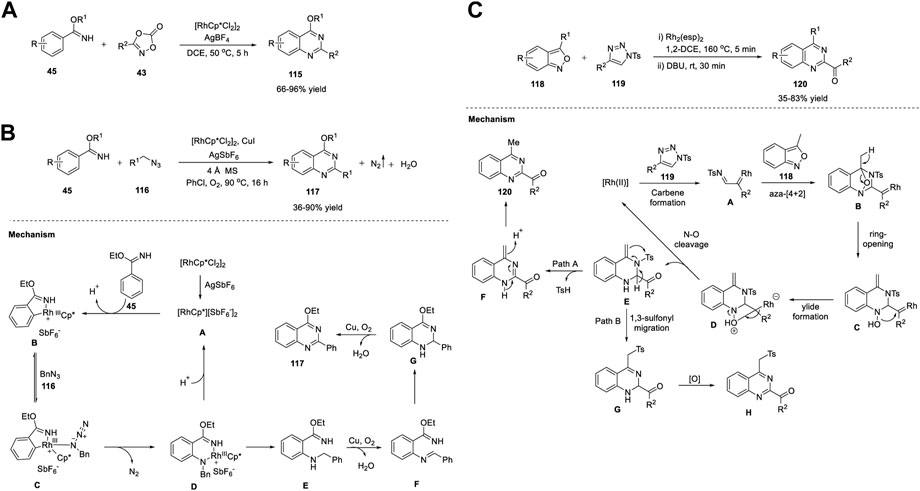
SCHEME 17. (A) Rh(III)-catalyzed synthesis of quinazolines (B) Rh- and Cu-co-catalyzed C-H activation for the synthesis of quinazolines (C) Rh(II)- catalyzed transannulation for the synthesis of quinazolines.
Jiao et al. (2016) presented a novel Rh- and Cu-co-catalyzed C-H bond activation and annulation approach for the facile access to quinazoline derivatives 117. The aerobic oxidative [4 + 2] C-H annulation of imidate esters 45 and alkyl azides 116 in the presence of [Cp*RhCl2]2 as the catalyst, Cu(I) as the co-catalyst, AgSbF6 as the additive in 4 Å MS, and chlorobenzene under O2 at 90°C for 16 h furnished desired product 117 in 36%–90% yields (Scheme 17B). (Wang and Jiao, 2016) This is the first report of [4 + 2] C-H annulation with carbon–heteroatom synthons. Atom economy, O2 as a green oxidant, N2 and H2O as the only byproducts, broad substrate scope, and the formation of functionalized quinazoline are the benefits of this approach. Mechanistically, the reaction proceeds via initial cationic Cp*Rh(III) catalyst A formation with the help of AgSbF6. Subsequently, imidate group 45 directs C-H bond activation, generating rhodacyclic intermediate B, which can coordinate with benzyl azide 116 to give intermediate C. Subsequently, C undergoes migratory insertion to furnish Rh species. Protonation of complex D regenerates cationic Cp*Rh(III) A and produces intermediate E via amination. The amine undergoes Cu-catalyzed aerobic oxidation to produce the corresponding imine F with the removal of H2O as a byproduct. In the last step, cascade intramolecular addition and aerobic oxidative aromatization lead to the formation of quinazoline 117 (Scheme 17B).
Rh-azavinylcarbene (Rh-AVC) has evolved as a versatile intermediate for the formation of N-heterocycles (Davies and Alford, 2014). Rh-AVC can be readily generated from N-sulfonyl-1,2,3-triazoles via denitrogenation catalyzed by the Rh(II) system and is widely used as a [1C]-, [2C]-, and aza-[3C]-component in several transformations (Miura et al., 2012; Lei et al., 2015; Ryu et al., 2015). Interestingly, Tang et al. (2016) reported an unprecedented Rh(II)-catalyzed transannulation of 2,1-benzisoxazoles 118 with N-sulfonyl-1,2,3-triazoles 119. The cycloaddition of 2,1-benzisoxazoles 118 with N-sulfonyl-1,2,3-triazoles 119 catalyzed by Rh2 (esp)2 in 1,2-DCE at 160°C for 5 min and then with DBU at room temperature for 30 min delivered quinazolines 120 in 35%–83% yields (Scheme 17C). (Lei et al., 2016) Moreover, N-sulfonyl-1,2,3-triazole 119 acts as an aza-[2C]-component in this cycloaddition reaction, which represents the first example of using Rh(II)-AVC as an aza-[2C]-component in the relevant cycloadditions. However, 2,1-benzisoxazoles 118 with an H atom or phenyl group at the C-3 position failed to form the desired products, which limits the substrate scope; otherwise, it is a remarkable approach. The reaction mechanism (Scheme 17C) proposed by the authors suggests that the initial treatment of triazole 119 with Rh(II)- catalyst yields Rh-azavinylcarbene A, which then reacts with 2,1-benzisoxazole 118 to generate intermediate B via aza-[4 + 2] cycloaddition. Next, ring opening of B produces C, which then leads to oxonium ylide D. Subsequently, cleavage of the N-O bond results in intermediate E with the release of the Rh(II)-catalyst. At this stage, E can be further proceeded by two pathways. In one pathway, it can be converted to quinazoline 120 via elimination of TsH, followed by tautomerization. This process plays a key role in the presence of a DBU (path A). However, E could also undergo formal 1,3-sulfonyl migration (Xin et al., 2015) to generate intermediate G, which upon autoxidation with air delivers quinazoline derivative H (path B). However, concerted intramolecular 1,3-sulfonyl migration cannot be completely excluded. Non-etheless, a stepwise mechanism via a close ion-pair intermediate is more likely to account for the transformation from E to 120 based on crossover experiments.
Xu et al. (2018) demonstrated a rarely explored Rh(III)-catalyzed [5 + 1] annulation via direct C-H activation. The 5-exo-cyclization reaction of amidines 17c with diynes 121 in the presence of [Cp*RhCl2]2, Ag2CO3, 2,6-dimethylbenzoic acid, H2O, Li2CO3, and DMA/EtOH/NMP (2:1:2) in air at 80°C for 21 h resulted in the formation of quinazolines 122 in 25%–91% yields (Scheme 18A) (Xu et al., 2018).
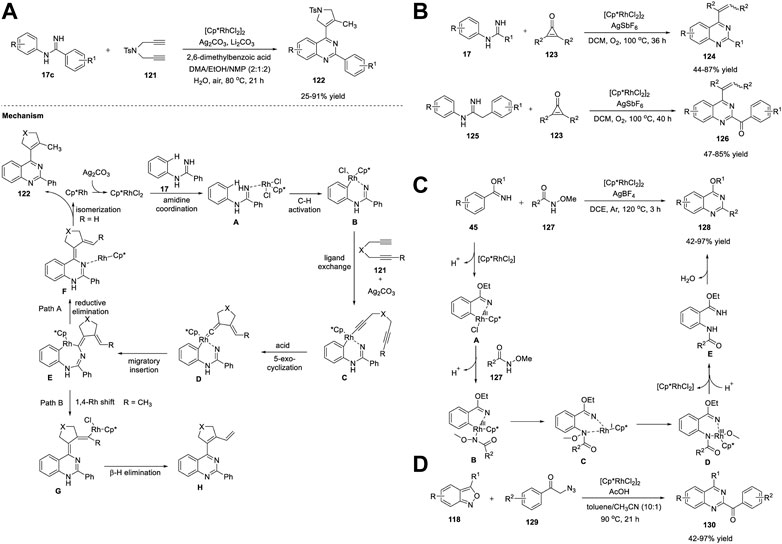
SCHEME 18. (A) Rh(III)-catalyzed [5 + 1] annulation for the synthesis of quinazolines (B) Rh(III)-catalyzed synthesis of quinazolines (C) Rh(III)-catalyzed one-pot cascade synthesis of quinazolines (D) Rh(III)-catalyzed tandem access to quinazolines.
Notable features of this strategy are as follows: a) diyne was used for the first time as a one-carbon precursor in C-H activation, b) amidines and diynes display high reactivity in air and water, c) broad functional group tolerance, and d) two heterocyclic rings are assembled in one step by cascade C-H activation/[5 + 1] annulation.
The reaction mechanism begins with the initial formation of Rh(III)-complex A. Next, C-H activation at the ortho-H of the amidine bond followed by ligand exchange generates rhodacycle C. The 5-exo-cyclization of C results in intermediate D, which upon migratory insertion yields intermediate E. Furthermore, E displayed two distinct pathways. In Path A, reductive elimination of E gives intermediate F with liberation of Cp*Rh(I), which then undergoes reoxidization into the Cp*Rh(III) catalyst by Ag2CO3 (Wang et al., 2017). Subsequently, demetalation followed by isomerization affords the [5 + 1] product, quinazoline 122 (Path A) (Yang et al., 2016). In Path B (Xu et al., 2017), for unsymmetrical diynes bearing a methyl group at one alkyne terminus, step-wise migratory insertion-1,4-rhodium shift-β-H elimination produces the dehydrogenation product H, as evidenced by NMR (Scheme 18A).
Adopting the [5 + 1] annulation strategy, Wu et al. (2020) demonstrated an unprecedented formation of 4-alkene quinazolines using a Rh(III) catalyst. In recent years, cyclopropenones have been widely used as appealing reaction partners, with ring-strain release as the driving force.
C-H activation between N-arylamidine and cyclopropenone catalyzed by [Cp*RhCl2]2 with AgSbF6 in DCM in O2 at 100°C for 36 h led to the construction of 4- ethenyl quinazolines in 44%–87% yields (Scheme 18B). (Xing et al., 2020) Moreover, 2-benzoyl quinazolines were obtained in good yields from C-benzyl imidamides via an additional oxidation step. Mild reaction conditions, high atom economy, and good substrate compatibility are merits of this protocol.
In the same year, Dong et al. (2020) took a step ahead and employed N-methoxyamide as an amidating agent for the first time, instead of dioxazolones or alky azides. The one-pot cascade reaction proceeded by the treatment of imidate esters with N-alkoxyamides utilizing [Cp*RhCl2]2 with AgBF4 in DCE in Ar at 120°C for 3 h, led to the production of quinazolines in 42%–97% yields (Scheme 18C). (Xu et al., 2020) Furthermore, under the standard reaction conditions, a series of N-methoxyamides, including heteroarylamides and 2-napthamides, gave the desired product in good yields. Moreover, the challenging aminating reagents from cinnamic acid derivatives also furnished the product, which could not be obtained using previously reported methods. The Rh(III)-catalyzed [4 + 2] reaction proceeds via C−H activation/amidation/annulation in a step-wise manner.
Very recently, Yu et al. (2022) reported that a novel Rh(III)-catalyzed tandem reaction of 2,1-benzisoxazoles and α-azido ketones in the presence of [Cp*RhCl2]2 as the catalyst, AcOH as the additive, and toluene/acetonitrile (10:1) as the solvent system at 90°C for 21 h delivered (quinazolin-2-yl)methanone derivatives in 21%–88% yields (Scheme 18D). (Liu et al., 2022) This tandem conversion involves Rh(III)-catalyzed denitrogenation of α-azido ketones, aza-[4 + 2] cycloaddition, ring opening, and dehydration aromatization. The key step in the synthesis is the aza-[4 + 2] cycloaddition of the imine Rh-complex with 2,1-benzisoxazole.
3.3 Palladium
Palladium is a non-abundant precious metal that displays variable oxidation states, high stability, and excellent selectivity. It can facilitate some exclusive transformations, such as cross-coupling reactions (Zhou et al., 2019) and hydrogenation (Reddy and Swamy, 2017).
Wu et al. (2014) reported the first carbonylative approach towards quinazoline formation from easily available 2-aminobenzylamine and aryl bromides. The carbonylative coupling of 2-aminobenzylamine 14 with aryl bromides 131 using Pd(OAc)2 and BuPAd2 with DBU as the base in DMSO at 140°C for 36 h generated quinazoline 132 scaffolds in <5%–93% yields (Scheme 19A). (Chen et al., 2014b) DMSO served both as a solvent and oxidant in this reaction. The one-pot reaction proceeded in a sequential manner, that is, aminocarbonylation, condensation, and oxidation. The substrate scope was extended to include several electron-donating and electron-withdrawing groups. Particularly, good to moderate yields were achieved, with the exception of multifluoro-substituted bromobenzene and more steric 2,4,6-trimethylbromobenzene, both of which failed to give the desired product.
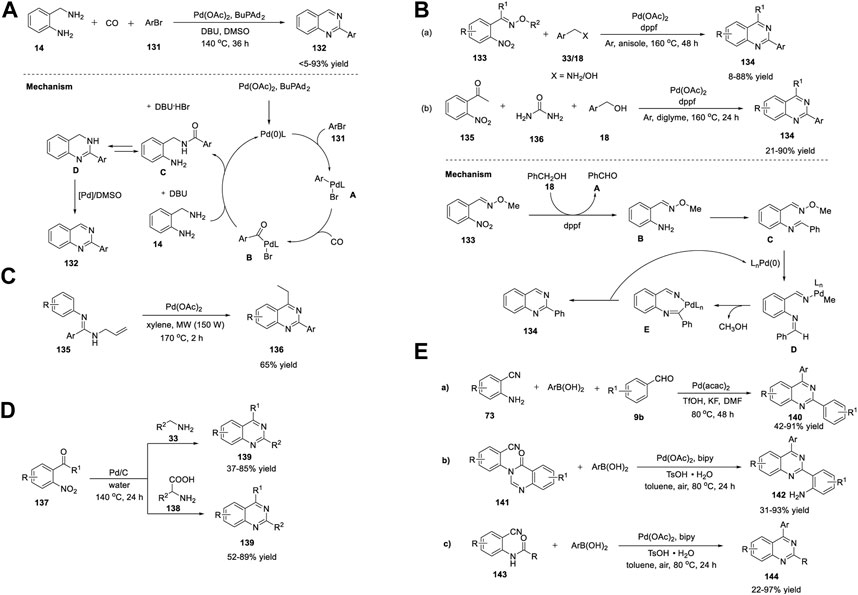
SCHEME 19. (A) Pd-catalyzed carbonylative synthesis of quinazolines (B) Pd-catalyzed carbonylative synthesis of quinazolines (C) Pd-catalyzed synthesis of quinazolines via hydrogen transfer strategy (D) Pd-catalyzed synthesis of quinazolines under microwave irradiation (E) Heterogenous Pd-catalyzed synthesis of quinazolines via HT approach.
The plausible mechanism depicted in Scheme 19A suggests that the reduced Pd(0) commenced the catalyst cycle, followed by the oxidative addition of aryl bromides 131 to Pd(0), resulting in the corresponding organopalladium species A. Subsequent coordination and insertion of CO delivered the key acylpalladium complex B. Nucleophilic attack of 14 intermediate B led to compound C and Pd(0) with the aid of a base. Compound C then underwent intramolecular cyclization to generate intermediate D, which was then oxidized to the terminal quinazoline product 132 in the presence of Pd and DMSO.
In the same year, another one-pot method was presented by Deng et al. (2014) for the efficient assembly of 2-aryl quinazolines using a Pd catalytic system for the first time (Scheme 19B). (Wang et al., 2014) This hydrogen transfer strategy proceeded via the reaction of a) (E)-2-nitrobenzaldehyde O-methyl oximes 133 and alcohols 18 or benzyl amines 33 or of b) 1-(2-nitrophenyl)ethenone 135, urea 136, and benzyl alcohols 18 under the optimized conditions of Pd(OAc)2 and DPPF (1,1′-Bis(diphenylphosphino)ferrocene) ligand in anisole/diglyme at 160°C for 24–48 h and produced 2-aryl quinazolines in 8%–90% yields (Scheme 19B). In this protocol, the nitro group was reduced by the hydrogen evolved from the alcohol dehydrogenation step. Mechanistically, benzaldehyde A is generated by the dehydrogenation of benzyl alcohol 18 and reduction of 133 to form intermediate B, which then undergoes condensation to as by-products. In the last step, the reductive elimination of E gave imine intermediate C. Oxidative addition, followed by palladation and cyclization, afford intermediate E and methanol results in quinazoline 134, and the active Pd(0) catalyst is released (Scheme 19B).
Wang et al. (2015) described Pd-catalyzed C-H activation under microwave irradiation for the synthesis of quinazolines. N-allylamidines 135 underwent C-H activation in the presence of Pd(OAc)2 in xylene under microwave irradiation (150 W) at 170°C for 2 h, resulting in quinazolines 136 in 65% yield and a mixture of quinazoline and imidazole in 73%–94% yields (Scheme 19C) (Xu et al., 2015), and the latter could be separated easily through column chromatography.
Tang et al. (2016) proposed another hydrogen transfer protocol utilizing a heterogeneous Pd-catalytic system for the efficient synthesis of 2,4-disubstituted quinazolines via the direct oxidative amination of C (sp3)-H bonds with nitro groups, which has not been previously reported. The first example of the reaction of o-nitroacetophenones with benzyl amines or amino acids catalyzed by Pd/C in water at 140°C for 24 h produced quinazolines in 37%–89% yields (Scheme 19D). (Tang et al., 2016) The reaction proceeded in a C-N bond cleavage formation sequence. Furthermore, when amino acids were used as substrates, the product was synthesized via decarboxylative oxidative amination. The recyclability of the catalyst for at least five times; broad substrate scope; tolerance to air; oxidant-, ligand-, and base-free; and usage of water as the solvent make this protocol remarkable.
Chen et al. (2018) reported the synthesis of quinazolines using three different methodologies: a) the one-pot reaction of 2-aminobenzonitriles, aldehydes, and arylboronic acids employing Pd (acac)2 as the catalyst, 5,5′-dimethyl-2,2′-bipyridine as the ligand, TfOH as the additive, and DMF as the solvent in KF at 80°C for 48 h produced quinazolines in 42%–91% yields (Scheme 19Ea) (Hu et al., 2018). This is the first report of one-pot, three-component tandem quinazoline synthesis via carbopalladation of the cyano group. b) The tandem sequential nucleophilic addition and intramolecular cyclization of readily available 2-(quinazolinone-3(4H)-yl)benzonitriles with arylboronic acids led to the preparation of quinazoline derivatives in 31%–93% yields in the presence of Pd(OAc)2, bipyridine, and TsOH·H2O in toluene in air at 80°C for 24 h (Scheme 19Eb) (Zhang et al., 2018b). c) The Pd(OAc)2-catalyzed tandem reaction of N-(2-cyanoaryl)benzamides with arylboronic acids in 1,10-phenanthroline, TFA, and THF at 80°C for 24 h delivered 2,4-disubstituted quinazolines in 22%–97% yields (Scheme 19Ec) (Zhu et al., 2018). The important advantages of these approaches are a) bromo and iodo group/amine group/halogen and hydroxyl groups were well tolerated under the optimized conditions, which are amenable to further synthetic transformations and b) excellent chemoselectivity.
Similarly, Wu et al. (2021) demonstrated Pd(OAc)2-catalyzed reductive carbonylation of N-(2-iodophenyl)benzimidamide 145 using P(C6F5)3 as the ligand, Ph2SiH2 as the hydrogen source, Mo(CO)6 as the carbonyl source, and triethylamine as the base in DMF at 120°C for 16 h, providing a series of quinazolines 146 in 48%–80% yields (Scheme 20A) (Lu et al., 2021).
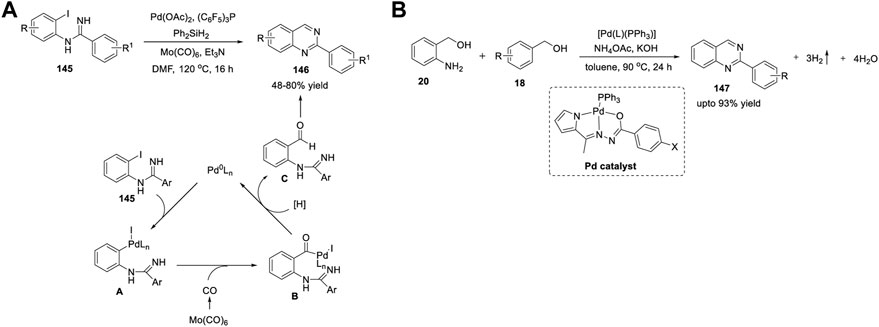
SCHEME 20. (A) Pd-catalyzed synthesis of quinazolines via reductive carbonylation (B) Pd-N,N,O-pincer type complex for the synthesis of quinazolines via double dehydrogenative coupling.
The considerable benefits of this protocol are that silane acts as a better nucleophile than amidine and has good functional group tolerance. An insight into the mechanistic investigation suggested that oxidative addition of Pd(0) gives arylpalladium species A. CO insertion followed by the generation of aldehyde intermediate C, aided by Ph2SiH2, is accompanied by the release of Pd(0) to close the catalytic cycle. Next, the intramolecular condensation of C leads to the formation of the desired quinazoline 146 (Scheme 20A).
Recently, Ramesh et al. (2022) demonstrated a new Pd(II) N,N,O-pincer-type catalyst for the sustainable synthesis of quinazolines via a sequential ADC strategy. The one-pot reaction of readily available alcohols 18 and 2-aminobenzylalcohol 20 in the presence of [Pd(L)(PPh3)], 4-substituted methyl-2-pyrrolyl benzhydrazone as the ligand, and ammonium acetate and KOH as the base in toluene at 90°C for 24 h produced the product 147, with up to 93% yield (Scheme 20B). (Anandaraj et al., 2021) The major benefits of this methodology are broad substrate scope, oxidant-free, and environmental friendliness.
3.4 Silver
Silver, a less toxic metal, displays excellent selectivity and stability, and is more economical than other precious transition metals. Due to its Lewis acid character, Ag can act as a cocatalyst, oxidant, radical precursor, etc. Silver provides a unique platform for synthetic transformations, such as olefin and alcohol oxidation (Kolobova et al., 2019), intramolecular cyclization (Yang et al., 2019b), cycloaddition (Liu et al., 2019), and C-H activation (Ju et al., 2019).
Sun et al. (2017) developed Ag/Pd NPs deposited on WO2.72 as an efficient catalytic system for high-yield synthesis of quinazoline scaffolds. The one-pot aromatization of ammonium formate, 2-nitroacetophenone 137, and aldehydes 9b in the presence of the Ag48Pd52/WO2.72 catalyst in dioxane and water at 60°C for 6 h afforded substituted quinazolines 148 in 88%–99% yields (Scheme 21). (Yu et al., 2017) In this green protocol, the catalyst could be recycled at least five times with no loss in activity.
4 Third-row transition metal catalysts
4.1 Iridium
Iridium complexes have emerged as attractive candidates for organic catalysis because of their stability, high conversion rates, short reaction times, and excellent enantioselectivity. Iridium catalysts perform a broad array of reactions such as hydrogen transfer reactions (Anxionnat et al., 2013), photoredox reactions (Kim et al., 2020), and C-H activation (Wang et al., 2019).
Zhou et al. (2013) reported a hydrogen transfer strategy for the efficient synthesis of 2-substituted quinazolines using an Ir catalyst. The one-pot reaction of 2-aminobenzylamine 14b and benzaldehydes 9c/benzyl alcohol 18 using [Cp*IrCl2]2 as the catalyst and styrene as the hydrogen acceptor in refluxing xylene for 24–48 h produced quinazolines 149 in 48%–66% yields (Scheme 22A). (Fang et al., 2013) Moreover, when benzyl alcohol 18 was used as the substrate, KOH was used as the base. Notably, a wide variety of aryl and alkyl aldehydes gave the desired product in good yields easily under the optimized conditions.
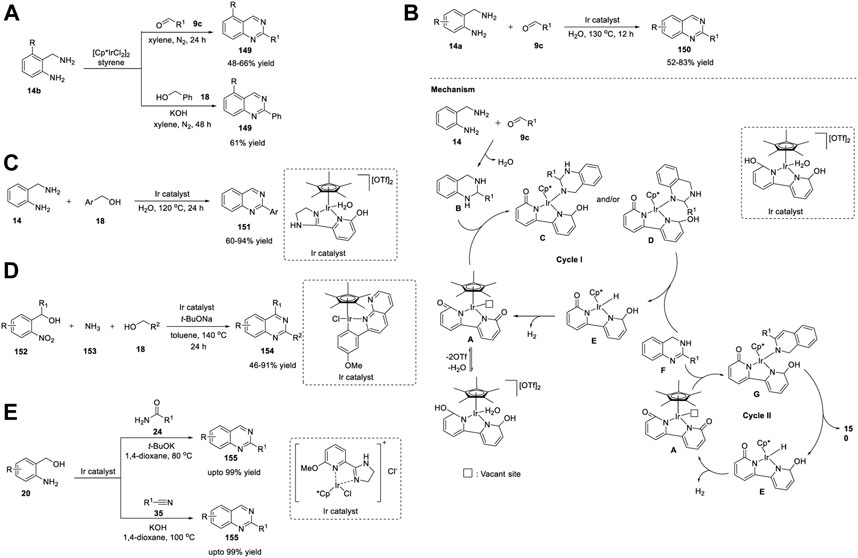
SCHEME 22. (A) Iridium catalyzed synthesis of quinazolines via hydrogen transfer strategy (B) Ir-catalyzed synthesis of quinazolines via acceptorless dehydrogenative cyclization (C) Ir-catalyzed synthesis of quinazolines via ligand promoted dehydrogenation (D) Naphthyridine-based Ir-catalyst for the synthesis of quinazolines (E) Ir-catalyzed synthesis of quinazolines via ADC reaction.
Similarly, Li et al. (2017) identified a novel water-soluble metal–ligand bifunctional Ir catalyst for the facile construction of quinazoline moieties via ADC. In the presence of the [Cp*Ir (6,6’-(OH)2bpy)(H2O)][OTf]2 catalyst, the ADC reaction between 2-aminobenzyl amines 14a and benzaldehydes 9c at 130°C for 12 h in water afforded quinazolines 150 in 52%–83% yields via ligand-promoted dehydrogenation (Scheme 22B). (Fan et al., 2017) Furthermore, electron-donating and electron-withdrawing groups on the aromatic ring and aliphatic aldehydes resulted in the corresponding products 150 in good yields. The attractive benefits of this environmentally benign approach include high atom efficiency and being oxidant- and organic solvent-free.
An insight into the mechanistic investigation depicted in Scheme 22B explains that the first step is the elimination of TfOH and water from the catalyst to give unsaturated species A. In cycle I, the ligand present in A accepts a proton from tetrahydroquinazoline B, which in turn is formed through condensation between 2-aminobenzylamine 14 and aldehyde 9c. This results in the formation of C and/or D, which then undergo β-hydrogen elimination to form the iridium hydride species E and dihydroquinazoline F. Simultaneous transfer hydrogenation from the hydroxyl proton on the bipyridine ligand and the hydride on the iridium results in hydrogen gas liberation and regenerated catalytic species A. In cycle II, the ligand of species A accepts a proton of dihydroquinazoline F to furnish species G, which then undergoes β-hydrogen elimination to give iridium hydride species E and quinazoline 150.
Based on the above-mentioned ligand-promoted dehydrogenation concept, Kundu et al. (2019) demonstrated a cooperative iridium complex-catalyzed synthesis of quinazolines 151 in 60%–94% yields via the treatment of 2-amino benzylamines 14 and alcohols 18 in water at 120°C for 24 h (Scheme 22C) (Chakrabarti et al., 2019).
In addition, 1-naphthalenemethanol and heteroatom-containing primary alcohols such as furfuryl alcohol, piperonyl alcohol, nicotinyl alcohol, and 2-thiophenemethanol, were tolerated well under these conditions and produced good yields. To date, there have been no reports of the generation of quinazolines from bio-renewable alcohols in water. The salient features of this approach are oxidant- and base-free reactions and usage of water as a green solvent.
Recently, Zhang et al. (2020) reported the first example of a novel iridium complex featuring a 2-(4-methoxyphenyl)-1,8-naphthyridyl ligand for the hydrogen-transfer-mediated annulation of 2-nitrobenzylic alcohols 152, ammonia 153, and alcohols 18. The reaction proceeded using t-BuONa as the base in toluene at 140°C for 24 h and generated quinazolines 154 in 46%–91% yields (Scheme 22D). (Tan et al., 2020) The novelty of this transformation lies in the utilization of hydrogen for substrate activation through transfer hydrogenation (TH) of the nitro group, which in turn is generated from the dehydrogenation of alcohols and the dehydroaromatization process and hence does not require external reductants. The non-coordinated N-atom in the ligand significantly promoted the condensation step via hydrogen bonding. The major advantages of this method are its high step and atom efficiency, good substrate and functional group compatibility, and non-toxic byproduct such as water.
Very recently, Luo et al. (2022) reported an efficient iridium-catalyzed ADC reaction between 2-aminoarylmethanols 20 and amides 24/nitriles 35 in the presence of t-BuOK/KOH in 1,4-dioxane at 80°C–100°C to provide quinazolines 155 (Scheme 22E). (Shui et al., 2022) Excellent yields of up to 99% were achieved by employing a variety of substituted 2-aminobenzyl alcohols 20, (hetero)aryl or alkyl benzamides 24, and nitriles 35. The salient features of this protocol are mild reaction conditions, a simple operation procedure, and high atom economy.
4.2 Platinum
Platinum metals provide synthetic routes to many useful and interesting compounds. Pt catalysts have proven their versatility in several transformations such as hydrogenation (Zhang et al., 2016), hydrosilylation (Lukin et al., 2020), hydroamination (Bender and Widenhoefer, 2005), and cyclic-isomerization (Lu et al., 2009).
Shimizu et al. (2014) reported the first acceptorless method for facile access to quinazolines 156 in 50%–93% yields from 2-aminobenzyl amine 14 and alcohols 18/aldehydes 9c, with the reaction catalyzed by a CeO2-supported Pt catalyst in mesitylene under reflux for 30–48 h (Scheme 23A). (Chaudhari et al., 2014) The major merits of this strategy include good catalyst reusability, low catalyst loading, wide substrate scope, and first acceptor- and additive-free catalytic systems.
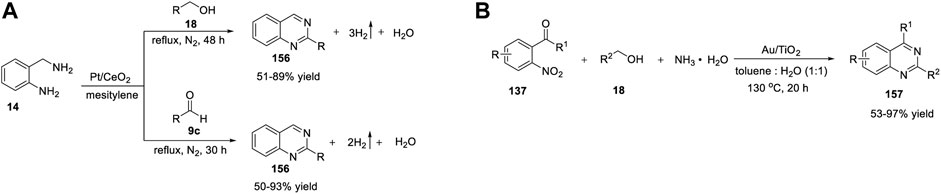
SCHEME 23. (A) Pt NPs catalyzed synthesis of quinazolines via acceptorless dehydrogenation (B) TiO2-supported Au-NPs for the synthesis of quinazolines via hydrogen transfer strategy.
4.3 Gold
Gold, a noble metal, possesses exclusive catalytic activities, exhibits excellent chemoselectivity, resistance to oxygen, and orthogonal reactivity, and requires mild reaction conditions. Au extends the route to a new class of homogenous and heterogeneous catalysis for a broad array of reactions such as epoxidation (Ji et al., 2018), cycloisomerization reactions (Hu et al., 2020), coupling reactions (Nijamudheen and Datta, 2019), and total syntheses of natural products (Pflasterer and Hashmi, 2016).
Wang et al. (2015) demonstrated an efficient and selective nitrogen-source-promoted hydrogen-transfer strategy for the construction of 2,4-disubstituted quinazolines 157 from o-nitroacetophenones 137, alcohols 18, and NH3·H2O (N source), with the reaction catalyzed by Au/TiO2 in the presence of toluene:H2O (1:1) at 130°C for 20 h (Scheme 23B). (Tang et al., 2015) A variety of alcohols 18 and nitro compounds 137 underwent the transformation smoothly in aqueous media, resulting in 53%–97% yields. This one-pot cascade reaction includes the dehydrogenative oxidation of alcohols and C-N bonds, reduction of the nitro group, and condensation of aldehydes with amines. The benefits of this protocol are as follows: a) no requirement of additional oxidant/additive/reducing agent, b) multiple C-N bond formation, c) good tolerance to air and water, and d) recyclable and reusable catalyst.
5 Conclusion
This review summarizes the methodology and importance of transition-metal catalysis in the synthesis of medicinally important quinazolines over the past decade. Reaction mechanisms are provided for every representative example, along with the advantages and limitations associated with it. Significant progress accomplished by first-row transition metals, such as Ti, Mn, Fe, Co, Ni, and Cu, is due to their high abundance, low toxicity, and low cost. Synthetic transformations performed by these metals include heterogeneous catalysis, multicomponent reactions, cyclization, one-pot or cascade or tandem reactions, C-N cross coupling, Ullmann-type condensation, aerobic oxidation, oxidative amination, C(sp3)–H/C(sp2)–H oxidative annulation, [4 + 2] cycloaddition, and annulation {[2 + 2 + 2], [3 + 2 + 1], [4 + 1 + 1]}. Despite their low availability and high cost, second- (Ru, Rh, Pd, and Ag) and third-row (Ir, Pt, and Au) transition metals catalyze unique transformations owing to their high stability and excellent selectivity. The majority of these are ADC, C-H activation ([4 + 2], aza-[4 + 2], [5 + 1]) and annulation, hydrogen transfer strategy, ligand-promoted dehydrogenative reaction, carbonylation, carbopalladation, and the use of NPs for the successful synthesis of quinazolines. In addition to homogeneous catalysis, heterogeneous catalytic approaches have also been adopted in the form of nanoporous octahedral molecular sieves, nanocomposites, ionic liquids, molecular organic frameworks, nanocubes, and magnetically recoverable nanocatalysts for the efficient synthesis of quinazolines. These catalysts tend to exhibit improved sustainability, stability, catalytic site availability, reusability, and recovery. Some of the reactions were performed using water as the green solvent and air as an oxidant, thus avoiding the use of organic solvents and excess oxidants and making the synthesis environmentally benign. New protocols with good substrate compatibility, chemoselectivity, simple operating conditions, readily available precursors, high atom economy, and high yields are always in demand. Based on the results, we believe that this review will provide a sufficient overview of transition-metal-catalyzed synthesis of quinazolines and encourage synthetic and medicinal chemists for further development in the future.
Author contributions
Conceptualization, RT, S-HK and DS; methodology, RT and DS; validation, RT and DS; formal analysis, RT; investigation, S-HK and DS; resources, S-HK and DS; writing—original draft preparation, RT, S-HK and DS; writing—review and editing, RT, S-HK and DS; supervision, S-HK and DS; project administration, S-HK and DS; funding acquisition, S-HK and DS. All authors have read and agreed to the published version of the manuscript.
Funding
This research was funded by the National Research Foundation of Korea (NRF-2020R1A6A1A03043708, NRF-2021R1A2C1012280 and NRF-2020R1F1A1075782).
Conflict of interest
The authors declare that the research was conducted in the absence of any commercial or financial relationships that could be construed as a potential conflict of interest.
Publisher’s note
All claims expressed in this article are solely those of the authors and do not necessarily represent those of their affiliated organizations, or those of the publisher, the editors and the reviewers. Any product that may be evaluated in this article, or claim that may be made by its manufacturer, is not guaranteed or endorsed by the publisher.
References
Alsalahi, W., and Trzeciak, A. M. (2021). Rhodium-catalyzed hydroformylation under green conditions: Aqueous/organic biphasic, “on water”, solventless and Rh nanoparticle based systems. Coord. Chem. Rev. 430, 213732–213755. doi:10.1016/j.ccr.2020.213732
Anandaraj, P., Ramesh, R., and Kumaradhas, P. (2021). Palladium(ii) N,N,O-pincer type complex-mediated dehydrogenative coupling of alcohols to quinazolines. New J. Chem. 45, 16572–16580. doi:10.1039/d1nj03146e
Anwarul Islam, A. K. M., Kashem, M. A., Shameem, I. A., and Kibria, S. A. M. G. (2005). Efficacy of terazosin and finasteride in symptomatic benign prostatic hyperplasia: A comparative study. Bangladesh Med. Res. Counc. Bull. 31, 54–61.
Anxionnat, B., Pardo, D. G., Ricci, G., Rossen, K., and Cossy, J. (2013). Iridium-catalyzed hydrogen transfer: Synthesis of substituted benzofurans, benzothiophenes, and indoles from benzyl alcohols. Org. Lett. 15, 3876–3879. doi:10.1021/ol401610e
Arachchige, P. T. K., and Yi, C. S. (2019). Synthesis of quinazoline and quinazolinone derivatives via ligand-promoted ruthenium-catalyzed dehydrogenative and deaminative coupling reaction of 2-aminophenyl ketones and 2-aminobenzamides with amines. Org. Lett. 21, 3337–3341. doi:10.1021/acs.orglett.9b01082
Auti, P. S., George, G., and Paul, A. T. (2020). Recent advances in the pharmacological diversification of quinazoline/quinazolinone hybrids. RSC Adv. 10, 41353–41392. doi:10.1039/d0ra06642g
Baghbanian, S. M., and Farhang, M. (2014). CuFe2O4 nanoparticles: A magnetically recoverable and reusable catalyst for the synthesis of quinoline and quinazoline derivatives in aqueous media. RSC Adv. 4, 11624–11633. doi:10.1039/c3ra46119j
Based on annual sales of drugs in the year 2009 (2009). Based on annual sales of drugs in the year 2009. see:. downloaded March 12, 2011 Available at: http://www.drugs.com/top200.html.
Battula, S., Vishwakarma, R. A., and Ahmed, Q. N. (2014). Cu–benzotriazole-catalyzed electrophilic cyclization of N-arylimines: A methodical tandem approach to O-protected-4hydroxyquinazolines. RSC Adv. 4, 38375–38378. doi:10.1039/c4ra07377k
Bender, C. F., and Widenhoefer, R. A. (2005). Platinum-catalyzed intramolecular hydroamination of unactivated olefins with secondary alkylamines. J. Am. Chem. Soc. 127, 1070–1071. doi:10.1021/ja043278q
Bhattacharyya, D., Adhikari, P., Deori, K., and Das, A. (2022). Ruthenium pincer complex catalyzed efficient synthesis of quinoline, 2-styrylquinoline and quinazoline derivatives via acceptorless dehydrogenative coupling reactions. Catal. Sci. Technol. 12, 5695–5702. doi:10.1039/d2cy01030e
Bottaro, F., and Madsen, R. (2019). In situ generated cobalt catalyst for the dehydrogenative coupling of alcohols and amines into imines. ChemCatChem 11, 2707–2712. doi:10.1002/cctc.201900392
Brittain, W. D. G., Buckley, B. R., and Fossey, J. S. (2016). Asymmetric copper-catalyzed azide–alkyne cycloadditions. ACS Catal. 6, 3629–3636. doi:10.1021/acscatal.6b00996
Bule, M. H., Ahmed, I., Maqbool, F., and Zia, M. A. (2017). Quinazolinone derivatives as a potential class of compounds in malaria drug discovery. Int. J. Pharmacol. 13, 818–831. doi:10.3923/ijp.2017.818.831
Cakici, M., Catir, M., Karabuga, S., Kilic, H., Ulukanli, S., Gulluce, M., et al. (2010). Synthesis and biological evaluation of (S)-4-aminoquinazoline alcohols. Tetrahedron Asymmetry 21, 2027–2031. doi:10.1016/j.tetasy.2010.05.040
Caneschi, A., Grand, A., Laugier, J., Rey, P., and Subra, R. (1988). Three-center binding of a nitroxyl free radical to copper(II) bromide. J. Am. Chem. Soc. 110, 2307–2309. doi:10.1021/ja00215a055
Chakrabarti, K., Maji, M., and Kundu, S. (2019). Cooperative iridium complex-catalyzed synthesis of quinoxalines, benzimidazoles and quinazolines in water. Green Chem. 21, 1999–2004. doi:10.1039/c8gc03744b
Chakraborty, G., Sikari, R., Das, S., Mondal, R., Sinha, S., Banerjee, S., et al. (2019). Dehydrogenative synthesis of quinolines, 2-aminoquinolines, and quinazolines using singlet diradical Ni(II)-Catalysts. J. Org. Chem. 84, 2626–2641. doi:10.1021/acs.joc.8b03070
Chandrikaa, P. M., Rao, A. R. R., Narsaiah, B., and Raju, M. B. (2008). Quinazoline derivatives with potent anti-inflammatory and anti-allergic activities. Int. J. Chem. Sci. 6, 1119–1146.
Charpe, V. P., Ragupathi, A., Sagadevan, A., and Hwang, K. C. (2021). Photoredox synthesis of functionalized quinazolines via copper-catalyzed aerobic oxidative Csp2–H annulation of amidines with terminal alkynes. Green Chem. 23, 5024–5030. doi:10.1039/d1gc01493e
Chau, N. G., and Haddad, R. I. (2013). Vandetanib for the treatment of medullary thyroid cancer. Clin. Cancer Res. 19, 524–529. doi:10.1158/1078-0432.ccr-12-2353
Chaudhari, C., Siddiki, S. M. A. H. N., Tamura, M., and Shimizu, K. (2014). Acceptorless dehydrogenative synthesis of 2-substituted quinazolines from 2-aminobenzylamine with primary alcohols or aldehydes by heterogeneous Pt catalysts. RSC Adv. 4, 53374–53379. doi:10.1039/c4ra09205h
Chen, C. Y., He, F., Tang, G., Yuan, H., Li, N., Wang, J., et al. (2018). Synthesis of quinazolines via an iron-catalyzed oxidative amination of N–H ketimines. J. Org. Chem. 83, 2395–2401. doi:10.1021/acs.joc.7b02943
Chen, J., Natte, K., Neumann, H., and Wu, X. F. (2014). A convenient palladium-catalyzed carbonylative synthesis of quinazolines from 2-aminobenzylamine and aryl bromides. RSC Adv. 4, 56502–56505. doi:10.1039/c4ra11303a
Chen, L., Yang, W. L., Shen, J. H., and Deng, W. P. (2019). Nickel(II)-Catalyzed diastereo- and enantioselective michael/hemiacetalization cascade reaction of α-ketoesters with 2-(2-nitrovinyl)phenolsphenols. Adv. Synthesis Catal. 361, 4611–4622. doi:10.1002/adsc.201900753
Chen, M., Zhang, M., Xiong, B., Tan, Z., Lv, W., and Jiang, H. (2014). A novel ruthenium-catalyzed dehydrogenative synthesis of 2-arylquinazolines from 2-aminoaryl methanols and benzonitriles. Org. Lett. 16, 6028–6031. doi:10.1021/ol503052s
Chen, Z., Chen, J., Liu, M., Ding, J., Gao, W., Huang, X., et al. (2013). Unexpected copper-catalyzed cascade synthesis of quinazoline derivatives. J. Org. Che 78, 11342–11348. doi:10.1021/jo401908g
Choudhury, S., and Singh, M. S. (2012). Recent developments in solvent-free multicomponent reactions: A perfect synergy for eco-compatible organic synthesis. RSC Adv. 2, 4547–4592. doi:10.1039/c2ra01056a
Das, K., Mondal, A., Pal, D., and Srimani, D. (2019). Sustainable synthesis of quinazoline and 2-aminoquinoline via dehydrogenative coupling of 2-aminobenzyl alcohol and nitrile catalyzed by phosphine-free manganese pincer complex. Org. Lett. 21, 3223–3227. doi:10.1021/acs.orglett.9b00939
Davies, H. M. L., and Alford, J. S. (2014). Reactions of metallocarbenes derived from N-sulfonyl-1,2,3-triazoles. Chem. Soc. Rev. 43, 5151–5162. doi:10.1039/c4cs00072b
Ding, W., and Yoshikai, N. (2019). Cobalt-catalyzed intermolecular [2+2] cycloaddition between alkynes and allenes. Angew. Chem. Int. Ed. 58, 2500–2504. doi:10.1002/anie.201813283
Duan, T., Zhai, T., Liu, H., Yan, Z., Zhao, Y., Feng, L., et al. (2016). One-pot three-component synthesis of quinazolines via a copper-catalysed oxidative amination reaction. Org. Biomol. Chem. 14, 6561–6567. doi:10.1039/c6ob00625f
Duarah, G., Kaishap, P. P., Begum, T., and Gogoi, S. (2018). Recent advances in ruthenium(II)-Catalyzed C−H bond activation and alkyne annulation reactions. Adv. Synth. Catal. 361, 654–672. doi:10.1002/adsc.201800755
Dutta, M., Bania, K. K., and Pratihar, S. (2019). Remote ‘imidazole’ based ruthenium(II) p -cymene precatalyst for selective oxidative cleavage of C−C multiple bonds. ChemCatChem 11, 2683–2694. doi:10.1002/cctc.201900242
Faisal, M., and Saeed, A. (2021). Chemical insights into the synthetic chemistry of quinazolines: Recent advances. Front. Chem. 8, 594717–594740. doi:10.3389/fchem.2020.594717
Fan, H., Zhang, W., Zhao, W., and Li, F. (2017). Acceptorless dehydrogenative cyclization ofo-aminobenzylamines and aldehydes to quinazolines in water catalyzed by a water-soluble metal-ligand bifunctional catalyst. Chem. Accept. 2, 5735–5739. doi:10.1002/slct.201700871
Fan, X., Li, B., Guo, S., Wang, Y., and Zhang, X. (2014). Synthesis of quinazolines and tetrahydroquinazolines: Copper-catalyzed tandem reactions of 2-bromobenzyl bromides with aldehydes and aqueous ammonia or amines. Chem. Asian J. 9, 739–743. doi:10.1002/asia.201301296
Fang, J., Zhou, J., and Fang, Z. (2013). Synthesis of 2-substituted quinazolinesvia iridium catalysis. RSC Adv. 3, 334–336. doi:10.1039/c2ra22278g
Fujimoto, H., Kusano, M., Kodama, T., and Tobisu, M. (2019). Cyclization of bisphosphines to phosphacycles via the cleavage of two carbon–phosphorus bonds by nickel catalysis. Org. Lett. 21, 4177–4181. doi:10.1021/acs.orglett.9b01355
Getman, R. B., Bae, Y. S., Wilmer, C. E., and Snurr, R. Q. (2012). Review and analysis of molecular simulations of methane, hydrogen, and acetylene storage in metal–organic frameworks. Chem. Rev. 112, 703–723. doi:10.1021/cr200217c
Gilson, P. R., Tan, C., Jarman, K. E., Lowes, K. N., Curtis, J. M., Nguyen, W., et al. (2017). Optimization of 2-anilino 4-amino substituted quinazolines into potent antimalarial agents with oral in vivo activity. J. Med. Chem. 60, 1171–1188. doi:10.1021/acs.jmedchem.6b01673
Gopalaiah, K., Saini, A., and Devi, A. (2017). Iron-catalyzed cascade reaction of 2-aminobenzyl alcohols with benzylamines: Synthesis of quinazolines by trapping of ammonia. Org. Biomol. Chem. 15, 5781–5789. doi:10.1039/c7ob01159h
Griess, C. (1869). Ueber die Einwirkung des Cyans auf Anthranilsäure. Berichte Dtsch. Chem. Ges. 2, 415–418. doi:10.1002/cber.186900201180
Gu, Q. S., Li, Z. L., and Liu, X. Y. (2020). Copper(I)-Catalyzed asymmetric reactions involving radicals. Acc. Chem. Res. 53, 170–181. doi:10.1021/acs.accounts.9b00381
Güçlü-Üstündağ, Ö., and Mazza, G. (2007). Saponins: Properties, applications and processing. Crit. Rev. Food Sci. Nutr. 47, 231–258. doi:10.1080/10408390600698197
Gupta, O. P., Sharma, M. L., Ghatak, B. J., and Atal, C. K. (1977). Pharmacological investigations of vasicine and vasicinone-the alkaloids of Adhatoda vasica. Indian J. Med. Res. 66, 680–691.
Hachiya, I., and Shimizu, S. N. M. (2019). Titanium tetraiodide/trimethylsilyl iodide synergistically induced cyclization of N-(2-Cyanophenyl)benzamides into 2-Aryl-4-iodoquinazolines. ACS Omega 4, 10463–10473. doi:10.1021/acsomega.9b01199
Hallett, J. P., and Welton, T. (2011). Room-temperature ionic liquids: Solvents for synthesis and catalysis. 2. Chem. Rev. 111, 3508–3576. doi:10.1021/cr1003248
Hameed, A., Al-Rashida, M., Uroos, M., Ali, S. A., Arshia Ishtiaq, M., Mohammed, K. K., et al. (2018). Quinazoline and quinazolinone as important medicinal scaffolds: A comparative patent review (2011–2016). Expert Opin. Ther. Pat. 28, 281–297. doi:10.1080/13543776.2018.1432596
Han, B., Yang, X. L., Wang, C., Bai, Y. W., Pan, T. C., Chen, X., et al. (2012). CuCl/DABCO/4-HO-TEMPO-Catalyzed aerobic oxidative synthesis of 2-substituted quinazolines and 4H-3,1-Benzoxazines. J. Org. Chem. 77, 1136–1142. doi:10.1021/jo2020399
Hayashi, S., and Hamaguchi, H. (2004). Discovery of a magnetic ionic liquid [bmim]FeCl4. Chem. Lett. 33, 1590–1591. doi:10.1246/cl.2004.1590
Higgins, B., Kolinsky, K., Smith, M., Beck, G., Rashed, M., Adames, V., et al. (2004). Antitumor activity of erlotinib (OSI-774, Tarceva) alone or in combination in human non-small cell lung cancer tumor xenograft models. Anticancer. Drugs 15, 503–512. doi:10.1097/01.cad.0000127664.66472.60
Hoyt, J. M., Schmidt, V. A., Tondreau, A. M., and Chirik, P. J. (2015). Iron-catalyzed intermolecular [2+2] cycloadditions of unactivated alkenes. Science 349, 960–963. doi:10.1126/science.aac7440
Hu, C., Wang, T., Rudolph, M., Oeser, T., Asiri, A. M., and Hashmi, A. S. K. (2020). Gold(I)-Catalyzed cycloisomerization of 3-Alkoxyl-1,6-diynes: A Facile access to bicyclo[2.2.1]hept-5-en-2-ones. Angew. Chem. Int. Ed. 59, 8522–8526. doi:10.1002/anie.201914284
Hu, K., Zhen, Q., Gong, J., Cheng, T., Qi, L., Shao, Y., et al. (2018). Palladium-catalyzed three-component tandem process: One-pot assembly of quinazolines. Org. Lett. 20, 3083–3087. doi:10.1021/acs.orglett.8b01070
Huang, C., Zhou, Y., Yu, X. X., Wang, L. S., Wu, Y. D., and Wu, A. X. (2021). I2/CuCl2-Copromoted formal [4 + 1 + 1] cyclization of methyl ketones, 2-aminobenzonitriles, and ammonium acetate: Direct access to 2-Acyl-4-aminoquinazolines. J. Org. Chem. 86, 16916–16925. doi:10.1021/acs.joc.1c02096
Huo, S., Kong, S., Zeng, G., Feng, Q., Hao, Z., Han, Z., et al. (2021). Efficient access to quinolines and quinazolines by ruthenium complexes catalyzed acceptorless dehydrogenative coupling of 2-aminoarylmethanols with ketones and nitrile. Molec. Catal. 514, 111773–111782.
Jafari, E., Khajouei, M. R., Hassanzadeh, F., Hakimelahi, G. H., and Khodarahmi, G. A. (2016). Quinazolinone and quinazoline derivatives: Recent structures with potent antimicrobial and cytotoxic activities. Res. Pharm. Sci. 11, 1–14.
Janssen, T., Severin, R., Diekmann, M., Friedemann, M., Haase, D., Saak, W., et al. (2010). Bis(η5:η1-pentafulvene)titanium complexes: Catalysts for intramolecular alkene hydroamination and reagents for selective reactions with N−H acidic substrates. Organometallics 29, 1806–1817. doi:10.1021/om100056q
Ji, J., Lu, Z., Lei, Y., and Turner, C. H. (2018). Theoretical studies on the direct propylene epoxidation using gold-based catalysts: A mini-review. Catalysts 8, 421–444. doi:10.3390/catal8100421
Jia, F. C., Zhou, Z. W., Xu, C., Cai, Q., Li, D. K., and Wu, A. X. (2015). Expeditious synthesis of 2-Phenylquinazolin-4-amines via a Fe/Cu relay-catalyzed domino strategy. Org. Lett. 17, 4236–4239. doi:10.1021/acs.orglett.5b02020
Johnston, S., Pippen, J., Pivot, X., Lichinitser, M., Sadeghi, S., Dieras, V., et al. (2009). Lapatinib combined with letrozole versus letrozole and placebo as first-line therapy for postmenopausal hormone receptor–positive metastatic breast cancer. J. Clin. Oncol. 27, 5538–5546. doi:10.1200/jco.2009.23.3734
Ju, J., Hua, R., and Su, J. (2012). Copper-catalyzed three-component one-pot synthesis of quinazolines. Tetrahedron 68, 9364–9370. doi:10.1016/j.tet.2012.09.035
Ju, M., Huang, M., Vine, L. E., Dehghany, M., Roberts, J. M., and Schomaker, J. M. (2019). Tunable catalyst-controlled syntheses of β- and γ-amino alcohols enabled by silver-catalysed nitrene transfer. nitrene 2, 899–908. doi:10.1038/s41929-019-0339-y
Kathiravan, S., Suriyanarayanan, S., and Nicholls, I. A. (2019). Electrooxidative amination of sp2 C–H bonds: Coupling of amines with aryl amides via copper catalysis. Org. Lett. 21, 1968–1972. doi:10.1021/acs.orglett.9b00003
Kazemnejadi, M., Nasseri, M. A., Sheikh, S., Rezazadeh, Z., and Gol, S. A. A. (2021). Fe3O4@Sap/Cu(ii): An efficient magnetically recoverable green nanocatalyst for the preparation of acridine and quinazoline derivatives in aqueous media at room temperature. RSC Adv. 11, 15989–16003. doi:10.1039/d1ra01373d
Kim, J., Kang, B., and Hong, S. H. (2020). Direct allylic C(sp3)–H thiolation with disulfides via visible light photoredox catalysis. ACS Catal. 10, 6013–6022. doi:10.1021/acscatal.0c01232
Kolobova, E. N., Pestryakov, A. N., Bogdanchikova, N., and Corberan, V. C. (2019). Silver catalysts for liquid-phase oxidation of alcohols in green chemistry: Challenges and outlook. Catal. Today 333, 81–88. doi:10.1016/j.cattod.2018.06.030
Kunes, J., Bazant, J., Pour, M., Waisser, K., Slosarek, M., and Janota, J. (2000). Quinazoline derivatives with antitubercular activity. Farmaco 55, 725–729. doi:10.1016/s0014-827x(00)00100-2
Lane, E. M., Zhang, Y., Hazari, N., and Bernskoetter, W. H. (2019). Sequential hydrogenation of CO2 to methanol using a pincer iron catalyst. Organometallics 38, 3084–3091. doi:10.1021/acs.organomet.9b00413
Lei, X., Gao, M., and Tang, Y. (2016). Rh(II)-Catalyzed transannulation of N-Sulfonyl-1,2,3-Triazoles with 2,1-benzisoxazoles or 1,2-benzisoxazoles. Org. Lett. 18, 4990–4993. doi:10.1021/acs.orglett.6b02454
Lei, X., Li, L., He, Y.-P., and Tang, Y. (2015). Rhodium(II)-Catalyzed formal [3 + 2] cycloaddition of N-Sulfonyl-1,2,3-triazoles with isoxazoles: Entry to polysubstituted 3-aminopyrroles. Org. Lett. 17, 5224–5227. doi:10.1021/acs.orglett.5b02570
Li, B., Li, C., Tian, L., Zhou, J., Huang, J., and Meng, X. (2018). Heterogeneous oxidative synthesis of quinazolines over OMS-2 under ligand-free conditions. New J. Chem. 42, 15985–15989. doi:10.1039/c8nj02551g
Li, C., An, S., Zhu, Y., Zhang, J., Kang, Y., Liu, P., et al. (2014). Copper-catalyzed intermolecular cyclization of nitriles and 2-aminobenzylamine for 3,4-dihydroquinazolines and quinazolines synthesis via cascade coupling and aerobic oxidation. RSC Adv. 4, 49888–49891. doi:10.1039/c4ra09240f
Li, C. C., Dai, X. J., Wang, H., Zhu, D., Gao, J., and Li, C. J. (2018). Iron-catalyzed nucleophilic addition reaction of organic carbanion equivalents via hydrazones. Org. Lett. 20, 3801–3805. doi:10.1021/acs.orglett.8b01391
Li, J. G., Hu, Y. F., Sun, S. F., Ling, S., and Zhang, J. Z. (2012). Ionic structures of nanobased FeCl3/[C4mim]Cl ionic liquids. J. Phys. Chem. 116, 6461–6464. doi:10.1021/jp206819h
Li, W., Guo, L., and Li, W. (2017). Anilinotropone nickel catalyzed tandem ethylene oligomerization and Friedel-Crafts addition to toluene. Mol. Catal. 433, 122–127. doi:10.1016/j.mcat.2016.12.019
Li, Z., Dong, J., Zhang, H., Zhang, Y., Wang, H., Cui, X., et al. (2021). Sonochemical catalysis as a unique strategy for the fabrication of nano-/micro-structured inorganics. Nanoscale Adv. 3, 41–72. doi:10.1039/d0na00753f
Li, Z., Torres-Ochoa, R. O., Wang, Q., and Zhu, J. (2020). Functionalization of remote C(sp3)-H bonds enabled by copper-catalyzed coupling of O-acyloximes with terminal alkynes. Nat. Commun. 11, 403. doi:10.1038/s41467-020-14292-2
Liang, E., Wu, Y., Chen, J., Xiong, W., Zhao, J., Yao, X., et al. (2019). Copper-catalyzed aerobic oxidative cyclization protocol for the synthesis of quinazolines via amination of C(sp3)-H bonds of methylazaarenes. Tetrahedron 75, 130783–130789. doi:10.1016/j.tet.2019.130783
Lipunova, G. N., Nosova, E. V., Charushin, V. N., and Chupakhin, O. N. (2018). Functionalized quinazolines and pyrimidines for optoelectronic materials. Curr. Org. Synth. 15, 793–814. doi:10.2174/1570179415666180622123434
Liu, J. Q., Shen, X., Shatskiy, A., Zhou, E., Karkas, M. D., and Wang, X. S. (2019). Silver-induced [3+2] cycloaddition of isocyanides with acyl chlorides: Regioselective synthesis of 2,5-disubstituted oxazoles. ChemCatChem 11, 4272–4275. doi:10.1002/cctc.201900965
Liu, L. Y., Yan, Y. Z., Bao, Y. J., and Wang, Z. Y. (2015). Efficient synthesis of 2-arylquinazolines via copper-catalyzed dual oxidative benzylic CH aminations of methylarenes. Chin. Chem. Lett. 26, 1216–1220. doi:10.1016/j.cclet.2015.07.008
Liu, Q., Zhao, Y., Fu, H., and Cheng, C. (2013). Copper-catalyzed sequential N-arylation and aerobic oxidation: Synthesis of quinazoline derivatives. Synlett 24, 2089–2094. doi:10.1055/s-0033-1339800
Liu, S., Wang, A. J., Li, M., Zhang, J., Yin, G. D., Shu, W. M., et al. (2022). Rh(III)-Catalyzed tandem reaction access to (Quinazolin-2-yl)methanone derivatives from 2,1-benzisoxazoles and α-azido ketones. J. Org. Chem. 87, 11253–11260. doi:10.1021/acs.joc.2c01214
Liu, W., and Ackermann, L. (2016). Manganese-catalyzed C–H activation. ACS Catal. 6, 3743–3752. doi:10.1021/acscatal.6b00993
Liu, Z., Wang, L., Fenga, M., Yi, Y., Zhang, W., Liu, W., et al. (2018). New acrylamide-substituted quinazoline derivatives with enhanced potency for the treatment of EGFR T790M-mutant non-small-cell lung cancers. Bioorg. Chem. 77, 593–599. doi:10.1016/j.bioorg.2018.01.035
Lu, J. M., Huo, Y. W., Qi, X., and Wu, X. F. (2021). Palladium-catalyzed carbonylative synthesis of quinazolines: Silane act as better nucleophile than amidine. Molec. Catal. 509, 111627–111629. doi:10.1016/j.mcat.2021.111627
Lu, L., Liu, X. Y., Shu, X. Z., Yang, K., Ji, K. G., and Liang, Y. M. (2009). Platinum-catalyzed cycloisomerization reaction of 1,6-enyne coupling with rearrangement of propargylic esters. J. Org. Chem. 74, 474–477. doi:10.1021/jo802043z
Luhning, L. H., Rosien, M., and Doye, S. (2017). Synlett titanium-catalyzed hydroaminoalkylation of vinylsilanes and a one-pot procedure for the synthesis of 1,4-benzoazasilines, 2489–2494.
Lukin, R. Y., Kuchkaev, A. M., Sukhov, A. V., Bekmukhamedov, G. E., and Yakhvarov, D. G. (2020). Platinum-catalyzed hydrosilylation in polymer chemistry. Polymers 12, 2174–2195. doi:10.3390/polym12102174
Lv, Y., Li, Y., Xiong, T., Pu, W., Zhang, H., Sun, K., et al. (2013). Copper-catalyzed annulation of amidines for quinazoline synthesis. Chem. Commun. 49, 6439–6441. doi:10.1039/c3cc43129k
Ma, Z., Liu, Z., Jiang, T., Zhang, T., Zhang, H., Du, L., et al. (2016). Discovery of fluorescence polarization probe for the ELISA-based antagonist screening of α1-adrenergic receptors. ACS Med. Chem. Lett. 7, 967–971. doi:10.1021/acsmedchemlett.6b00048
Machara, A., Lux, V., Kozisek, M., Grantz-Saskova, K., Stepanek, O., Kotora, M., et al. (2016). Specific inhibitors of HIV capsid assembly binding to the C-terminal domain of the capsid protein: Evaluation of 2-arylquinazolines as potential antiviral compounds. J. Med. Chem. 59, 545–558. doi:10.1021/acs.jmedchem.5b01089
Maeda, S., Komagawa, S., Uchiyama, M., and Morokuma, K. (2011). Finding reaction pathways for multicomponent reactions: The passerini reaction is a four-component reaction. Angew. Chem. Int. Ed. 50, 644–649. doi:10.1002/anie.201005336
Mako, T. L., and Bayers, J. A. (2016). Recent advances in iron-catalysed cross coupling reactions and their mechanistic underpinning. Inorg. Chem. Front. 3, 766–790. doi:10.1039/c5qi00295h
Mazloumi, M., and Shirini, F. (2020). Poly. Aromatic compds. Synthesis of quinolines, quinazolines and spiro-quinazolines using nanoporous TiO2 containing an ionic liquid bridge as an efficient and reusable catalyst 2020, 2087–2106.
McMurray, L., O’Hara, F., and Gaunt, M. J. (2011). Recent developments in natural product synthesis using metal-catalysed C–H bond functionalisation. Chem. Soc. Rev. 40, 1885. doi:10.1039/c1cs15013h
McMurry, J. E., Fleming, M. P., Kees, K. L., and Krepski, L. R. (1978). Titanium-induced reductive coupling of carbonyls to olefins. J. Org. Chem. 43, 3255–3266. doi:10.1021/jo00411a002
Michael, J. P. (2008). Quinoline, quinazoline and acridonealkaloids. Nat. Prod. Rep. 25, 166–187. doi:10.1039/b612168n
Miura, T., Biyajima, T., Fujii, T., and Murakami, M. J. (2012). Synthesis of α-amino ketones from terminal alkynes via rhodium-catalyzed denitrogenative hydration of N-Sulfonyl-1,2,3-triazoles. J. Am. Chem. Soc. 134, 194–196. doi:10.1021/ja2104203
Mondal, A., Sahoo, M. K., Subaramanian, M., and Balaraman, E. (2020). Manganese(I)-Catalyzed sustainable synthesis of quinoxaline and quinazoline derivatives with the liberation of dihydrogen. J. Org. Chem. 85, 7181–7191. doi:10.1021/acs.joc.0c00561
Muto, K., Yamaguchi, J., Musaev, D. G., and Itami, K. (2015). Decarbonylative organoboron cross-coupling of esters by nickel catalysis. Nat. Commun. 6, 7508–7515. doi:10.1038/ncomms8508
Nijamudheen, A., and Datta, A. (2019). Gold-catalyzed cross-coupling reactions: An overview of design strategies, mechanistic studies, and applications. Chem. Eur. J. 26, 1442–1487. doi:10.1002/chem.201903377
Nugent, W. A., and RajanBabu, T. V. (1988). Transition-metal-centered radicals in organic synthesis. Titanium(III)-induced cyclization of epoxy olefins. J. Am. Chem. Soc. 110, 8561–8562. doi:10.1021/ja00233a051
Ohta, Y., Tokimizu, Y., Oishi, S., Fujii, N., and Ohno, H. (2010). Direct synthesis of quinazolines through copper-catalyzed reaction of aniline-derived benzamidines. Org. Lett. 12, 3963–3965. doi:10.1021/ol1016756
Omar, M. A., Conrad, J., and Beifuss, U. (2014). Copper-catalyzed domino reaction between 1-(2-halophenyl)methanamines and amidines or imidates for the synthesis of 2-substituted quinazolines. Tetrahedron 70, 3061–3072. doi:10.1016/j.tet.2014.02.066
Pal, P., Pahari, S. K., Giri, A. K., Pal, S., Bajaj, H. C., and Panda, A. B. (2013). A hierarchically order porous lotus shaped nano-structured MnO2 through MnCO3: Chelate mediated growth and shape dependent improved catalytic activity. J. Mater. Chem. 1, 10251–10258.
Panja, S. K., and Saha, S. (2013). RSC Adv. Recyclable, magnetic ionic liquid bmim[FeCl4]-catalyzed, multicomponent, solvent-free. green synthesis quinazolines 3, 14495–14500.
Parua, S., Sikari, R., Sinha, S., Chakraborty, G., Mondal, R., and Paul, N. D. (2018). Accessing polysubstituted quinazolines via nickel catalyzed acceptorless dehydrogenative coupling. J. Org. Chem. 83, 11154–11166. doi:10.1021/acs.joc.8b01479
Pflasterer, D., and Hashmi, A. S. K. (2016). Gold catalysis in total synthesis – recent achievements. Chem. Soc. Rev. 45, 1331–1367. doi:10.1039/c5cs00721f
Prakash, R., Bora, B. R., Boruah, R. C., and Gogoi, S. (2018). Ru(II)-Catalyzed C–H activation and annulation reaction via carbon–carbon triple bond cleavage. Org. Lett. 20, 2297–2300. doi:10.1021/acs.orglett.8b00643
Rahman, M. U., Rathore, A., Siddiqui, A. A., Parveen, G., and Yar, M. S. (2014). Synthesis and characterization of quinazoline derivatives: Search for hybrid molecule as diuretic and antihypertensive agents. J. Enzyme Inhib. Med. Chem. 29, 733–743. doi:10.3109/14756366.2013.845820
Raut, A. B., Tiwari, A. R., and Bhanage, B. M. (2017). Ultrasound-assisted preparation of copper(I) oxide nanocubes: High catalytic activity in the synthesis of quinazolines. ChemCatChem 9, 1292–1297. doi:10.1002/cctc.201601330
Reddy, A. S., and Swamy, K. C. K. (2017). Ethanol as a hydrogenating agent: Palladium-catalyzed stereoselective hydrogenation of ynamides to give enamides. Angew. Chem. Int. Ed. 56, 6984–6988. doi:10.1002/anie.201702277
Riadi, Y., Kadhim, M. M., Shoja, S. J., Ali, M. H., Mustafa, Y. F., and Sajjadi, A. (2022). Copper (II) complex supported on the surface of magnetic nanoparticles modified with S-benzylisothiourea (Fe3O4@SiO2-SMTU-Cu): A new and efficient nanomagnetic catalyst for the synthesis of quinazolines and amides. A new Effic. nanomagnetic Catal. synthesis quinazolines amides 52, 875–887. doi:10.1080/00397911.2022.2056849
Rosini, M., Antonello, A., Cavalli, A., Bolognesi, M. L., Minarini, A., Marucci, G., et al. (2003). Prazosin-related compounds. Effect of transforming the piperazinylquinazoline moiety into an aminomethyltetrahydroacridine system on the affinity for α1-adrenoreceptors. J. Med. Chem. 46, 4895–4903. doi:10.1021/jm030952q
Ryu, T., Baek, Y., and Lee, P. H. (2015). Synthesis of pyrazines from rhodium-catalyzed reaction of 2H-azirines with N-sulfonyl 1,2,3-triazoles. J. Org. Chem. 80, 2376–2383. doi:10.1021/acs.joc.5b00036
Sasmal, S., Balaji, G., Reddy, H. R. K., Balasubrahmanyam, D., Srinivas, G., Kyasa, S. K., et al. (2012). Design and optimization of quinazoline derivatives as melanin concentrating hormone receptor 1 (MCHR1) antagonists. Bioorg. Med. Chem. Lett. 22, 3157–3162. doi:10.1016/j.bmcl.2012.03.050
Sastry, K. N. V., Prasad, B., Nagaraju, B., Reddy, V. G., Alarifi, A., Babu, B. N., et al. (2017). Copper-Catalysed tandem synthesis of substituted quinazolines from phenacyl azides and O-carbonyl anilines. ChemistrySelect 2, 5378–5383. doi:10.1002/slct.201700889
Satish, G., Polu, A., Kota, L., and Ilangovan, A. (2019). Copper-catalyzed oxidative amination of methanol to access quinazolines. Org. Biomol. Chem. 17, 4774–4782. doi:10.1039/c9ob00392d
Sayed, S. E., Bordet, A., Weidenthaler, C., Hetaba, W., Luska, K. L., and Leitner, W. (2020). Selective hydrogenation of benzofurans using ruthenium nanoparticles in Lewis acid-modified ruthenium-supported ionic liquid phases. ACS Catal. 10, 2124–2130. doi:10.1021/acscatal.9b05124
Schiwek, C. H., Jandl, C., and Bach, T. (2020). Diastereoselective rhodium-catalyzed hydrogenation of 2-oxindoles and 3,4-dihydroquinolones. Org. Lett. 22, 9468–9472. doi:10.1021/acs.orglett.0c03427
Shagufta, I. A. (2017). An insight into the therapeutic potential of quinazoline derivatives as anticancer agents. MedChemComm 8, 871–885. doi:10.1039/c7md00097a
Shiju, N. R., and Guliants, V. V. (2009). Recent developments in catalysis using nanostructured materials. Appl. Catal. 356, 1–17. doi:10.1016/j.apcata.2008.11.034
Shui, H., Zhong, Y., Ouyang, L., Luo, N., and Luo, R. (2022). Iridium-catalyzed acceptorless dehydrogenative coupling of 2-aminoarylmethanols with amides or nitriles to synthesize quinazolines. Synthesis 54, 2876–2884. doi:10.1055/a-1755-4700
Sikari, R., Sinha, S., Chakraborty, G., Das, S., Leest, N. P. V., and Paul, N. D. (2019). C−N cross-coupling reactions under mild conditions using singlet di-radical nickel(II)-Complexes as catalyst: N-Arylation and quinazoline synthesis. N-Arylation Quinazoline Synthesis 361, 4342–4353. doi:10.1002/adsc.201900545
Sivakumar, G., Subaramanian, M., and Balaraman, E. (2022). Single-molecular Mn(I)-Complex-Catalyzed tandem double dehydrogenation cross-coupling of (Amino)Alcohols under solventless conditions with the liberation of H2 and H2O. ACS Sustain. Chem. Eng. 10, 7362–7373. doi:10.1021/acssuschemeng.2c01222
Sordella, R., Bell, D. W., Harber, D. A., and Settleman, J. (2004). Gefitinib-sensitizing EGFR mutations in lung cancer activate anti-apoptotic pathways. Science 305, 1163–1167. doi:10.1126/science.1101637
Srivastava, S., and Srivastava, S. (2015). Biological activity of quinazoline: A review. Int. J. Pharma Sci. Res. 6, 1206–1213.
Su, X., Chen, C., Wang, Y., Chen, J., Lou, Z., and Li, M. (2013). One-pot synthesis of quinazoline derivatives via [2+2+2] cascade annulation of diaryliodonium salts and two nitriles. Chem. Commun. 49, 6752–6754. doi:10.1039/c3cc43216e
Sun, Y., Sun, H., Wang, Y., and Xie, F. (2020). Cu/Ag-Catalyzed reaction of azirines with anthranils: Synthesis of (Quinazolin-2-yl)methanone derivatives. Org. Lett. 22, 6756–6759. doi:10.1021/acs.orglett.0c02222
Szollosi, G., Kovacs, L., and Makra, Z. (2015). Three consecutive steps over the chirally modified Pt surface: Asymmetric catalytic cascade reaction of 2-nitrophenylpyruvates. Catal. Sci. Technol. 5, 697–704. doi:10.1039/c4cy00883a
Tan, Z., Fu, Z., Yang, J., Wu, Y., Cao, L., Jiang, H., et al. (2020). iScience hydrogen transfer-mediated multicomponent reaction for direct synthesis of quinazolines by a naphthyridine-based. Iridium Catal. 23, 101003–101139.
Tang, L., Wang, P., Fan, Y., Yang, X., Wan, C., and Zha, Z. (2016). Heterogeneous palladium-catalyzed hydrogen-transfer cyclization of nitroacetophenones with benzylamines: Access to C−N bonds. Access C−N Bonds 8, 3565–3569. doi:10.1002/cctc.201601060
Tang, L., Yang, Y., Wen, L., Zhang, S., Zha, Z., and Wang, Z. (2015). Supported gold-catalyzed and ammonia-promoted selective synthesis of quinazolines in aqueous media. Org. Chem. Front. 2, 114–118. doi:10.1039/c4qo00278d
Tian, K. M., Li, J. J., and Xu, S. W. (2019). Rutaecarpine: A promising cardiovascular protective alkaloid from evodia rutaecarpa (Wu zhu Yu). Pharmacol. Res. 141, 541–550. doi:10.1016/j.phrs.2018.12.019
Truong, T., Hoang, T. M., Nguyen, C. K., Huynh, Q. T. N., and Phan, N. T. S. (2015). Expanding applications of zeolite imidazolate frameworks in catalysis: Synthesis of quinazolines using ZIF-67 as an efficient heterogeneous catalyst. RSC Adv. 5, 24769–24776. doi:10.1039/c4ra16168h
Truong, V. L., and Morrow, M. (2010). Mild and efficient ligand-free copper-catalyzed condensation for the synthesis of quinazolines. Tetrahedron Lett. 51, 758–760. doi:10.1016/j.tetlet.2009.11.133
Van Horn, K. S., Zhu, X., Pandharkar, T., Yang, S., Vesely, B., Vanaerschot, M., et al. (2014). Antileishmanial activity of a series of N2,N4-disubstituted quinazoline-2,4-diamines. J. Med. Chem. 57, 5141–5156. doi:10.1021/jm5000408
Viereck, P., Krautwald, S., Pabst, T. P., and Chirik, P. J. (2020). A boron activating effect enables cobalt-catalyzed asymmetric hydrogenation of sterically hindered alkenes. J. Am. Chem. Soc. 142, 3923–3930. doi:10.1021/jacs.9b12214
Waiba, S., and Maji, B. (2020). Manganese catalyzed acceptorless dehydrogenative coupling reactions. ChemCatChem 12, 1891–1902. doi:10.1002/cctc.201902180
Wan, X. M., Liu, Z. L., Liu, W. Q., N Cao, X., Zhu, X., Zhao, X. M., et al. (2019). NNN pincer Ru(II)-catalyzed dehydrogenative coupling of 2-aminoarylmethanols with nitriles for the construction of quinazolines. Tetrahedron 75, 2697–2705. doi:10.1016/j.tet.2019.03.046
Wan, Z., Hu, D., Li, P., Xie, D., and Gan, X. (2015). Synthesis, antiviral bioactivity of novel 4-thioquinazoline derivatives containing chalcone moiety. Molecules 20, 11861–11874. doi:10.3390/molecules200711861
Wang, C., Li, S., Liu, H., Jiang, Y., and Fu, H. (2010). Copper-catalyzed synthesis of quinazoline derivatives via ullmann-type coupling and aerobic oxidation. J. Org. Chem. 75, 7936–7938. doi:10.1021/jo101685d
Wang, C., Rui, X., Si, D., Dai, R., Zhu, Y., Wen, H., et al. (2021). Copper-catalyzed three-component cascade reaction of benzaldehyde with benzylamine and hydroxylamine or aniline: Synthesis of 1,2,4-oxadiazoles and quinazolines. Adv. Synth.Catal. 363, 2825–2833. doi:10.1002/adsc.202001535
Wang, D., and Gao, F. (2013). Quinazoline derivatives: Synthesis and bioactivities. Chem. Cent. J. 7, 95–110. doi:10.1186/1752-153X-7-95
Wang, F., Wang, H., Wang, Q., Yu, S., and Li, X. (2016). Co(III)-Catalyzed synthesis of quinazolines via C–H activation of N-sulfinylimines and benzimidates. Org. Lett. 18, 1306–1309. doi:10.1021/acs.orglett.6b00227
Wang, H., Chen, H., Chen, Y., and Deng, G. J. (2014). Palladium-catalyzed one pot 2-arylquinazoline formation via hydrogen-transfer strategy. Org. Biomol. 12, 7792–7799. doi:10.1039/c4ob01296h
Wang, H., Park, Y., Bai, Z., Chang, S., He, G., and Chen, G. (2019). Iridium-catalyzed enantioselective C(sp3)–H amidation controlled by attractive noncovalent interactions. J. Am. Chem. Soc. 141, 7194–7201. doi:10.1021/jacs.9b02811
Wang, H. W., Lu, Y., Zhang, B., He, J., Xu, H. J., Kang, Y. S., et al. (2017). Ligand-promoted rhodium(III)-Catalyzed ortho C−H amination with free amines. Angew. Chem. Int. Ed. 56, 7449–7453. doi:10.1002/anie.201703300
Wang, J., Zha, S., Chen, K., Zhang, F., Song, C., and Zhu, J. (2016). Quinazoline synthesis via Rh(III)-Catalyzed intermolecular C–H functionalization of benzimidates with dioxazolones. Org. Lett. 18, 2062–2065. doi:10.1021/acs.orglett.6b00691
Wang, X., He, D., Huang, Y., Fan, Q., Wu, W., and Jiang, H. (2018). Copper-catalyzed synthesis of substituted quinazolines from benzonitriles and 2-ethynylanilines via carbon–carbon bond cleavage using molecular oxygen. J. Org. Chem. 83, 5458–5466. doi:10.1021/acs.joc.8b00378
Wang, X., and Jiao, N. (2016). Rh- and Cu-cocatalyzed aerobic oxidative approach to quinazolines via [4 + 2] C–H annulation with alkyl azides. Org. Lett. 18, 2150–2153. doi:10.1021/acs.orglett.6b00774
Watile, R. A., Bunrit, A., Margalef, J., Akkarasamiyo, S., Ayub, R., Lagerspets, E., et al. (2019). Intramolecular substitutions of secondary and tertiary alcohols with chirality transfer by an iron(III) catalyst. Nat. Commun. 10, 3826–3829. doi:10.1038/s41467-019-11838-x
Wight, A. P., and Davis, M. E. (2002). Chemical reviews design and preparation of Organic−Inorganic hybrid catalysts, 3589–3614.
Wilt, T. J., Howe, R. W., Rutks, I. R., and MacDonald, R. (2002). Terazosin for benign prostatic hyperplasia. Cochrane Database Syst. Rev. 4, CD003851. doi:10.1002/14651858.CD003851
Xin, X. Y., Wang, H. L., Li, X. C., Wang, D. P., and Wan, B. S. (2015). Base-catalyzed selective synthesis of 2-Azabicyclo[3.2.0]hept-2-enes and sulfonyl vinyl-substituted pyrroles from 3-Aza-1,5-enynes. Org. Lett. 17, 3944–3947. doi:10.1021/acs.orglett.5b01474
Xing, H., Chen, J., Shi, Y., Huang, T., Hai, L., and Wu, Y. (2020). Synthesis of 4-ethenyl quinazolines via rhodium(iii)-catalyzed [5 + 1] annulation reaction of N-arylamidines with cyclopropenones. Org. Chem. Front. 7, 672–677. doi:10.1039/c9qo01372e
Xu, C., Jia, F. C., Zhou, Z. W., Zheng, S. J., Li, H., and Wu, A. X. (2016). Copper-catalyzed multicomponent domino reaction of 2-bromoaldehydes, benzylamines, and sodium azide for the assembly of quinazoline derivatives. J. Org. Chem. 81, 3000–3006. doi:10.1021/acs.joc.5b02843
Xu, F., Kang, W. F., Wang, Y., Liu, C. S., Tian, J. Y., Zhao, R. R., et al. (2018). Rhodium(III)-Catalyzed cascade [5 + 1] annulation/5-exo-cyclization initiated by C–H activation: 1,6-Diynes as one-carbon reaction partners. Org. Lett. 20, 3245–3249. doi:10.1021/acs.orglett.8b01105
Xu, H. B., Zhu, Y. Y., Yang, J. H., Chai, X. Y., and Dong, L. (2020). RhIII-Catalyzed one-pot cascade synthesis of quinazolines with N-alkoxyamide as an amidating reagent. Org. Chem. Front. 7, 1230–1234. doi:10.1039/d0qo00084a
Xu, L., Li, H., Liao, Z., Lou, K., Xie, H., Li, H., et al. (2015). Divergent synthesis of imidazoles and quinazolines via Pd(OAc)2-Catalyzed annulation of N-allylamidines. Annu. N-Allylamidines 17, 3434–3437. doi:10.1021/acs.orglett.5b01435
Xu, Y., Young, M. C., and Dong, G. (2017). Catalytic coupling between unactivated aliphatic C–H bonds and alkynes via a metal–hydride pathway. J. Am. Chem. Soc. 139, 5716–5719. doi:10.1021/jacs.7b02020
Wang, X., Lerchen, A., and Glorius, F. (2016). A comparative investigation: Group 9 Cp*M(III)-Catalyzed formal [4 + 2] cycloaddition as an atom-economic approach to quinazolines. Org. Lett. 18, 2090–2093. doi:10.1021/acs.orglett.6b00716
Yang, J., Sekiguchi, Y., and Yoshikai, N. (2019). Cobalt-catalyzed enantioselective and chemodivergent addition of cyclopropanols to oxabicyclic alkenes. ACS Catal. 9, 5638–5644. doi:10.1021/acscatal.9b00655
Yang, K., Niu, B., Ma, Z., Wang, H., Lawrence, B., and Ge, H. (2019). Silver-promoted site-selective intramolecular cyclization of 2-methylthiobenzamide through α-C(sp3)–H functionalization. J. Org. Chem. 84, 14045–14052. doi:10.1021/acs.joc.9b02202
Yang, L., Luo, H., Sun, Y., Shi, Z., Ni, K., Li, F., et al. (2017). A highly efficient copper-catalyzed three-component synthesis of 4-aminoquinazolines. Aminoquinazolines 49, 2535–2543. doi:10.1055/s-0036-1588727
Yang, X., and Wang, C. (2018). Manganese-catalyzed hydrosilylation reactions. Chem.-Asian J. 13, 2307–2315. doi:10.1002/asia.201800618
Yang, Y. F., Houk, K. N., and Wu, Y. D. (2016). Computational exploration of RhIII/RhV and RhIII/RhI catalysis in rhodium(III)-Catalyzed C–H activation reactions of N-phenoxyacetamides with alkynes. J. Am. Chem. Soc. 138, 6861–6868. doi:10.1021/jacs.6b03424
Yao, N., Bi, X., Zhang, L., Tao, L., Zhao, P., Meng, X., et al. (2021). Mixed crystalline phases and catalytic performance of OMS-2 based nanocomposites for one-pot synthesis of quinazolines with O2 as an oxidant. Molec. Catal. 504, 111499–111508. doi:10.1016/j.mcat.2021.111499
Ye, B., and Cramer, N. (2015). Chiral cyclopentadienyls: Enabling ligands for asymmetric Rh(III)-Catalyzed C–H functionalizations. Acc. Chem. Res. 48, 1308–1318. doi:10.1021/acs.accounts.5b00092
Ye, C., You, J., Li, X. F., You, R., Weng, Y., Li, J., et al. (2010). Design, synthesis and anticoccidial activity of a series of 3-(2-(2-methoxyphenyl)-2-oxoethyl) quinazolinone derivatives. Pestic. Biochem. Physiol. 97, 194–198. doi:10.1016/j.pestbp.2010.02.001
Yu, C., Guo, X., Xi, Z., Muzzio, M., Yin, Z., Shen, B., et al. (2017). AgPd nanoparticles deposited on WO2.72 nanorods as an efficient catalyst for one-pot conversion of nitrophenol/nitroacetophenone into benzoxazole/quinazoline. J. Am. Chem. Soc. 139, 5712–5715. doi:10.1021/jacs.7b01983
Zhang, J., Yu, C., Wang, S., Wan, C., and Wang, Z. (2010). A novel and efficient methodology for the construction of quinazolines based on supported copper oxide nanoparticles. Chem. Commun. 46, 5244–5246. doi:10.1039/c002454f
Zhang, L., Zhao, Y., Wang, J., Yang, D., Zhao, C., Wang, C., et al. (2018). Design, synthesis and bioevaluation of 1,2,3,9-tetrahydropyrrolo[2,1-b]quinazoline-1-carboxylic acid derivatives as potent neuroprotective agents. Eur. J. Med. Chem. 151, 27–38. doi:10.1016/j.ejmech.2018.03.052
Zhang, S. Q., Cui, Y., Guo, B., Young, D. J., Xu, Z., and Li, H. X. (2021). Efficient synthesis of quinazolines by the iron-catalyzed acceptorless dehydrogenative coupling of (2-aminophenyl)methanols and benzamides. Tetrahedron 78, 131825–131832. doi:10.1016/j.tet.2020.131825
Zhang, W., Guo, F., Wang, F., Zhao, N., Liu, L., Li, J., et al. (2014). Synthesis of quinazolines via CuO nanoparticles catalyzed aerobic oxidative coupling of aromatic alcohols and amidines. Org. Biomol. Chem. 12, 5752–5756. doi:10.1039/c4ob00569d
Zhang, X., Durndell, L. J., Isaacs, M. A., Parlett, C. M. A., Lee, A. F., and Wilson, K. (2016). Platinum-catalyzed aqueous-phase hydrogenation of d-glucose to d-sorbitol. ACS Catal. 6, 7409–7417. doi:10.1021/acscatal.6b02369
Zhang, X., Xamena, F. X. L. i., Corma, A., and CatalGold, J. (2009). Gold(III) – metal organic framework bridges the gap between homogeneous and heterogeneous gold catalysts. J. Catal. 265, 155–160. doi:10.1016/j.jcat.2009.04.021
Zhang, Y., Shao, Y., Gong, J., Hu, K., Cheng, T., and Chen, J. (2018). Palladium-catalyzed tandem reaction of quinazolinone-based nitriles with arylboronic acids: Synthesis of 2-(4-Arylquinazolin-2-yl)anilines. Adv. Synth. Catal. 360, 3260–3265. doi:10.1002/adsc.201800615
Zhang, Z., Wang, F., Wang, M., Xu, S., Chen, H., Zhang, C., et al. (2014). tert-Butyl hydroperoxide (TBHP)-mediated oxidative self-coupling of amines to imines over a α-MnO2 catalyst. Green Chem. 16, 2523–2527. doi:10.1039/c3gc42312c
Zhang, Z., Wang, M., Zhang, C., Zhang, Z., Lu, J., and Wang, F. (2015). The cascade synthesis of quinazolinones and quinazolines using an α-MnO2 catalyst and tert-butyl hydroperoxide (TBHP) as an oxidant. Chem. Commun. 51, 9205–9207. doi:10.1039/c5cc02785c
Zhao, D., Shen, Q., Zhou, Y. R., and Li, J. X. (2013). KOtBu-mediated stereoselective addition of quinazolines to alkynes under mild conditions. Org. Biomol. 11, 5908–5912. doi:10.1039/c3ob41083h
Hao, Z., Zhou, X., Ma, Z., Zhang, C., Han, Z., Lin, J., et al. (2022). Dehydrogenative synthesis of quinolines and quinazolines via ligand-free cobalt-catalyzed cyclization of 2-aminoaryl alcohols with ketones or nitriles. J. Org. Chem. 87, 12596–12607. doi:10.1021/acs.joc.2c00734
Zhou, T., Ji, C. L., Hong, X., and Szostak, M. (2019). Palladium-catalyzed decarbonylative Suzuki–Miyaura cross-coupling of amides by carbon–nitrogen bond activation. Chem. Sci. 10, 9865–9871. doi:10.1039/c9sc03169c
Zhou, T., Li, B., and Wang, B. (2016). Rhodium-catalyzed C–H activation of 3-(indolin-1-yl)-3-oxopropanenitriles with diazo compounds and tandem cyclization leading to hydrogenated azepino[3,2,1-hi]indoles. Chem. Commun. 52, 14117–14120. doi:10.1039/c6cc07758g
Keywords: quinazoline, transition-metal, catalysis, synthesis, mechanism
Citation: Tamatam R, Kim S-H and Shin D (2023) Transition-metal-catalyzed synthesis of quinazolines: A review. Front. Chem. 11:1140562. doi: 10.3389/fchem.2023.1140562
Received: 09 January 2023; Accepted: 27 February 2023;
Published: 16 March 2023.
Edited by:
Virginia Spanò, University of Palermo, ItalyReviewed by:
Jun Xu, Hangzhou Normal University, ChinaVijay Kumar Siripuram, The Rockefeller University, United States
Copyright © 2023 Tamatam, Kim and Shin. This is an open-access article distributed under the terms of the Creative Commons Attribution License (CC BY). The use, distribution or reproduction in other forums is permitted, provided the original author(s) and the copyright owner(s) are credited and that the original publication in this journal is cited, in accordance with accepted academic practice. No use, distribution or reproduction is permitted which does not comply with these terms.
*Correspondence: Seok-Ho Kim, a3NoMzQxMEBrYW5nd29uLmFjLmty; Dongyun Shin, ZHlzaGluQGdhY2hvbi5hYy5rcg==