- 1Faculty of Natural Resource Sciences, University of Akureyri, Akureyri, Iceland
- 2BioPol ehf. Marine Biotechnology, Skagaströnd, Iceland
- 3Institute of Biological Sciences, University of Rostock, Rostock, Germany
- 4Plankton and Microbial Ecology, Leibniz-Institute of Freshwater Ecology and Inland Fisheries, Berlin, Germany
- 5Institute of Biochemistry and Biology, Potsdam University, Potsdam, Germany
- 6Department of Biochemistry and Molecular Biology, Faculty of Medicine, University of Iceland, Reykjavik, Iceland
- 7Institute of Coastal Environmental Chemistry, Helmholtz-Zentrum Hereon, Geesthacht, Germany
The cryosphere faces increasing threats from anthropogenic pollutants, including per- and polyfluoroalkyl substances (PFAS), a class of synthetic chemicals produced in significant quantities and released into the environment for over seven decades. PFAS are widely utilized for their water- and grease-resistant properties in numerous industrial, household, personal care, and medical products. Despite their widespread applications, all PFAS or their degradation and transformation products are environmentally persistent and pose health risks to humans. PFAS are detected ubiquitously, even in remote regions like the Arctic and Antarctica, and they bioaccumulate within polar trophic food chains. The primary transport and transmission mechanisms for PFAS involve atmospheric transport through volatile precursors, atmospheric oxidation, ocean currents, and the formation of sea spray aerosols. Additionally, contamination of surface snow, post-deposition processes in snow, and sediment interactions significantly contribute to PFAS transport. The physical and chemical properties, including density, melting points (Tm), boiling points (Tb), solubility, vapor pressure, electronegativity, low polarizability, chemical stability, and thermal stability, play key roles in determining their environmental fate and transformation. The toxicity of certain PFAS has raised concerns, prompting bans and efforts to develop safer alternatives. Despite increasing public awareness and regulations to limit the production of legacy PFAS, their long-term environmental impacts remain unclear. As global warming accelerates cryosphere shrinkage, which releases PFAS with meltwater, cold-adapted ecosystems and associated biota face unprecedented challenges and uncertainties, particularly regarding the accumulation of non-degradable materials. This situation underscores the urgent need to comprehensively understand the fate of PFAS and adopt effective management strategies for polar systems. This review summarizes current literature on the transport, distribution, and legacy of PFAS, along with their known ecological impacts, bioremediation potential, and other management options in the cryosphere.
1 The cryosphere
The cryosphere, derived from the Greek word “κρῧος” meaning icy cold, encompasses the planet’s frozen (solid) water systems found across marine and terrestrial environments. This includes snow, glaciers, lake and river ice, frozen ground and permafrost, the Antarctic and Greenland Ice Sheets, mountain ice caps, ice shelves and icebergs, and sea ice. These environments form a vital part of our planet´s ecosystems and influence Earth´s albedo, surface energy, and moisture fluxes, that in turn impact atmospheric and oceanic circulation patterns (Ásmundsdóttir and Scholz, 2021; NOAA, 2024). These intricate dynamics are increasingly offset by environmental pollutants, alongside the pervasive effects of climate change (Borgå et al., 2022; Bargagli and Rota, 2024; Zhou et al., 2024b).
In the Anthropocene epoch, the Earth’s systems have been significantly impacted by rapid industrialization, population growth, deforestation, pollution, and climate change (Zalasiewicz et al., 2019). Global warming, ocean acidification, melting glaciers, and thawing permafrost disrupt cryosphere ecosystems (Sonne et al., 2023; Bargagli and Rota, 2024). Compounding this challenge is environmental pollution that disrupts these fragile systems by altering the long-range transport, remobilization, bioavailability, and fate of persistent contaminants, as this frozen region acts as a storage of various pollutants. When the different forms of the cryosphere melt due to warming, the trapped contaminants are released into the environment and become bioavailable for organisms (Codling et al., 2014; Chen et al., 2019; McGovern et al., 2022; Zhou et al., 2024a). These chemical changes could threaten the survival of cold-adapted organisms and compromise their vital biogeochemical functions in polar ecosystems (Bargagli and Rota, 2024). Per-and polyfluoroalkyl substances (PFAS) are a group of emerging contaminants that raise significant concerns among scientists due to their widespread presence. Their detection in remote regions, from Mount Everest to the Arctic and Antarctic ice cores, demonstrates their long-range transport (Miner et al., 2021; Shan et al., 2021; Ahrens et al., 2023). As such, the integrity of these cold ecosystems is at considerable risk, emphasizing the urgent need for comprehensive measures to mitigate their impacts and protect the integrity of these vulnerable environments.
2 PFAS in the cryosphere: chemical nature, transformation, transport, distribution and occurrence
2.1 PFAS chemical nature and significance
Production of perfluorocarbons can be traced back to the late 19th century, with the synthesis of carbon tetrafluoride, recognized as the simplest perfluorocarbon, being produced in 1886 (Banks and Tatlow, 1986; Okazoe, 2009; Gaines, 2023). Synthesis of PFAS with various functional groups started in the mid-20th century, wherein the application of PFAS was applied as fluoropolymer coatings and materials designed to withstand heat, repel oil, resist stains, and provide protection against grease and water (Meegoda et al., 2020; Hamid et al., 2024). DuPont introduced the first commercial fluoropolymer, polytetrafluoroethylene (PTFE), branded as ‘Teflon,’ in 1948 (Okazoe, 2009). Shortly after World War II, the Minnesota Mining and Manufacturing Company (3M) began using electrochemical fluorination, leading to the commercial production of fluorocarbons in 1951. This expansion included fluorocarbon derivatives with functional groups like perfluoroethers, perfluoroacyl fluorides, perfluoroalkanesulfonyl fluorides, and perfluorinated amines. These derivatives have played a vital role for corporations, including DuPont, 3M, and W. L. Gore and Associates, which still use them to produce well-known products such as Teflon and Gore-Tex. Subsequently, during the 1960s, DuPont advanced partially-fluorinated polymers, including poly (vinylidene fluoride) (PVDF) and its modified form, polyvinyl fluoride (PVF) (Okazoe, 2009).
The commercial production of PFAS began in the 1970s with the advent of telomerization, marking the inception of these widely used anthropogenic chemicals (Okazoe, 2009). Due to their persistence, PFAS are often described as “forever chemicals” (Miner et al., 2021; Renfrew and Pearson, 2021; Manojkumar et al., 2023). They are a group of synthetic organofluorine compounds with a fluorocarbon backbone attached to a functional group (Figure 1). PFAS are defined by the presence of at least one fully fluorinated methyl (−CF3) or methylene (−CF2) group, with no hydrogen, chlorine, bromine, or iodine atoms attached to these carbon atoms (Wang Z. et al., 2021).
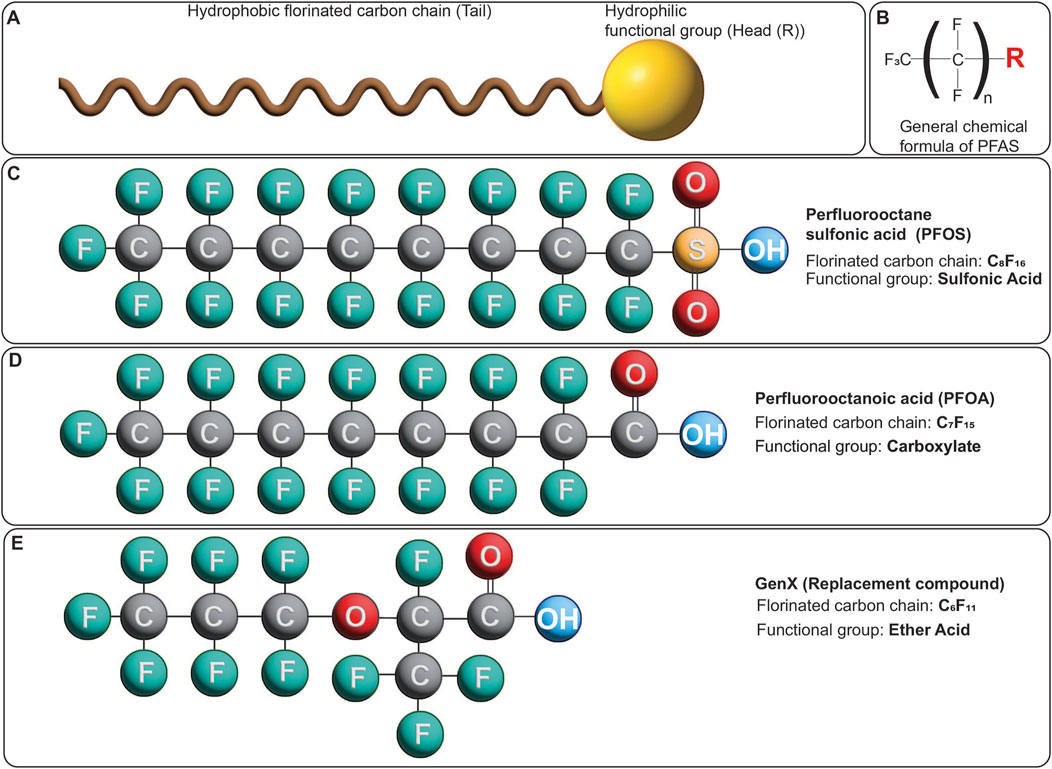
Figure 1. (A) Diagram representing the dual chemical nature of PFAS, showing the hydrophilic functional “head” group and the hydrophobic fluorinated carbon chain “tail.” (B) The basic framework of PFAS is where Cn F2n+1 denotes the perfluoroalkyl chain length, and “R” represents the functional group. (C) Shown are the example examples of general chemical structures of a fully fluorinated compound and the perfluoroalkane sulfonic acid (PFSA) with the functional group of (SO3−) (e.g., PFOS), and (D) is the illustration of the chemical structure of a fully fluorinated perfluoroalkyl carboxylic acids (PFCAs) with a functional group of (-COOH) (e.g., PFOA), and (E) represents an example of a short chain replacement compound hexafluoropropylene oxide dimer acid (GenX), often used as an alternative to long-chain PFOS and PFOA.
Polyfluoroalkyl substances are composed of a fluorinated aliphatic backbone, and compared to their hydrocarbon analogs, several hydrogen atoms are replaced by fluorine atoms (Buck et al., 2011). The corresponding perfluoroalkyl substances maintain the same structural configuration, with the distinction being that the chain is completely saturated with fluorine atoms, resulting in a more compact and electronegative demeanor (Buck et al., 2011).
PFAS are amphipathic and, as such, have emulsifying properties, making them excellent surface-active compounds as they interact with water and nonpolar substances (Figure 1). Owing to these attributes, PFAS are extensively utilized in various household products and industrial applications (Gaines, 2023), including waterproof clothing, carpets, furniture, paper, food packaging, heat-resistant non-stick cooking surfaces, and electrical wire insulation. Due to their broad applications, PFAS-based products enter the environment through multiple pathways, such as waste and rainwater, atmospheric deposition, water currents, etc., (Jansson, 2019; Ahrens et al., 2023). They spread globally, even reaching the most remote regions like the Arctic and Antarctica. PFAS have received significant attention due to their ability to travel long distances and documented bioaccumulation from the base of the food chain to apex predators, including polar bears (Khan et al., 2023; Figures 2A–D).
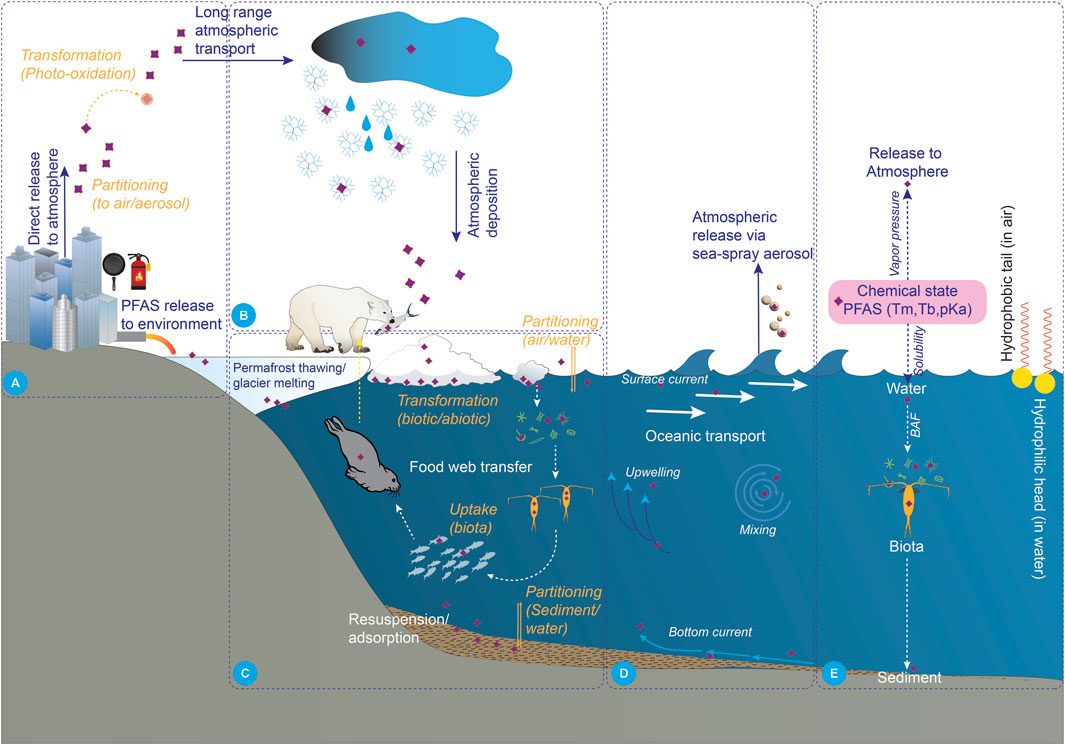
Figure 2. Schematic illustration of compound environmental processes influencing the PFAS transformation, fate, transport, and distribution of PFAS in the cryosphere. In the cryosphere, organisms are exposed to PFAS through contaminated water (sea, ice, snow, or glacier meltwater), sediment, and prey, leading to their transfer and magnification through the polar food web. BAF- bioaccumulation factor, the accumulation of PFAS in organisms from both seawater and their diet. (A) various sources of PFAS entering the environment., (B) Atmospheric transport of PFAS through snow, air, and rain., (C) potential entry points of PFAS to the food chain and biomagnification., (D) Oceanic transport routes of PFAS., and (E) PFAS chemical states influenced by factors, i.e., Tm- melting point, Tb- boiling point, pKa- acid dissociation constant.
2.2 PFAS phase behavior and transformation
The unique physicochemical properties influence the PFAS’ environmental fate and transformation. Key factors such as density, melting points (Tm), boiling points (Tb), solubility, vapor pressure, electronegativity, low polarizability, chemical stability, and thermal stability dictate their mobility and persistence (Figure 2E; Interstate Technology and Regulatory Council, 2023). Additionally, PFAS can partition into mobile colloids, facilitating their transport across different environmental matrices, a phenomenon relevant in cryosphere environments undergoing freeze-thaw cycles (Garnett et al., 2019; Garnett et al. 2021b; Sherman-Bertinetti et al., 2024).
PFAS mobility can be affected by salinity, pH, organic carbon content, and the presence of polyvalent cations (Nguyen et al., 2020). Cold environments often have unique geochemical conditions, such as acidic soils, permafrost, and glacial sediments, further impacting PFAS behavior. PFAS tend to associate the air-water interface with organic matter hydrophobically and form an electrostatic connection with charged surfaces (Interstate Technology and Regulatory Council, 2023). This is relevant in cold environments where organic matter is preserved in permafrost, thereby PFAS potentially affecting its mobility (Figure 2E).
PFAS density influences their behavior as dense, non-aqueous phase liquids or remain dissolved in water (Figures 2E, 3A). High-density PFAS, like 4:2 Fluorotelomer alcohol (FTOH) (1.59 g/cm3), can sink downward through the water column, potentially accumulating in deep-sea sediments. Volatile precursors undergo oxidation, converting into persistent perfluorooctanoic acid (PFOA) and perfluorooctanesulfonic acid (PFOS), leading to secondary contamination (Figures 3B–D; Ellis et al., 2004; Interstate Technology and Regulatory Council, 2023). Their higher vapor pressure allows them to exist in the gases, increasing their potential for long-range atmospheric transport (Hartz et al., 2023). The breakdown of 8:2 FTOH in the atmosphere has been linked to PFOA in Arctic summers due to increased photochemical activity (Van de Vijver et al., 2003). This illustrates the role of atmospheric degradation of FTOH into persistent PFCAs, including PFOA and PFNA (Figure 3B).
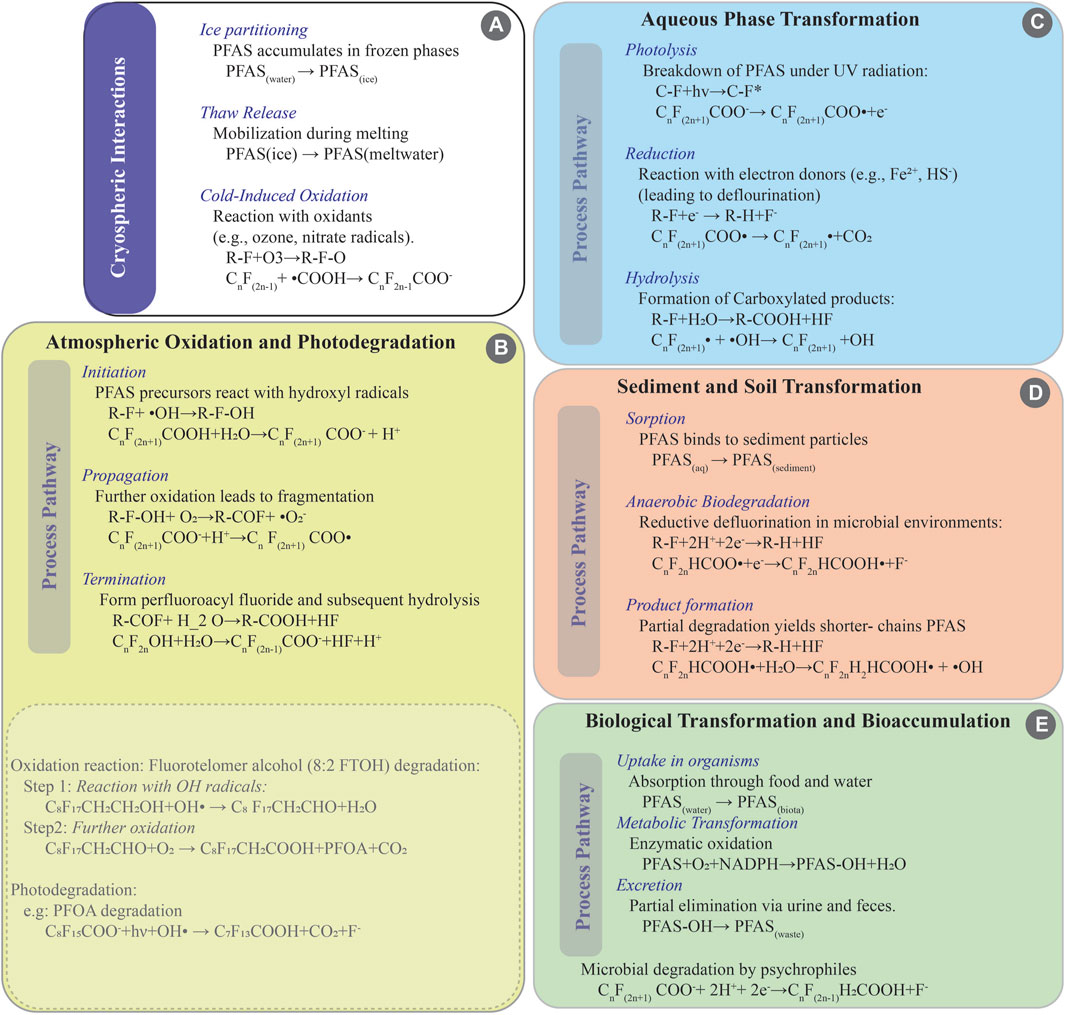
Figure 3. Possible transformation and degradation pathways of PFAS in cold environments. The diagram outlines key equations and processes affecting PFAS, i.e., fate and mobilization (A), atmospheric oxidation and photodegradation (B), aqueous phase transformation (C), sediment and soil transformation (D), and biological transformation and bioaccumulation (E). These processes collectively determine PFAS degradation/transformation, distribution, persistence, and potential environmental impacts of PFAS in the cryosphere.
Sea ice formation concentrates long-chain (C8-C12) PFAS in surface layers, enriching them up to threefold compared to short-chain (C4-C7) and sodium chloride (NaCl). As seawater freezes, expelled salts create brine channels that concentrate with PFAS. This partitioning process plays a key role in PFAS accumulation (Garnett et al., 2021a).) While some PFAS can form floating separate-phase layers, this behavior is primarily due to their surface activity rather than density alone (Figure 3A). This can enhance PFAS deposition in polar snowpacks and ice layers (Hartz et al., 2024). Factors like melting ice and snow, temperature changes, and sunlight durations also significantly affect the PFAS incorporation into air, water, and sediment (MacInnis et al., 2019b). For hexafluoropropylene oxide dimer acid (HFPO-DA: GenX), deposition in surface snow was notably higher during periods of continuous daylight, suggesting that sunlight-driven atmospheric degradation processes play a significant role in their formation and deposition (Hartz et al., 2024).
Cold condensation effects allow PFAS with low boiling points to volatilize and undergo long-range atmospheric transport before condensing in colder regions, known as global distillation. This is evidenced with the presence of short-chain PFAS and volatile precursors like FTOH in Arctic and Antarctic environments (Garnett et al., 2022). Moreover, PFAS with high melting points for long-chain PFAS, such as perfluortetradecanoic acid (PFTeDA), melting at 130°C–135°C, and chlorinated polyfluorinatedether sulfonic acid (Cl-PFESA), tend to sorb onto ice and snow, delaying the release until thawing occurs. This results in seasonal (summer) pulses of PFAS contamination into Arctic waters (Garnett et al., 2021b).
Acid dissociation constants (pKa) influence PFAS’sPFAS solubility and bioavailability (Interstate Technology and Regulatory Council, 2023). Low pKa PFAS, such as perfluorobutanoic acid (PFBA), perfluorohexanoic acid (PFHxA), and PFTeDA, exist almost entirely in their ionic form, increasing their mobility in Arctic waters and, leading to uptake by marine organisms (Joerss et al., 2020). PFBA (Tm is −17°C) has been detected at high concentrations (53% of total PFAS measured) in Svalbard meltwater, indicating a potential risk for polar biota (Ahrens et al., 2023). Neutral or weakly acidic PFAS such as polyfluorinated alkyl phosphoric acid esters (PAP) and FTOH cross the cell membranes before undergoing transformation and bioaccumulation (Wang et al., 2015b; Xie et al., 2015). They also partition into organic matter and lipid-rich marine organisms, thereby increasing long-term exposure (Gebbink et al., 2016; Xie and Kallenborn, 2023; Feng et al., 2024).
2.3 PFAS transport and distribution
PFAS concentrations vary geographically and are influenced by human population density and proximity to urbanized regions and landfills (Wee and Aris, 2023). These spatial differences suggest that PFAS distribution results from biological and geographical factors, as the latter alone do not fully account for concentration variations.
Their environmental fate, such as bioavailability, potential entry into the marine food web (Figure 2), and broader dispersal, is shaped by Arctic ocean surface conditions, air and water circulation patterns, and temperature-dependent physical-chemical characteristics like vapor pressure and partitioning coefficients (Lamon et al., 2009). While horizontal transport via surface currents is the primary mechanism for most PFAS, certain compounds- such as perfluorodecanoic acid (PFDA) and PFOS precursors, exhibit notable vertical fluxes through sediment resuspension, upwelling, and mixing, underscoring the need to consider multiple transport processes when assessing the distribution of PFAS (Figure 2D; Zhang et al., 2017).
2.3.1 Currents of contamination: PFAS in oceanic pathways
Ocean currents serve as a slow but significant pathway for the long-range transport of PFAS, gradually moving these pollutants from industrial areas to remote Arctic regions over decades (Joerss et al., 2020). This process contributes to the steady accumulation of PFAS, particularly long-chain compounds in the cryosphere regions (Ahrens et al., 2023; Hartz et al., 2023). Oceanic transport of PFAS to the Arctic cryosphere is substantial, with significant mass flows reported through key gateways like the Fram Strait (Joerss et al., 2020).
Global ocean models, such as the Berkeley-Trent Global Model (BETR), have shown that ocean currents are the primary transport route for PFOA-like long-chain PFCAs to enter polar waters (Armitage, 2009; Stemmler and Lammel, 2010). For example, the Norwegian Sea is a major PFAS pathway, particularly for PFOA, which moves northward into the Arctic (Stemmler and Lammel, 2010). Similarly, in the Mid-Atlantic, PFAS concentrations remain highest in surface waters, as isolation from deeper layers creates a long residence time in warmer surface currents like the Gulf Stream and North Atlantic drift. In contrast, PFAS levels in the South Pacific Ocean are low from surface to depth, reflecting limited inputs and influence from older, deep currents (Stemmler and Lammel, 2010).
2.3.2 Oceanic transport and impact on PFAS storage in the cryosphere
The cryosphere serves as both a PFAS reservoir and a secondary contamination source. Less volatile PFAS are deposited via aerosols, accumulating in water bodies, sediments, marine ice, and vegetation through cold trapping (Bengtson Nash et al., 2010; Wild et al., 2015; Sha et al., 2022; Egas et al., 2023; Xie and Kallenborn, 2023). Increased melting glaciers due to climate change may release previously trapped PFAS into the Arctic waters. This contamination effect is compounded by the weakening of the Atlantic Meridional Overturning Circulation (AMOC), which alters the distributional dynamics of PFAS in the oceans (Zhang et al., 2017).
The Arctic Ocean is predicted to become a significant PFOS reservoir, potentially storing between 63 and 180 t in the future (Yeung et al., 2017). Moreover, long-chain and emerging short-chain PFAS show significant temporal changes and are transported by ocean currents, resulting in consistent exposure over time within environmental matrices (Ahrens et al., 2023). The shift from long-chain to short-chain PFAS production in recent years has altered the types of PFAS reaching the Arctic. With rapid atmospheric responses to industrial changes, the Arctic is experiencing higher concentrations of short-chain PFAS alongside legacy compounds like PFOA and PFOS, despite their phase-out in 2020 and 2009 (ECHA, 2024). Although certain PFAS precursors have decreased due to reduced production, recent studies indicate that PFAS levels have stabilized since around 2009–2010, suggesting ongoing exposure through ocean currents (Routti et al., 2017).
2.3.3 Atmospheric transport
Due to their persistent nature and capacity for long-range atmospheric travel, PFAS depositions occur in remote Arctic regions. This process may occur over days to weeks, making it a relatively rapid mechanism for distributing PFAS, particularly for short-chain PFAS and their volatile precursors (Wania, 2007; Blévin et al., 2017). Volatile precursors of PFAS, such as FTOH, are known to travel considerable distances before degrading into stable compounds like PFCAs (Van de Vijver et al., 2003).
In Antarctica, the atmospheric delivery of FTOH-derived ionic PFAS is influenced by wind patterns and the rate of FTOH oxidation, which has a modest yield of stable PFAS (3%–10%) (Wania, 2007). Estimates suggest that about 40% of North American FTOH production has been linked to ionic PFAS contamination in Canadian Arctic ice caps, emphasizing atmospheric pathways as primary sources of PFAS in these regions (Young et al., 2007). Casas et al. (2023) reported a higher concentration of per- and polyfluoroalkyl acids (PFAA) in snow, emphasizing the role of atmospheric deposition in introducing PFAA to Antarctica, as demonstrated by their presence in aerosols, rain, snow, and small lakes.
Laboratory and modeling studies highlight that sea spray aerosols (SSA) are a significant source of PFAS in the atmosphere and are capable of long-range transport (Reth et al., 2011; Johansson et al., 2019; Casas et al., 2020). While SSA formation and organic matter enrichment are understood, field data on PFAS concentrations in marine sea surface microlayers, crucial for aerosol formation, is limited. More field measurements are needed to enhance our understanding of PFAS emission and transport dynamics through SSA-mediated processes (Ju et al., 2008; Figure 2).
Seasonal variations in PFAS concentrations of PFAS indicate that atmospheric oxidation is a critical contributor to Arctic contamination (Young et al., 2007). High levels in winter are likely linked to increased deposition events that gather PFAS on glaciers, frozen lakes, and streams (Muir et al., 2019). Snow pit samples from the high Arctic ice caps further confirm these atmospheric deposition patterns (Hartz et al., 2023).
2.3.4 Snow, glacial meltwater, and permafrost thaw as PFAS transport vectors
Snow and glacial melt water are crucial pathways for contaminant transport in cryosphere regions. As an effective scavenger, snow captures PFAS from atmospheric deposition, leading to contamination even in remote polar, the Tibetan Plateau, and the Alps regions (Benskin et al., 2011; Wang et al., 2014; Pickard et al., 2018; Casal et al., 2019; Chen et al., 2019; Skaar et al., 2019; Wang et al., 2019). The interplay of atmospheric deposition, meltwater mixing, and permafrost thaw accelerates the spread of PFAS (Vonk et al., 2015; Gruber et al., 2017; Jones et al., 2022). The Antarctic “snow amplification effect” demonstrates how snow scavenges persistent organic pollutants (POP), including PFAS, from the atmosphere, which are later released into seawater during snowmelt (Casal et al., 2019; Casas et al., 2020; Casas et al., 2023).
Warming-induced snow and glacier melt contribute to remobilizing both recently deposited and legacy PFAS, leading to increased contamination of freshwater and marine environments. Elevated PFAS concentrations have been documented in soils, permafrost layers, and lake sediments downstream from glaciers (Chen et al., 2019; MacInnis et al., 2019b; Zhou et al., 2024a). Recent studies indicate that freshly deposited snow in Antarctica contained higher concentrations of PFCA compared to coastal seawater, with a concentration ratio ranging from one to over 30 ratios, increasing with the alkylated chain length of PFAS (Casal et al., 2017a; Casas et al., 2023; Bargagli and Rota, 2024). Similarly, in the Lake Hazen watershed, Canada, higher PFAS concentrations were detected upstream, indicating snowmelt as the primary transport mechanism integrating inputs from atmospheric sources and permafrost thaw (MacInnis et al., 2022; Figures 2B, D).
Recent studies have also detected novel PFAS compounds in the Arctic and Antarctica, such as HFPO-DA and fluorotelomer sulfonates (6:2 and 8:2 FTS). These compounds were substitutes for traditional PFAS like PFOS and PFOA (Zhou et al., 2024a). In Antarctica, 16 PFAS were measured in snow samples collected at the Dome C ice core drilling station (French/Italian Station Dome Concordia, Wilkes Land, East Antarctica) in the summer of 2016, with PFOA identified as the dominant compound, followed by perfluoroheptanoic acid (PFHpA), perfluorohexanoic acid (PFHxA), and perfluoropentanoic acid (PFPeA). Long-chain PFCA (C9-C14) represented 10% of the total PFCA content, while PFOS, perfluorobutane sulfonate (PFBS), and PFHxS were the predominant PFSA. Notably, HFPO-DA (4.7–13.0 pg/L) was detected in Antarctic snow for the first time (Xie et al., 2015). In high-Arctic ice cores, PFOA (66 pg/L) detected in ice cores during 1977–2015 have been regarded as the background levels, likely resulting from atmospheric deposition (Pickard et al., 2018; Joerss et al., 2020).
2.3.5 PFAS trapped in sea ice and released when it melts
Sea ice is a critical reservoir and transport vector for PFAS and POP. During winter, sea ice acts as a seasonal “sink,” accumulating PFAS and other contaminants. Early models predicted that the decline of sea ice and subsequent increases in open water would enhance the exchange of chemicals between the atmosphere and ocean surface waters, raising exposure levels in marine environments (Stemmler and Lammel, 2010; Dunn et al., 2024). However, recent research revealed that PFAS behavior in sea ice involves complex interactions between contaminant retention, seasonal ice dynamics, and environmental release processes (Yeung et al., 2017; Hartz et al., 2023).
Various types of PFAS have been detected in ice and water samples at different concentrations from the cryosphere (Figure 4). For instance, PFBA was the dominant PFAS in the ice of Canadian Lake Hazen in 2013 and 2014, followed by PFNA, PFOA, and PFDA (MacInnis et al., 2019a). Recently, a large number of PFAS were detected in an ice core from the Lomonosovfonna ice cap on Svalbard in the Arctic, where short-chain PFAS, such as trifluoroacetic acid (TFA), perfluoropropionic acid (PFPrA) and PFBA dominated atmospheric deposition fluxes. These findings align with similar results from Canada’s High Arctic (Pickard et al., 2018; Hartz et al., 2023). Interestingly, the occurrence of long-chain PFAS, like perfluorododecanoic acid (PFDoDA) and perfluorotridecanoic acid (PFTrDA), was substantially higher in the Lomonosovfonna ice core (82%) compared to the Devon Ice Cap core, Canada (13%) (von Appen, 2018). Additionally, in both polar regions, glacier melting and sea ice retreat serve as secondary sources of PFAS, releasing previously deposited contaminants back into the environment as ice and snow thaw (Xie and Kallenborn, 2023).
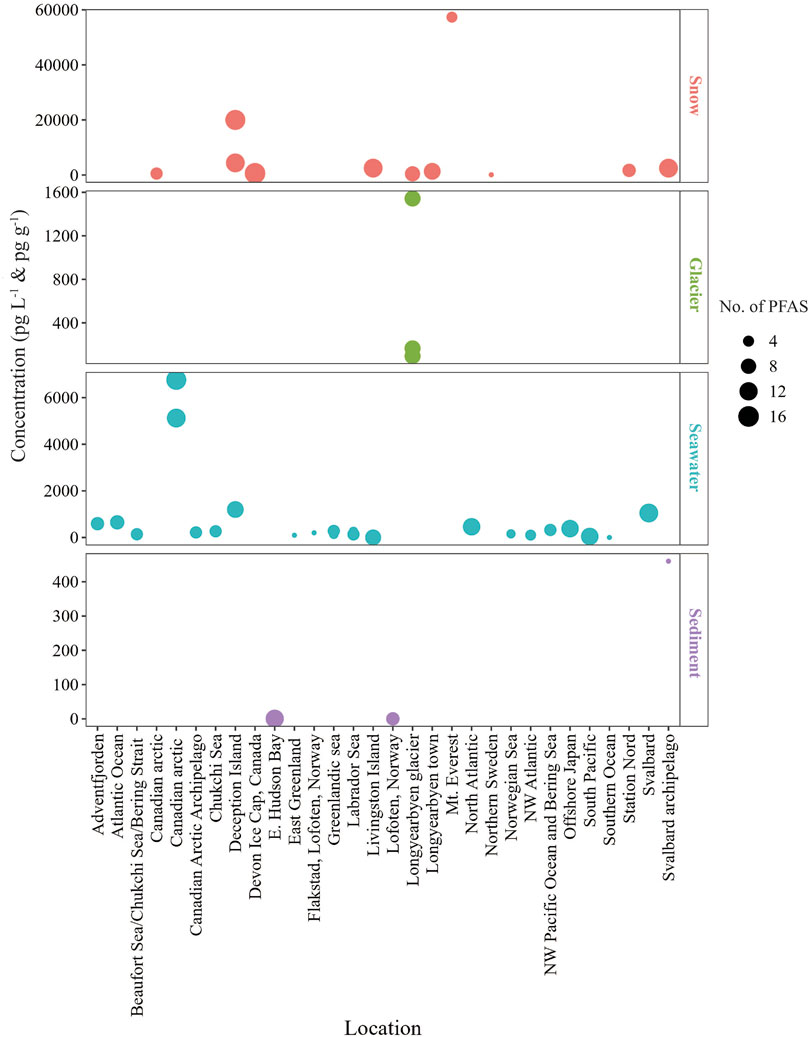
Figure 4. PFAS levels in distinct cryosphere compartments across diverse geographic regions summarized based on the literature (Young et al., 2007; Yamashita et al., 2008; Benskin et al., 2012; Zhao et al., 2012; Kwok et al., 2013; Codling et al., 2014; Pickard et al., 2018; MacInnis et al., 2019a; Muir et al., 2019; Skaar et al., 2019; Miner et al., 2021; Ahrens et al., 2023; Casas et al., 2023). The right-side legend shows the number of PFAS analyzed in each sampling location (Supplementary Table S1).
2.3.6 Regional dynamics and barriers in PFAS oceanic distribution
PFAS concentrations reported from various studies show notable variations influenced by oceanic circulation. For instance, PFAS levels decreased from high-emission zones in Northern Europe toward the South Atlantic due to dilution and circulation effects (Joerss et al., 2020; Ahrens et al., 2010).
Regional studies underscore the impact of ocean currents on PFAS distribution in polar regions, where the Arctic experiences higher PFAS accumulation than Antarctica. Concentrations declined from the North Sea continental shelf toward the Norwegian Sea, and only PFOS was detected in the Antarctic circumpolar current zone (Bengtson Nash et al., 2010). However, several studies reported the presence of PFAS in remote regions of Antarctica (Figure 4; Table 1) (Bengtson Nash et al., 2010; Wild et al., 2015; Shan et al., 2021; Casas et al., 2023). Though oceanic currents mainly facilitate the transport of PFAS on a global scale, the Antarctic circumpolar currents act as a barrier, limiting the influx of PFAS from northern marine regions to the Southern Ocean (Bengtson Nash et al., 2010; Stemmler and Lammel, 2010; Benskin et al., 2012). Quantitative estimates suggested that approximately 123 t of PFAS flow annually into the Arctic Ocean from the North Atlantic, with 110 t returning to the Atlantic (Dunn et al., 2024). This pattern highlights an extensive and ongoing circulation of PFAS within northern waters, impacting surface and deeper ocean layers (Sha et al., 2022).
2.4 PFAS transformation and degradation pathways in the cryosphere
2.4.1 Atmospheric oxidation and photodegradation
PFAS, particularly volatile PFAS precursors, such as FTOH and perfluoroalkyl sulfonamides (FASA), undergo oxidative transformation in the atmosphere. These reactions are primarily driven by hydroxyl radicals (•OH) and photochemical processes, particularly during the polar summer when sunlight exposure is prolonged (Garnett et al., 2019; Hartz et al., 2024; Figure 3B). Oxidation leads to the formation of PFCAs, such as PFOA and PFNA, which are more persistent and water-soluble, facilitating their deposition in cold environments. The yield of long-chain PFCA is higher in remote areas due to low NOx conditions, which are typical for cold areas (Sulbaek Andersen et al., 2005; Young et al., 2007).
PFOA undergoes degradation via photochemical oxidation initiated by UV radiation. This process is enhanced by high snow reflectivity (albedo)) of snow, increasing UV penetration during the Arctic summer, and the presence of ice-induced reactive species (OH⋅, HO⋅2). The secondary products of FTOH oxidation, such as PFOA, PFNA, and PFDA, can enter seawater via wet and dry deposition and subsequently undergo sea spray aerosol (SSA), acting) which act as a re-emission pathway for these PFAS into the atmosphere. However, FTOH atmospheric oxidation is a chemical transformation process, while SSA is a physical process of PFAS from seawater to the atmosphere via bubble bursting and wave action (Figure 2).
2.4.2 Aqueous phase transformation
In cold environments, PFAS transformation exhibits slow reaction kinetics as it can be influenced by unique low temperatures and more prolonged UV exposure in summer. These factors affect the rates and pathways of PFAS degradation (Figure 3C). PFAS can undergo direct photolysis when exposed to UV radiation, leading to cleavage of carbon-fluorine bonds (C-F) (Xin et al., 2023). Therefore, the photolysis rate and its extent are influenced by the molar absorptivity of particular PFAS compounds.
The reduction process involves reactions where PFAS molecules gain electrons, leading to fluorination. In cold environments, the presence of electron donors such as ferrous ions (Fe2+) or bisulfide (HS−) can facilitate such chemical reactions. Exposure to artificial UV-C can induce PFAS degradation of PFAS, by generating hydrated electrons that can attack PFAS molecules, resulting in the cleavage of C-F bonds with subsequent defluorination (Yin and Villagrán, 2022).
Hydrolysis involves the reaction of PFAS with water, leading to the formation of carboxylated products. While PFAS are generally resistant to hydrolysis due to the strength of the C-F bonds, certain precursor compounds can undergo hydrolytic transformation under specific environmental conditions. For example, perfluorooctane sulfonyl fluoride (POSF) can hydrolyze to produce PFOS. The hydrolysis reactions can be influenced by temperature, pH, and the presence of catalysts (Interstate Technology and Regulatory Council, 2023).
2.4.3 Sediment and soil transformation
Permafrost thaw significantly influences the transport of PFAS in Arctic freshwater systems and soils (Schuur et al., 2015). Mostly, permafrost acts as a reservoir for airborne PFAS, and climate-induced thawing now transforms these frozen aquatic systems into a source of PFAS pollution (Gruber et al., 2017; MacInnis et al., 2022).
The release of PFAS from thawing permafrost induced by local pollution sources, such as sewage and landfills, creates a complex interaction of multiple contamination pathways (Garcia-Barrios et al., 2021; Alfaro Garcia et al., 2022). Warming accelerates freeze-thaw cycles, which alter PFAS mobilization, particularly affecting the distribution and chemical behavior of short-chain PFAS, which persist in deeper soil layers (Brendel et al., 2018). The efficiency of PFAS transport depends on the composition of thawed organic matter, the morphology of lakes and streams, and landscape characteristics, such as topography, soil type, and ground ice content (Vonk et al., 2015). Thawing permafrost further deepens the active soil and releases dissolved organic carbon and other nutrients that tend to change the water chemistry of nearby lakes and rivers, consequently impacting hydrological pathways (Roberts et al., 2017). Furthermore, releasing carbon-rich organic matter may increase the bioavailability of POP, amplifying their ecological risks (MacInnis et al., 2019a; MacInnis et al., 2022). This complex interplay shows how PFAS may be redistributed across the cryosphere.
PFAS binds to organic matter and mineral surfaces like clay, and iron oxide and manganese oxide act as sorbents, affecting PFAS mobility and degradation. Iron. Iron oxides in sediments enhance PFAS adsorption and influence degradation kinetics under reducing conditions (Figure 3D; Interstate Technology and Regulatory Council, 2023). Anoxic conditions in sediments favor reductive fluorination. This is usually effective when coupled with microbial degradation by sulfite-reducing bacteria (e.g., Desulfovibrio spp.), which enhances PFAS breakdown in aquatic sediments (Douna and Yousefi, 2023; Jin et al., 2023).
2.4.4 Biological transformation
The biological transformation of PFAS can be facilitated by microbes and their metabolic activities mediated by cold-adapted enzymes at low temperatures (Figure 3E). Enzymes like peroxidases or cytochrome P450 enhance defluorination (Beškoski et al., 2018; Huang and Jaffé, 2019). Pseudomonas and Dehalococcoides species have been detected in Arctic environments, where they use PFAS as terminal electron acceptors under anoxic conditions (Huang and Jaffé, 2019; Zhang Z. et al., 2022). Acidomicrobium species have been identified in diverse habitats, including cold environments such as soils and sediments, and they defluorinated PFOA and PFOS under Feammox conditions (where ammonium is oxidized while iron is reduced) (Huang and Jaffé, 2019).
3 Bioaccumulation and biomagnification impacts
3.1 PFAS far-reaching overall impacts on cryosphere
The environmental transport of PFAS inevitably leads to their accumulation in the cryosphere. PFAS concentrations are notably high in the cryosphere, with unique bioaccumulation patterns compared to other regions (Khan et al., 2023) with several studies documenting a range of compounds including long-chain PFAA, such as PFDA and PFOS, and newer replacement PFAS across multiple marine taxa (Table 2; Casal et al., 2017b; Alfaro Garcia et al., 2022; Khan et al., 2023; Zhang et al., 2023). These compounds accumulate in individual species and are also biomagnifying along the food chain, heightening exposure risks for top predators (Figures 2C, E). Recent toxicological research showed that PFAS exposure affects growth, behavior, reproduction, physiology, and genetic characteristics across various species (Zhao et al., 2012; Jouanneau et al., 2023; Manojkumar et al., 2023). PFAS contamination has been documented not only in wildlife but also in drinking water, groundwater, and even rain, underscoring their widespread environmental health risks (Boiteux et al., 2012; Table 1; Kim and Kannan, 2007; Schwanz et al., 2016; Kim et al., 2023; Olney et al., 2023; Sonne et al., 2023). Consequently, PFAS poses a complex threat to cryosphere ecosystems by affecting organisms across different trophic levels (Figure 2C; Tomy et al., 2004; Muir et al., 2019; Ali et al., 2021).
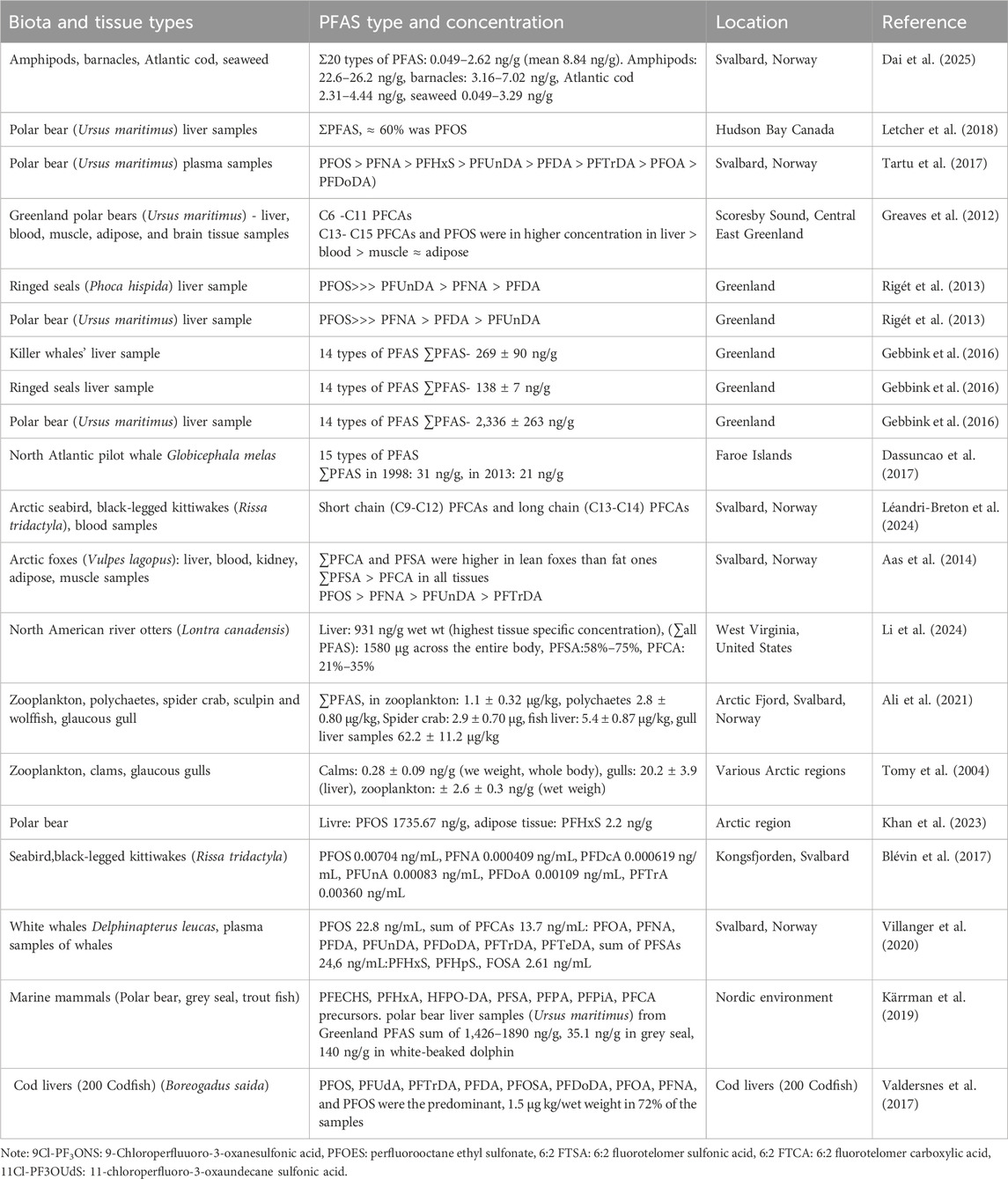
Table 2. PFAS documented in various tissues and organisms around the cryosphere regions (If there are more than eight types of PFAS studied in a particular location, the most abundant type, and their concentration are given; otherwise, the sum of all identified PFAS is provided).
3.2 Bioaccumulation in apex predators and marine mammals
Apex predators in cryosphere ecosystems, including seals, whales, and polar bears, are exposed to significant challenges from PFAS contamination (Table 2; Alfaro Garcia et al., 2022; Chen et al., 2023; Lu et al., 2024). Despite regulatory phase-outs of C8-based PFAS, recent studies have shown that PFOS and C9 - C11 PFCA generally persist in tissue samples from polar bears (Ursus maritimus) (liver) and ringed seals (Phoca hispida) in regions like Greenland (Figure 2; Rigét et al., 2013; Gebbink et al., 2016; Dietz et al., 2019) and Hudson Bay (Routti et al., 2017; Letcher et al., 2018). Furthermore, elevated PFSA (C4, C6, C8, and C10) levels in the liver samples of higher trophic level species, such as East Greenland polar bears, with concentrations reaching 2,611 ± 202 ng/g wet weight, predominantly consisting of PFOS were also reported (Boutet et al., 2023). The mean ∑4 PFSA in seal liver was 111 ± 5 ng/g wet weight with 98% PFOS. This concentration was three orders of magnitude higher than in seal blubber, which had a concentration of 0.05 ± 0.01 ng/g ww, consisting entirely of PFHxS. Biomagnification factors (BMFs) were significantly higher for PFHxS and C9 to C13 PFCA when comparing polar bear liver to seal liver. BMFs decreased as chain length increased, like PFCA (C9 to C13) (Kärrman et al., 2019; Boutet et al., 2023). Therefore, BMF comparison between seal blubber and bear liver more accurately reflects the dietary exposure of PFAS from seals to bears (Boutet et al., 2023). Specifically, the exposure of polar bears to PFAS has been associated with a range of health issues, such as hormonal imbalances and immune system impairment (Miner et al., 2017; Dietz et al., 2019). A risk assessment study over three decades (1983–2013) indicated that the reproductive, immunotoxic, and carcinogenic effects of PFOS in East Greenland polar bears were significant during this period, with only a slight decline over the years (Dietz et al., 2019; Xie and Kallenborn, 2023).
In addition, PFAS biomagnification is particularly pronounced in air-breathing animals, likely due to their protein-affinity (proteinophilic nature) and respiratory differences compared to aquatic species (Chen et al., 2023; Kelly et al., 2009; Khan et al., 2023). For instance, studies on beluga calves in Hudson Bay indicate that PFOS and PFCA (C7-C14) levels exceed recommended exposure limits, with trophic magnification factors (TMFs) ranging from 2 to 11, suggesting potential health risks for Arctic marine life (Kelly et al., 2009). In addition, the research emphasizes that cetaceans, unlike other mammals, cannot convert precursor perfluorooctane sulfonamide (FOSA) into PFOS, which leads to different tissue burdens of both compounds (Dassuncao et al., 2017). FOSA accounted for many PFAS in North Atlantic pilot whales in 2000 but has significantly declined in recent years, while PFOS and other long-chain PFAS (C9-C13) have increased. This change is linked to shifts in atmospheric inputs of FOSA, especially after 2000, which have reduced PFOS exposure in species that metabolize FOSA, such as whales and seafood-consuming humans (Dassuncao et al., 2017). These findings stress the importance of considering PFAS precursors when studying bioaccumulation and environmental impacts.
3.3 Bioaccumulation in birds
Arctic seabirds, so far studied, always exhibited high PFAS concentrations in their tissues, leading to reduced reproductive success and impaired chick development (Ask et al., 2021; Jouanneau et al., 2023). Long-chain PFAS such as PFUnDA (C11) and PFTrDA (C13) are highly bioaccumulative, often exceeding PFOS levels in Arctic seabirds (Khan et al., 2023). These long-chain PFCA often exceed or are comparable to PFOS levels, as seen in species like northern fulmar (Fulmarus glacialis) and thick-billed murre (Uria lomvia). Although long-chain PFCA is increasingly dominant, PFOS remains a major fluorinated contaminant in Arctic bird species. For instance, extreme cases of PFOS concentrations have been reported in white-tailed eagle eggs (Haliaeetus albicilla) (1,514 ng/g wet weight) (Khan et al., 2023). Perfluoroundecanoic acid (PFUnA) concentrations in the blood of Arctic birds, such as glaucous gulls (Larus hyperboreus), have reached 74.4 ng/g wet weight. In contrast, egg concentrations of PFOA in Audouin gulls (Larus audouinii) were recorded at 23.53 ng/g, indicating significant PFAS burdens in these species.
In Antarctica, PFAS have been identified in the south polar skua (Catharacta maccormicki), highlighting biomagnification levels that vary between different colonies (Garcia-Barrios et al., 2021; Alfaro Garcia et al., 2022). Seven of the 22 PFAS analyzed were commonly found, with increasing concentrations from prey to skuas. Notably, PFOS levels were higher in skuas at Dumont d’Úrville (French research station, Antarctica) compared to other colonies, indicating complex source pathways and bioaccumulation trends (Alfaro Garcia et al., 2022). The findings emphasize the importance of diet and location in determining PFAS levels in cryosphere species.
3.4 Bioaccumulation in lower trophic levels
Sea ice facilitates the uptake of atmospherically deposited contaminants into marine ecosystems and is then released into the aquatic food web as the ice melts (Khan et al., 2023). This process supports phytoplankton blooms and subsequently increases zooplankton biomass, marking the start of a bioaccumulation chain that can extend up to higher trophic levels (Miner et al., 2018). Food web analysis revealed that bioaccumulation patterns in marine plankton are complex. Certain PFCA and PFOS exhibited higher-than-expected bioaccumulation factors (BAFs) in Arctic waters (Zhang et al., 2019). PFAS have been identified in both phytoplankton (Zhang Y. et al., 2022; Davis et al., 2024; Ma et al., 2022) and zooplankton (Miner et al., 2017), indicating their bioavailability at the base of the marine food chain (Rigét et al., 2013).
Zooplankton, vital for energy transfer in marine ecosystems, also showed significant PFAS accumulation. PFAS can disrupt reproductive and developmental processes, leading to population declines and altered food web dynamics (Ali et al., 2021). Changes in zooplankton populations can affect fish species that depend on them as food sources, causing ripple effects across the food web and affecting the entire ecosystem (Ren et al., 2022; Munoz et al., 2019). As larger organisms consume contaminated prey, PFAS concentrations increase with each trophic level, known as biomagnification, which raises significant ecological Ma et al., 2022 concerns since primary producers and consumers form the foundation of every ecosystem.
4 PFAS regulation and phase-out: progress and challenges
4.1 Forever chemicals in focus
The chemical structure of PFAS contributes to their high stability and low degradability, posing a significant environmental challenge (Kannan et al., 2001). PFOS, PFOA, and other long-chain PFAA are often referred to as “legacy contaminants,” i.e., they are recognized to continue impacting ecosystems and human health long after their use has been reduced or even banned because of their persistence in the environment, tendency to bioaccumulate, and potential toxic effects (Chen et al., 2023). Among legacy compounds like polychlorinated biphenyls (PCB), dichlorodiphenyltrichloroethane (DDT), chlorofluorocarbons (CFC), and heavy metals, some long-chain PFAS are currently under regulation.
In contrast, replacement chemicals that are not yet regulated or included in routine monitoring programs are termed “contaminants of emerging concern” or “emerging contaminants” (Gaden et al., 2012). They can consist of either newly introduced compounds and their metabolites or molecules with previously unrecognized adverse effects on wildlife. PFAS has emerged as a growing concern in the past two decades as more than 5,000 varieties of synthetic highly fluorinated organofluoride substances were developed in this relatively short time frame (OECD, 2021; Gaines, 2023). This is mainly driven by the rising demand for products and their intriguing characteristics mentioned above (EU, 2019, 2021; OECD, 2021), as well as the result of the phase-out of some of the long-chain PFAS, leading to the use of replacement compounds like short-chain and ether-based PFAS (e.g., hexafluoropropylene oxide dimer acid: HFPO-DA, on the market as its ammonium salt GenX) (ECHA, 2024).
4.2 Historical efforts and global regulation
Several regulatory bodies and international agreements are currently addressing the management and regulation of PFAS in the Arctic and globally. The Stockholm Convention on Persistent Organic Pollutants is a global treaty that aims to eliminate or restrict the production of POPs, including certain PFAS. Only a comparatively small number of PFAS have been listed under the Stockholm Convention, i.e., PFOA and perfluorohexanoic sulfonic acid (PFHxS), their salts and related substances are included in Annex A, and PFOS and its salts are included in Annex B to regulate and control their production and application (UNEP, 2023; Davis et al., 2024; Hamid et al., 2024).
ECHA, operating under the European Union, has been instrumental in proposing restrictions and evaluating risks associated with various PFAS compounds to protect human health and the environment. The European Registration, Evaluation, Authorization and Restriction of Chemicals (REACH) Regulation aims to identify and restrict the use of substances of very high concern, including many PFAS with long-chain fluorinated carbon (C9-C14) atoms (Teymourian et al., 2021; ECHA, 2024). Temporal trends, like those observed in Sweden, show declining concentrations of certain PFAS, indicating the effectiveness of such regulatory measures (Haque et al., 2023). Recently, it has been proposed to restrict the entire group of PFAS unless a specific use is proven essential for society under REACH (ECHA, 2024).
Individual European countries have implemented bans on specific PFAS, citing their toxicological effects according to the European Food Safety Authority (EFSA), European Human Biomonitoring Initiative (HBM4EU) and the inadequacy of replacement PFAS (Schrenk et al., 2020). Advocacy groups and responsible companies call for immediate eradication of PFAS through enhanced regulatory frameworks, such as United Kingdom REACH and global conventions (Fernández, 2024; REACH, 2024). Many of these efforts are being carried out in European and North American regions where environmental concerns and consumer pressures often drive businesses to adopt more sustainable practices ahead of regulatory requirements (Magazine, 2024).
Arctic Council working groups, like the Arctic monitoring and assessment programme (AMAP) and the Arctic contaminants action program (ACAP), are addressing PFAS contamination in the Arctic regions (ACAP, 2021). AMAP conducts scientific pollution assessments of pollution, including PFAS, and provides policy-relevant advice to protect Arctic ecosystems and communities from these harmful contaminants (Arctic Council, 2024). ACAP focuses on preventing and reducing pollutants and provides policy recommendations to nations to implement actions that mitigate environmental health risks associated with contaminants (AFFF, 2021).
The US EPA addresses PFAS contamination through comprehensive strategies and regulatory actions. Step vice series of action plans from October 2021, the agency announced a Strategic Roadmap outlining plans to confront PFAS pollution, including developing national drinking water standards for PFOA, PFOS, and PFBS (US EPA, 2024). Recently, they have been expanding the list of PFAS that are subject to reporting under the Toxics Release Inventory (TRI) as mandated by the National Defense Authorization Act (NDAA). This act provides a framework for the annual addition of PFAS to the TRI list (US EPAO, 2019; US EPAO, 2025).
Environmental and Climate Change Canada (ECCC) is considering a class-based approach to address PFAS under the Canadian Environmental Protection Act, 1999 (CEPA), moving beyond individual substance assessments (ECCC, 2024). The latest ECCC draft report includes fluoropolymers, acknowledging their distinct exposure and hazard profiles. This class-based approach addresses the potential cumulative effects of exposure to PFAS mixtures and prevents the substitution of regulated PFAS with unregulated ones that may have similar hazardous properties. This strategy aligns with international efforts, as other jurisdictions, such as the European Union and certain U.S. states, are also exploring class-based approaches to PFAS regulation (ECCC, 2024).
4.3 Transition to alternatives and challenges
Despite being introduced as “safer” PFAS alternatives, many of these molecules share similar negative characteristics with legacy PFAS in terms of their persistence, broader mobility, and toxicity with varying degrees of environmental harm (Wang et al., 2015a). PFAS alternatives from PFCAs were perfluroethercarboxylic acids (PFECAs), perfluoroalkylethersulfonic acids (PFESAs), C6 fluorotelomer-based compounds, polyfluorinated alkyl phosphoric acid esters (PAPs) and chlorinated polyfluorinated ether sulfonic acids (Cl-PFESAs) are the currently common PFAS replacement compounds marketed, and they have been identified with potential accumulation and health effects (OECD, 2022; Feng et al., 2024; Table 3).
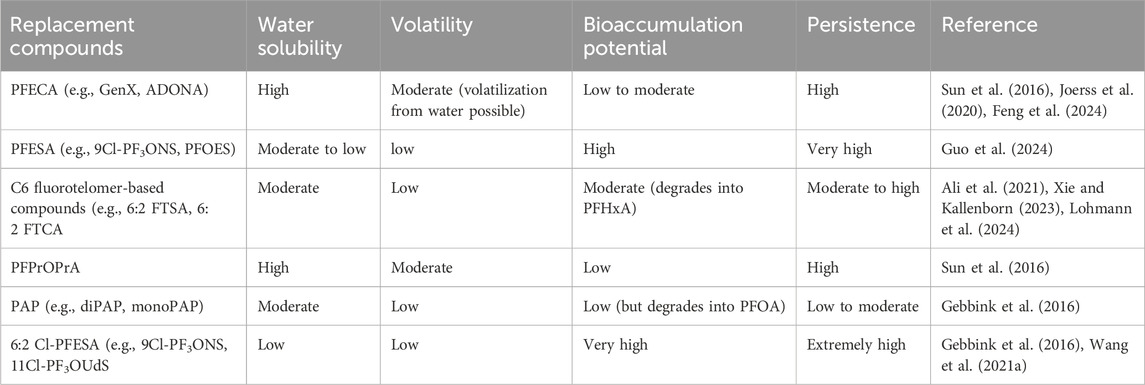
Table 3. Most common PFAS alternatives identified in cold environments and their environmental fate and potential transformation (Wang et al., 2015a; US EPA, 2021; Hamid et al., 2024).
Cl-PFESAs, such as 6:2 Cl-PFESAs, are highly persistent in the environment due to their higher thermal and chemical stability, high toxicity, and more bioaccumulative than PFOS, raising serious health risks. They have been identified as bioaccumulated substances in various marine organisms, including gastropods, bivalves, crabs, shrimps, cephalopods, and fish. These compounds tend to magnify along the food chain, raising severe concerns about their health impacts (Feng et al., 2024).
C6 fluorotelomer-based compounds like FTOHs can transform into PFCA in the environment, and due to their moderate solubility and low volatility, they exhibit a higher persistence and bioaccumulation potential in cold environments (MacInnis et al., 2019b; Munoz et al., 2019). Studies have detected Cl-PFPECAs in vegetation and subsoils, indicating their environmental persistence and tendency to accumulate in vegetation, indicating potential for atmospheric transport and deposition (Davis et al., 2023). PAP, used in food packaging and paper coating, can degrade into PFOA and other PFCAs (OECD, 2022; Feng et al., 2024).
Of the PFECAs, GenX as an alternative for PFOA was recorded in Arctic waters, especially in the Fram Strait between Greenland and Svalbard, highlighting their persistence and ability to travel long distances from their source (Joerss et al., 2020). In addition to GenX, ADONA (4,8-dioxa-3-H-perfluorononanoic acid) also shares similar properties of GenX as it is resistant to hydrolysis, photolysis, and biodegradation (Haque et al., 2023).
The EPA’ toxicity assessment mentioned that GenX exhibits significant persistence and is more mobile than longer-chain PFAS, increasing their contamination risks through water and air. GenX is highly water soluble and exhibits mobility in aquatic environments, as well as transformation pathways like volatilization, allowing atmospheric transport and potential redistribution (U.S. EPA, 2022). Because of these characteristics, they are highly bioaccumulate and have an increasing trend of presence in different environmental matrices and organisms; the transition from legacy PFAS to GenX raises concerns as a “regrettable substitution,” albeit being increased application and environmentally benign, and poses a severe risk than even PFOA (EEA, 2023).
Since GenX and other replacement compounds, intended as a safer alternative, are identified with similar environmental and health risks to the compound they are replacing, their toxicity and regulatory scrutiny highlight the need for continued research and precautionary policies to assess the long-term exposure effects, mainly when they are present as a mixture with other contaminant scenarios. Thus, future restrictions or phase-outs are urgently needed for safer alternative fluoropolymer production without any persistent nature, hopefully becoming the next major industrial focus.
4.4 Voluntary measures
The voluntary commitments by manufacturers to cease the production and sale of PFAS-containing food contact substances reflect industry recognition of the need to address safety concerns. However, challenges remain in ensuring the complete elimination of PFAS exposure and mitigating the environmental legacy of their past use. Voluntary measures by industry players play a significant role in reducing PFAS emissions. Although some companies, such as Páramo and Finisterre, have phased out PFAS or pledged to do so by 2025, nearly half of the assessed brands lack plans to stop their use (REACH, 2024).
Several companies and retailers, such as IKEA, Lindex, H&M, COOP in Denmark, L´Oreal in Europe, Jack Wolfskin, Fjallraven, and Houdini Sportswear, have come forward to phase out PFAS from their supply chains (Magazine, 2024). However, it is not as successful as they had proposed; in particular, many outdoor clothes (various brands) still contain PFAS. Further, by January 2025, under the California Safer Clothes and Textiles Act (AB 1817), US-based outdoor apparel and equipment brands, along with major industry suppliers, are expected to eliminate PFAS from some product lines and supply chains following 10 years of advocacy by Green Science Policy Institute (ECHA, 2024). These initiatives reduce PFAS usage and put pressure on chemical manufacturers to develop safer alternatives. Moreover, these voluntary measures can serve as case studies for regulators, demonstrating that functional alternatives are visible and encouraging broader regulatory action.
4.5 PFAS management- future directions
Phaseout of PFAS and adopting safer alternatives are imperative for protecting the environment and public health. Through structured assessments of alternatives, robust regulatory measures using a class-based approach, and proactive industry initiatives, the transition away from PFAS could be effectively managed, paving the way for a safer and more sustainable future. Moreover, PFAS management by harmonizing measurement methods, enhancing supply chain transparency, industrial discharge control, introducing extended producer responsibility to shift remediation costs, and balancing regulations with essential PFAS uses (Feng et al., 2024) Besides, more funding is needed for research on PFAS alternatives and removal technologies, along with establishing best practices for safe PFAS concentrate management to prevent environmental re-contamination.
At the same time, a comprehensive assessment of PFAS alternatives is crucial due to significant data gaps in exposure ways, transport routes, environmental and biological fate, and long-term-health effects. Limited research on PFAS alternatives, both long and short-chain PFAS transformation or degradation mechanisms, lifecycle, production, and used impurities raise concerns about their long-term impact despite indications of a reduced hazard profile (Fernández, 2024). The OECD report highlights this regard, making it essential to address these uncertainties to ensure effective management and regulation of PFAS alternatives (OECD, 2024).
5 Coupling microbial degradation for PFAS management
5.1 Current methods and limitations
Due to the widespread presence of PFAS in remote regions of the Arctic and Antarctica, the challenges associated with these contaminants have sparked interest in whether microbial degradation could provide a viable remediation strategy that would complement existing physical and chemical techniques. These include photocatalytic reactions utilizing boron nitride and titanium dioxide, as well as non-photocatalytic methods using dimethylsulfoxide (DMSO) which lead to the mineralization of certain PFAS (Mahinroosta and Senevirathna, 2020; Senevirathna et al., 2022; Garg et al., 2023; Kang et al., 2023; Antonopoulou et al., 2024). Other physicochemical methods such as adsorption using colloidal activated carbon, pyrolysis, reduction methods using iodide, sulfite, and dithionite, thermal treatments like incineration and microwave-hydrothermal processing, ion exchange, electrochemical oxidation, and some other treatment processes like ball milling have also been investigated for PFAS treatment (Merino et al., 2016; Mahinroosta and Senevirathna, 2020). While these methods have shown effectiveness in PFAS mineralization, they often require significant energy inputs or specialized equipment.
In contrast, microbial degradation offers a potential alternative, often cheaper approach by utilizing microorganisms’ natural capacity to degrade pollutants, even though traditional microbial treatment or remediation techniques that effectively degrade other organic pollutants have mainly proven ineffective against PFAS (Ross et al., 2018). Some studies indicate that PFAS, particularly PFOA and PFOS, resist biodegradation under various environmental conditions (Liou et al., 2010).
Despite these challenges, recent research has identified specific microbial species capable of degrading particular PFAS compounds under controlled conditions, such as mixed cultures (Huang and Jaffé, 2019; Chiavola et al., 2020; Choi and Kan, 2024). This suggests that microbial degradation, although challenging, may be possible with further advancements. Success in microbial degradation depends on the ´biodegradation triangle´- the interplay between the structural complexity of the PFAS compounds, microbial community composition, and environmental conditions (Zhang Z. et al., 2022).
5.2 Potential of polar microorganisms for PFAS bioremediation
Polar microorganisms, particularly those adapted to extreme cold (psychrophiles), show a unique potential for PFAS bioremediation due to their resilience and metabolic versatility (Egas et al., 2023). Psychrophiles are adapted to thrive at temperatures between −20°C and 20°C and have developed mechanisms to maintain cellular function in cold environments, such as enhanced membrane fluidity, cold-shock protein synthesis, and accumulation of cryoprotectants like mannitol (Feller and Gerday, 2003; Baraúna et al., 2017; Clarke et al., 2013).
Several studies identified the potential biodegrading capacity of polycyclic aromatic hydrocarbons (PAH) from polar microbes (Egas et al., 2023). Polar environments have been studied in this context, often focusing on bioprospecting for psychrophiles and polyextremophiles (Orellana et al., 2018; Pearce 2012; Thomas and Dickmann, 2002; Cavicchioli et al., 2002; Nizovoy et al., 2021).
Psychrophiles isolated from Antarctic regions, for instance, contain enzymes capable of degrading hydrocarbons at sub-zero temperatures, a function attributed to enzymes like alkane hydroxylases (Fuentes et al., 2014, Figure 3E; Bowman and Deming, 2014). Beyond hydrocarbon degradation, some Antarctic bacterial communities have demonstrated the capacity to reduce PFOS (Cerro-Gálvez et al., 2020). While not achieving complete degradation, this process promoted the growth of certain bacterial groups, such as Gammaproteobacteria, Roseobacter, and Flavobacteria. These observations signal microbial involvement in modifying PFAS compounds, albeit not entirely breaking them down Orellana et al., 2018; Pearce 2012; Thomas and Dickmann, 2002; Cavicchioli et al., 2002 (MacInnis et al., 2019b).
Besides, environmental toxicity of PFAS studies found that Pseudomonas lineages have the potential to break down PFOA (Yi et al., 2019), PFOS (Chetverikov et al., 2017), and other PFAA (Zhang Z. et al., 2022; Smorada et al., 2024). Specific bacterial genera like Rhodanobacter and Chujaibacter were dominant in PFAS-contaminated soils (Senevirathna et al., 2022). Their growth and degradation of PFAS-enriched materials showed potential bioremediation insights under varying physio-chemical conditions (Smorada et al., 2024). However, despite established links between biodegradation, bacterial communities, and gene expression in laboratory settings, there remains a significant knowledge gap in our understanding of PFAS biodegradation, particularly in natural environments.
5.3 Biotechnological insights and research directions
The metabolic pathways used by cold-adapted microorganisms for organic pollutant degradation, such as those for PCBs and PAHs, could serve as models for PFAS degradation (Bamforth and Singleton, 2005; Crisafi et al., 2016; Gran-Scheuch et al., 2017; Papale et al., 2017). Strains of Salinibacter, Arthrobacter, and Pusillimonas from Svalbard possess biphenyl dioxygenase (bphA) and have the potential to metabolize PCB mixtures at low temperatures (Papale et al., 2017; Papale et al., 2022). These findings suggest that similar microbial mechanisms, e.g., dehalogenation oxidation by members of the Actinobacteria and Gammaproteobacteria, may likely occur for PFAS degradation in Arctic habitats.
Enzymatic analyses of polar microbes have revealed potential for degrading SVOCs, particularly several PAHs like anthracene, naphthalene, phenanthrene, PCBs, and PFOS (Egas et al., 2023). Genes encoding enzymes for sulfur metabolism in polar microbes, such as sulfonate and alkanesulfonate monooxygenases, could facilitate the degradation of PFAS, especially PFOS (Cerro-Gálvez et al., 2020). Several studies have documented polar microbes, mainly from Antarctica and the Arctic marine environment, that can potentially degrade SVOCs (Egas et al., 2023).
Laboratory studies have also indicated that Arctic marine bacteria possess phosphoesterases capable of hydrolyzing phosphate ester bonds in organophosphates, pointing to another potential pathway for PFAS degradation (Martinez-Varela et al., 2021). Additional studies identified various species of bacteria as well as fungi from polar regions, such as bacterial Sphingobium xenophagum, Burkholderia glathei, Paeniglutamicibacter sp., Psychrobacter spp., Pseudomonas sp., Rhodococcus sp., Polaromonas sp., and Pseudoalteromonas sp., as well as fungal Penicillium sp. and Aspergillus sp., exhibit diverse genetic and enzymatic machineries capable of degrading complex organic pollutants, suggesting a yet untapped potential for PFAS degradation (Gran-Scheuch et al., 2017; Singh et al., 2023).
In this context, the high stability of PFAS compounds suggests that complete microbial degradation is unlikely without significant advances in biotechnology and the discovery of new microbes or microbial pathways. Non-biological methods such as hydrolysis, pyrolysis, and chemical oxidation remain the most effective for breaking down these resilient chemicals (Garg et al., 2023). Nonetheless, continued exploration of microbial degradation pathways could lead to breakthroughs in mitigating the environmental impacts of PFAS. Despite the harsh conditions of cryosphere environments, psychrophiles’ metabolic versatility and resilience make them promising candidates for bioremediation efforts. They offer a cost-effective and environmentally friendly solution to mitigate PFAS pollution in polar and other cold environments. Further research is crucial to fully elucidate the underlying mechanisms and refine bioremediation strategies for effective PFAS degradation.
5.4 Synthetic solutions through genetically engineered microbes
Bioremediation of PFAS is a significant research area, focusing on developing efficient ways to improve the biodegradation capabilities of microorganisms and their enzymes, including using genetically engineered microbes (GEM) (Berhanu et al., 2023). The typical PFAS breakdown process involves three key steps: defluorination, carbon-fluorine chain shortening, and mineralization, resulting in harmless fragments or by-products. Studies identified certain microbial groups and their enzymes have greater potential, making them ideal candidates for GEM applications (Huang and Jaffé, 2019; Wackett and Robinson, 2020; Yu et al., 2020).
Relevant enzymes identified for their potential C-F bond cleavage can be further studied and incorporated into psychrophilic bacteria capable of rapid evolutionary adaptation to enhance their PFAS degradation. For example, hydrolytic defluorinase from Streptomyces spp has been shown to metabolize fluoroacetate to glycolate (Berhanu et al., 2023), showing the breaking mechanism of PFAS. In addition, oxygenases such as alkane monooxygenases can shorten the chain by adding hydroxyl groups into carbon atoms, completing the activity of hydrolytic enzymes in breaking C-F bonds (Huang and Jaffé, 2019).
Cold-adapted bacteria like Polaromonas vacuolate, host dehalogenases that can be used alongside hydrolytic enzymes to enhance defluorination capabilities (Martinez Alvarez et al., 2022). Similarly, dehalogenases from the Acidimicrobium sp. Strain A6 facilitates PFAS reduction through defluorination (Huang and Jaffé, 2019; Wackett and Robinson, 2020). Other psychrophiles, including Polaromonas spp., Psychrobacter spp., Colwellia psychreythraea, are also promising candidates for defluorination due to their hydrolytic enzyme systems (Bakermans et al., 2006; Bowman and Deming, 2014; Papale et al., 2017; Martinez Alvarez et al., 2022).
Biotechnological tools can be employed to improve enzyme activity and substrate specificity for PFAS to explore the potential of using the above microbes. Genetic modifications can induce synthetic pathway networks that promote oxidation and reduction, generating reactive intermediates for further breakdown or adding harmless by-products. Additionally, specific regulatory pathways can be engineered to activate PFAS-degrading enzymes when fluorinated compounds are accessible for further breakdown. Developing a GEM that integrates these competencies can provide a robust platform for PFAS remediation, supporting ongoing efforts to mitigate contaminants in the cryosphere and other diverse environments.
6 Conclusion: implications and urgent research needs
The dual abiotic pressures of rising temperatures and melting sea ice significantly foster the release of stored PFAS from ice and increase exposure through atmospheric deposition and ocean currents. However, the different physicochemical properties of PFAS, their accumulation in ice, their movements via marine and atmospheric pathways, and their transformation into persistent contaminants necessitate further studies to comprehend the specific underlying mechanisms in cold environments. Additionally, assessing long-term environmental risks in cryosphere regions and implementing regulatory measures for PFAS management is urgently needed. This requires comprehensive monitoring of PFAS levels, their physiological effects on critical species, and their cascading impact on ecosystem functioning.
On the other hand, addressing the existing PFAS in the vulnerable cryosphere environment necessitates reliable remediation techniques. Bioremediation presents a potential sustainable solution for PFAS removal. Psychrophiles play a unique and crucial role in cold-environment biogeochemical cycles, and some show promising PFAS degradation capabilities. Genetic engineering may enable the development of modified microbes by optimizing key enzymes to enhance defluorination efficiency. Therefore, future efforts should concentrate on bioremediation approaches utilizing genetically engineered microbial consortia adapted to cryosphere conditions. These strategies must be scalable, cost-effective, and environmentally friendly. At the same time, identifying and developing new, safer chemical alternatives, along with robust environmental management strategies and stringent regulatory frameworks, are essential for addressing the impact of PFAS.
Author contributions
AA: Conceptualization, Writing–original draft, Investigation, Methodology, Visualization, Formal Analysis. OV: Conceptualization, Funding acquisition, Resources, Supervision, Writing–review and editing, Data curation. UK: Data curation, Supervision, Writing–review and editing, Methodology. H-PG: Conceptualization, Data curation, Visualization, Writing–review and editing, Supervision. AS: Supervision, Writing–review and editing. ÓR: Supervision, Writing–review and editing, Resources, Methodology. HJ: Data curation, Writing–review and editing, Investigation, Validation. BS: Conceptualization, Funding acquisition, Methodology, Project administration, Supervision, Writing–review and editing.
Funding
The author(s) declare that financial support was received for the research and/or publication of this article. The Icelandic Research Fund has supported the work in the frame of the project “Per- and poly-fluoroalkyl substances (PFAS) meet Arctic and sub-Arctic marine diatoms and their associated symbionts” (RANNIS ID: 239548-051).
Acknowledgments
The authors gratefully acknowledge the support of the Icelandic Research Fund in the frame of the project “Per- and poly-fluoroalkyl substances (PFAS) meet Arctic and sub-Arctic marine diatoms and their associated symbionts” (RANNIS ID: 239548-051).
Conflict of interest
The authors declare that the research was conducted in the absence of any commercial or financial relationships that could be construed as a potential conflict of interest.
Generative AI statement
The author(s) declare that Generative AI was used in the creation of this manuscript. ChatGPT, a language model developed by OpenAI for language improvement, was used for stylistic polishing, but the authors completed the final writing and revisions in their own voice.
Publisher’s note
All claims expressed in this article are solely those of the authors and do not necessarily represent those of their affiliated organizations, or those of the publisher, the editors and the reviewers. Any product that may be evaluated in this article, or claim that may be made by its manufacturer, is not guaranteed or endorsed by the publisher.
Supplementary material
The Supplementary Material for this article can be found online at: https://www.frontiersin.org/articles/10.3389/fenvs.2025.1559941/full#supplementary-material
References
Aas, C. B., Fuglei, E., Herzke, D., Yoccoz, N. G., and Routti, H. (2014). Effect of body condition on tissue distribution of perfluoroalkyl substances (PFASs) in arctic fox (Vulpes lagopus). Environ. Sci. Technol. 48, 11654–11661. doi:10.1021/es503147n
ACAP (2021). Aqueous film-forming foam (AFFF) Phaseout in the Arctic factsheet (Fact Sheet). Arctic. Council. Available online at: http://hdl.handle.net/11374/2737.
AFFF (2021). Aqueous film-forming foam (AFFF) Phaseout in the Arctic factsheet. Available online at: https://oaarchive.arctic-council.org/items/670868f4-d8bc-4cd3-ac4e-feb995bc29a0 (Accessed February 24, 2025).
Ahrens, L., Rakovic, J., Ekdahl, S., and Kallenborn, R. (2023). Environmental distribution of per- and polyfluoroalkyl substances (PFAS) on Svalbard: local sources and long-range transport to the Arctic. Chemosphere 345, 140463. doi:10.1016/j.chemosphere.2023.140463
Ahrens, L., Xie, Z., and Ebinghaus, R. (2010). Distribution of perfluoroalkyl compounds in seawater from northern Europe, atlantic ocean, and Southern Ocean. Chemosphere 78, 1011–1016. doi:10.1016/j.chemosphere.2009.11.038
Alfaro Garcia, L. A., Descamps, S., Herzke, D., Chastel, O., Carravieri, A., Cherel, Y., et al. (2022). Bioaccumulation of per and polyfluoroalkyl substances in antarctic breeding south polar skuas (Catharacta maccormicki) and their prey. Front. Mar. Sci. 9. doi:10.3389/fmars.2022.819525
Ali, A. M., Langberg, H. A., Hale, S. E., Kallenborn, R., Hartz, W. F., Mortensen, Å.-K., et al. (2021). The fate of poly- and perfluoroalkyl substances in a marine food web influenced by land-based sources in the Norwegian Arctic. Environ. Sci. Process. Impacts 23, 588–604. doi:10.1039/D0EM00510J
Antonopoulou, M., Spyrou, A., Tzamaria, A., Efthimiou, I., and Triantafyllidis, V. (2024). Current state of knowledge of environmental occurrence, toxic effects, and advanced treatment of PFOS and PFOA. Sci. Total Environ. 913, 169332. doi:10.1016/j.scitotenv.2023.169332
Arctic Council (2024). 6 facts about PFAS and Arctic Council efforts to address threats posed by them. Available online at: https://arctic-council.org/news/6-facts-about-pfas-and-arctic-councils-efforts-to-address-threats-posed-by-them/(Accessed February 24, 2025).
Armitage, J. M. (2009). Modeling the global fate and transport of perfluoroalkylated substances (PFAS). Available online at: https://urn.kb.se/resolve?urn=urn:nbn:se:su:diva-8571 (Accessed September 10, 2024).
Ask, A. V., Jenssen, B. M., Tartu, S., Angelier, F., Chastel, O., and Gabrielsen, G. W. (2021). Per- and polyfluoroalkyl substances are positively associated with thyroid hormones in an arctic seabird. Environ. Toxicol. Chem. 40, 820–831. doi:10.1002/etc.4978
Ásmundsdóttir, Á. M., and Scholz, B. (2021). “Effects of microplastics in the cryosphere,” in Handbook of microplastics in the environment. Editors T. Rocha-Santos, M. Costa, and C. Mouneyrac (Cham: Springer International Publishing), 1–46.
Bakermans, C., Ayala-Del-Río, H. L., Ponder, M. A., Vishnivetskaya, T., Gilichinsky, D., Thomashow, M. F., et al. (2006). Psychrobacter cryohalolentis sp. nov. and Psychrobacter arcticus sp. nov., isolated from Siberian permafrost. Int. J. Syst. Evol. Microbiol. 56, 1285–1291. doi:10.1099/ijs.0.64043-0
Bamforth, S. M., and Singleton, I. (2005). Bioremediation of polycyclic aromatic hydrocarbons: current knowledge and future directions. J. Chem. Technol. and Biotechnol. 80, 723–736. doi:10.1002/jctb.1276
Banks, R. E., and Tatlow, J. C. (1986). Synthesis of CF bonds: the pioneering years, 1835 – 1940. J. Fluor. Chem. 33, 71–108. doi:10.1016/S0022-1139(00)85272-0
Baraúna, R. A., Freitas, D. Y., Pinheiro, J. C., Folador, A. R. C., and Silva, A. (2017). A proteomic perspective on the bacterial adaptation to cold: integrating OMICs data of the psychrotrophic bacterium exiguobacterium antarcticum B7. Proteomes 5, 9. doi:10.3390/proteomes5010009
Bargagli, R., and Rota, E. (2024). Environmental contamination and climate change in Antarctic ecosystems: an updated overview. Environ. Sci. Adv. 3, 543–560. doi:10.1039/D3VA00113J
Bengtson Nash, S., Rintoul, S. R., Kawaguchi, S., Staniland, I., Hoff, J. v. d., Tierney, M., et al. (2010). Perfluorinated compounds in the Antarctic region: ocean circulation provides prolonged protection from distant sources. Environ. Pollut. 158, 2985–2991. doi:10.1016/j.envpol.2010.05.024
Benskin, J. P., Muir, D. C. G., Scott, B. F., Spencer, C., De Silva, A. O., Kylin, H., et al. (2012). Perfluoroalkyl acids in the atlantic and Canadian arctic oceans. Environ. Sci. Technol. 46, 5815–5823. doi:10.1021/es300578x
Benskin, J. P., Phillips, V., St. Louis, V. L., and Martin, J. W. (2011). Source elucidation of perfluorinated carboxylic acids in remote alpine lake sediment cores. Environ. Sci. Technol. 45, 7188–7194. doi:10.1021/es2011176
Berhanu, A., Mutanda, I., Taolin, J., Qaria, M. A., Yang, B., and Zhu, D. (2023). A review of microbial degradation of per- and polyfluoroalkyl substances (PFAS): biotransformation routes and enzymes. Sci. Total Environ. 859, 160010. doi:10.1016/j.scitotenv.2022.160010
Beškoski, V. P., Yamamoto, A., Nakano, T., Yamamoto, K., Matsumura, C., Motegi, M., et al. (2018). Defluorination of perfluoroalkyl acids is followed by production of monofluorinated fatty acids. Sci. Total Environ. 636, 355–359. doi:10.1016/j.scitotenv.2018.04.243
Blévin, P., Angelier, F., Tartu, S., Bustamante, P., Herzke, D., Moe, B., et al. (2017). Perfluorinated substances and telomeres in an Arctic seabird: cross-sectional and longitudinal approaches. Environ. Pollut. 230, 360–367. doi:10.1016/j.envpol.2017.06.060
Boiteux, V., Dauchy, X., Rosin, C., and Munoz, J.-F. (2012). National screening study on 10 perfluorinated compounds in raw and treated tap water in France. Arch. Environ. Contam. Toxicol. 63, 1–12. doi:10.1007/s00244-012-9754-7
Boitsov, S., Bruvold, A., Hanssen, L., Jensen, H. K. B., and Ali, A. (2024). Per- and polyfluoroalkyl substances (PFAS) in surface sediments of the North-east Atlantic Ocean: a non-natural PFAS background. Environ. Adv. 16, 100545. doi:10.1016/j.envadv.2024.100545
Borgå, K., McKinney, M. A., Routti, H., Fernie, K. J., Giebichenstein, J., Hallanger, I., et al. (2022). The influence of global climate change on accumulation and toxicity of persistent organic pollutants and chemicals of emerging concern in Arctic food webs. Environ. Sci. Process. Impacts 24, 1544–1576. doi:10.1039/D1EM00469G
Boutet, V., Dominique, M., Eccles, K., Branigan, M., Dyck, M., van Coeverden de Groot, P., et al. (2023). An exploratory spatial contaminant assessment for polar bear (Ursus maritimus) liver, fat, and muscle from northern Canada. Environ. Pollut. 316, 120663. doi:10.1016/j.envpol.2022.120663
Bowman, J. S., and Deming, J. W. (2014). Alkane hydroxylase genes in psychrophile genomes and the potential for cold active catalysis. BMC Genomics 15, 1120. doi:10.1186/1471-2164-15-1120
Brendel, S., Fetter, É., Staude, C., Vierke, L., and Biegel-Engler, A. (2018). Short-chain perfluoroalkyl acids: environmental concerns and a regulatory strategy under REACH. Environ. Sci. Eur. 30, 9. doi:10.1186/s12302-018-0134-4
Buck, R. C., Franklin, J., Berger, U., Conder, J. M., Cousins, I. T., de Voogt, P., et al. (2011). Perfluoroalkyl and polyfluoroalkyl substances in the environment: terminology, classification, and origins. Integr. Environ. Assess. Manag. 7, 513–541. doi:10.1002/ieam.258
Casal, P., Casas, G., Vila-Costa, M., Cabrerizo, A., Pizarro, M., Jiménez, B., et al. (2019). Snow amplification of persistent organic pollutants at coastal Antarctica. Environ. Sci. Technol. 53, 8872–8882. doi:10.1021/acs.est.9b03006
Casal, P., González-Gaya, B., Zhang, Y., Reardon, A. J. F., Martin, J. W., Jiménez, B., et al. (2017a). Accumulation of perfluoroalkylated substances in oceanic plankton. Environ. Sci. Technol. 51, 2766–2775. doi:10.1021/acs.est.6b05821
Casal, P., Zhang, Y., Martin, J. W., Pizarro, M., Jiménez, B., and Dachs, J. (2017b). Role of snow deposition of perfluoroalkylated substances at coastal livingston island (maritime Antarctica). Environ. Sci. Technol. 51, 8460–8470. doi:10.1021/acs.est.7b02521
Casas, G., Iriarte, J., D’Agostino, L. A., Roscales, J. L., Martinez-Varela, A., Vila-Costa, M., et al. (2023). Inputs, amplification and sinks of perfluoroalkyl substances at coastal Antarctica. Environ. Pollut. 338, 122608. doi:10.1016/j.envpol.2023.122608
Casas, G., Martínez-Varela, A., Roscales, J. L., Vila-Costa, M., Dachs, J., and Jiménez, B. (2020). Enrichment of perfluoroalkyl substances in the sea-surface microlayer and sea-spray aerosols in the Southern Ocean. Environ. Pollut. 267, 115512. doi:10.1016/j.envpol.2020.115512
Cavicchioli, R., Siddiqui, K. S., Andrews, D., and Sowers, K. R. (2002). Low-temperature extremophiles and their applications. Curr. Opin. Biotechnol. 13, 253–261. doi:10.1016/s0958-1669(02)00317-8
Cerro-Gálvez, E., Roscales, J. L., Jiménez, B., Sala, M. M., Dachs, J., and Vila-Costa, M. (2020). Microbial responses to perfluoroalkyl substances and perfluorooctanesulfonate (PFOS) desulfurization in the Antarctic marine environment. Water Res. 171, 115434. doi:10.1016/j.watres.2019.115434
Chen, M., Wang, C., Wang, X., Fu, J., Gong, P., Yan, J., et al. (2019). Release of perfluoroalkyl substances from melting glacier of the Tibetan plateau: insights into the impact of global warming on the cycling of emerging pollutants. J. Geophys. Res. Atmos. 124, 7442–7456. doi:10.1029/2019JD030566
Chen, Z., zhan, X., Zhang, J., Diao, J., Su, C., Sun, Q., et al. (2023). Bioaccumulation and risk mitigation of legacy and novel perfluoroalkyl substances in seafood: insights from trophic transfer and cooking method. Environ. Int. 177, 108023. doi:10.1016/j.envint.2023.108023
Chetverikov, S. P., Sharipov, D. A., Korshunova, T.Y., and Loginov, O. N. (2017). Degradation of perfluorooctanyl sulfonate by strain Pseudomonas plecoglossicida 2.4-D. Appl. Biochem. Microbiol. 53, 533–538. doi:10.1134/S0003683817050027
Chiavola, A., Di Marcantonio, C., Boni, M. R., Biagioli, S., Frugis, A., and Cecchini, G. (2020). “PFOA and PFOS removal processes in activated sludge reactor at laboratory scale,” in Frontiers in water-energy-nexus—nature-based solutions, advanced technologies and best practices for environmental sustainability. Editors V. Naddeo, M. Balakrishnan, and K.-H. Choo (Cham: Springer International Publishing), 375–377.
Choi, G., and Kan, E. (2024). Effects of perfluorooctanoic acid and perfluorooctane sulfonic acid on microbial community structure during anaerobic digestion. Bioresour. Technol. 393, 129999. doi:10.1016/j.biortech.2023.129999
Clarke, A., Morris, G. J., Fonseca, F., Murray, B. J., Acton, E., and Price, H. C. (2013). A low temperature limit for life on Earth. PLOS ONE 8, e66207. doi:10.1371/journal.pone.0066207
Codling, G., Halsall, C., Ahrens, L., Del Vento, S., Wiberg, K., Bergknut, M., et al. (2014). The fate of per- and polyfluoroalkyl substances within a melting snowpack of a boreal forest. Environ. Pollut. 191, 190–198. doi:10.1016/j.envpol.2014.04.032
Crisafi, F., Giuliano, L., Yakimov, M. M., Azzaro, M., and Denaro, R. (2016). Isolation and degradation potential of a cold-adapted oil/PAH-degrading marine bacterial consortium from Kongsfjorden (Arctic region). Rend. Fis. Acc. Lincei 27, 261–270. doi:10.1007/s12210-016-0550-6
Dai, S., Zhang, G., Dong, C., Yang, R., Pei, Z., Li, Y., et al. (2025). Occurrence, bioaccumulation and trophodynamics of per- and polyfluoroalkyl substances (PFAS) in terrestrial and marine ecosystems of Svalbard, Arctic. Water Res. 271, 122979. doi:10.1016/j.watres.2024.122979
Dassuncao, C., Hu, X. C., Zhang, X., Bossi, R., Dam, M., Mikkelsen, B., et al. (2017). Temporal shifts in poly- and perfluoroalkyl substances (PFASs) in North Atlantic pilot whales indicate large contribution of atmospheric precursors. Environ. Sci. Technol. 51, 4512–4521. doi:10.1021/acs.est.7b00293
Davis, M. J. B., Evich, M. G., Goodrow, S. M., and Washington, J. W. (2023). Environmental fate of Cl-PFPECAs: accumulation of novel and legacy perfluoroalkyl compounds in real-world vegetation and subsoils. Environ. Sci. Technol. 57, 8994–9004. doi:10.1021/acs.est.3c00665
Davis, S. N., Klumker, S. M., Mitchell, A. A., Coppage, M. A., Labonté, J. M., and Quigg, A. (2024). Life in the PFAS lane: the impact of perfluoroalkyl substances on photosynthesis, cellular exudates, nutrient cycling, and composition of a marine microbial community. Sci. Total Environ. 927, 171977. doi:10.1016/j.scitotenv.2024.171977
Dietz, R., Letcher, R. J., Desforges, J.-P., Eulaers, I., Sonne, C., Wilson, S., et al. (2019). Current state of knowledge on biological effects from contaminants on arctic wildlife and fish. Sci. Total Environ. 696, 133792. doi:10.1016/j.scitotenv.2019.133792
Douna, B. K., and Yousefi, H. (2023). Removal of PFAS by biological methods. Asian Pac. J. Environ. Cancer 6, 53–68. doi:10.31557/apjec.2023.6.1.53-68
Dunn, M., Vojta, S., Soltwedel, T., Von Appen, W.-J., and Lohmann, R. (2024). Passive sampler derived profiles and mass flows of perfluorinated alkyl substances (PFASs) across the Fram Strait in the North Atlantic. Environ. Sci. Technol. Lett. 11, 166–171. doi:10.1021/acs.estlett.3c00835
ECCC (2024). Updated draft state of per- and polyfluoroalkyl substances (PFAS) report. Available online at: https://www.canada.ca/en/environment-climate-change/services/evaluating-existing-substances/updated-draft-state-per-polyfluoroalkyl-substances-report.html (Accessed February 24, 2025).
ECHA (2024). Per- and polyfluoroalkyl substances (PFAS) - ECHA. Available online at: https://echa.europa.eu/hot-topics/perfluoroalkyl-chemicals-pfas (Accessed November 18, 2024).
EEA (2023). Cross-cutting story 3: PFAS — European environment agency. Available online at: https://www.eea.europa.eu/publications/zero-pollution/cross-cutting-stories/pfas (Accessed May 18, 2024).
Egas, C., Galbán-Malagón, C., Castro-Nallar, E., and Molina-Montenegro, M. A. (2023). Role of Microbes in the degradation of organic semivolatile compounds in polar ecosystems: a review. Sci. Total Environ. 879, 163046. doi:10.1016/j.scitotenv.2023.163046
Ellis, D. A., Martin, J. W., De Silva, A. O., Mabury, S. A., Hurley, M. D., Sulbaek Andersen, M. P., et al. (2004). Degradation of fluorotelomer alcohols: a likely atmospheric source of perfluorinated carboxylic acids. Environ. Sci. Technol. 38, 3316–3321. doi:10.1021/es049860w
Feller, G., and Gerday, C. (2003). Psychrophilic enzymes: hot topics in cold adaptation. Nat. Rev. Microbiol. 1, 200–208. doi:10.1038/nrmicro773
Feng, S., Lu, X., Ouyang, K., Su, G., Li, Q., Shi, B., et al. (2024). Environmental occurrence, bioaccumulation and human risks of emerging fluoroalkylether substances: insight into security of alternatives. Sci. Total Environ. 922, 171151. doi:10.1016/j.scitotenv.2024.171151
Fernández, S. G. (2024). Proactive approaches towards the phase-out of the PFAS family: The PFAS Movement status and a multi-national automotive enterprise towards hazardous chemicals phase-out. Sweden: University of Lund. Available online at: https://lup.lub.lu.se/student-papers/search/publication/9171566
Fuentes, S., Méndez, V., Aguila, P., and Seeger, M. (2014). Bioremediation of petroleum hydrocarbons: catabolic genes, microbial communities, and applications. Appl. Microbiol. Biotechnol. 98, 4781–4794. doi:10.1007/s00253-014-5684-9
Gaden, A., Ferguson, S. H., Harwood, L., Melling, H., Alikamik, J., and Stern, G. A. (2012). Western Canadian arctic ringed seal organic contaminant trends in relation to sea ice break-up. Environ. Sci. Technol. 46, 4427–4433. doi:10.1021/es204127j
Gaines, L. G. T. (2023). Historical and current usage of per- and polyfluoroalkyl substances (PFAS): a literature review. Am. J. Industrial Med. 66, 353–378. doi:10.1002/ajim.23362
Garcia-Barrios, J., Drysdale, M., Ratelle, M., Gaudreau, É., LeBlanc, A., Gamberg, M., et al. (2021). Biomarkers of poly- and perfluoroalkyl substances (PFAS) in Sub-Arctic and Arctic communities in Canada. Int. J. Hyg. Environ. Health 235, 113754. doi:10.1016/j.ijheh.2021.113754
Garg, A., Shetti, N. P., Basu, S., Nadagouda, M. N., and Aminabhavi, T. M. (2023). Treatment technologies for removal of per- and polyfluoroalkyl substances (PFAS) in biosolids. Chem. Eng. J. 453, 139964. doi:10.1016/j.cej.2022.139964
Garnett, J., Halsall, C., Thomas, M., Crabeck, O., France, J., Joerss, H., et al. (2021a). Investigating the uptake and fate of poly- and perfluoroalkylated substances (PFAS) in sea ice using an experimental sea ice chamber. Environ. Sci. Technol. 55, 9601–9608. doi:10.1021/acs.est.1c01645
Garnett, J., Halsall, C., Thomas, M., France, J., Kaiser, J., Graf, C., et al. (2019). Mechanistic insight into the uptake and fate of persistent organic pollutants in sea ice. Environ. Sci. Technol. 53, 6757–6764. doi:10.1021/acs.est.9b00967
Garnett, J., Halsall, C., Vader, A., Joerss, H., Ebinghaus, R., Leeson, A., et al. (2021b). High concentrations of perfluoroalkyl acids in arctic seawater driven by early thawing sea ice. Environ. Sci. Technol. 55, 11049–11059. doi:10.1021/acs.est.1c01676
Garnett, J., Halsall, C., Winton, H., Joerss, H., Mulvaney, R., Ebinghaus, R., et al. (2022). Increasing accumulation of perfluorocarboxylate contaminants revealed in an antarctic firn core (1958–2017). Environ. Sci. Technol. 56, 11246–11255. doi:10.1021/acs.est.2c02592
Gebbink, W. A., Bossi, R., Rigét, F. F., Rosing-Asvid, A., Sonne, C., and Dietz, R. (2016). Observation of emerging per- and polyfluoroalkyl substances (PFASs) in Greenland marine mammals. Chemosphere 144, 2384–2391. doi:10.1016/j.chemosphere.2015.10.116
Gran-Scheuch, A., Fuentes, E., Bravo, D. M., Jiménez, J. C., and Pérez-Donoso, J. M. (2017). Isolation and characterization of phenanthrene degrading bacteria from diesel fuel-contaminated antarctic soils. Front. Microbiol. 8, 1634. doi:10.3389/fmicb.2017.01634
Greaves, A. K., Letcher, R. J., Sonne, C., Dietz, R., and Born, E. W. (2012). Tissue-specific concentrations and patterns of perfluoroalkyl carboxylates and sulfonates in East Greenland polar bears. Environ. Sci. Technol. 46, 11575–11583. doi:10.1021/es303400f
Gruber, S., Fleiner, R., Guegan, E., Panday, P., Schmid, M.-O., Stumm, D., et al. (2017). Review article: inferring permafrost and permafrost thaw in the mountains of the Hindu Kush Himalaya region. Cryosphere 11, 81–99. doi:10.5194/tc-11-81-2017
Guo, W., Hao, W., and Xiao, W. (2024). Emerging perfluorinated chemical GenX: environmental and biological fates and risks. Environ. Health. doi:10.1021/envhealth.4c00164
Hamid, N., Junaid, M., Sultan, M., Yoganandham, S. T., and Chuan, O. M. (2024). The untold story of PFAS alternatives: insights into the occurrence, ecotoxicological impacts, and removal strategies in the aquatic environment. Water Res. 250, 121044. doi:10.1016/j.watres.2023.121044
Haque, F. L., Soerensen, A., Sköld, M., Awad, R., Spaan, M., Lauria, Z., et al. (2023). Per- and polyfluoroalkyl substances (PFAS) in white-tailed sea eagle eggs from Sweden: temporal trends (1969–2021), spatial variations, fluorine mass balance, and suspect screening. Environ. Sci. Process. and Impacts 25, 1549–1563. doi:10.1039/D3EM00141E
Hartz, W. F., Björnsdotter, M. K., Yeung, L. W. Y., Hodson, A., Thomas, E. R., Humby, J. D., et al. (2023). Levels and distribution profiles of per- and polyfluoroalkyl substances (PFAS) in a high arctic svalbard ice core. Sci. Total Environ. 871, 161830. doi:10.1016/j.scitotenv.2023.161830
Hartz, W. F., Björnsdotter, M. K., Yeung, L. W. Y., Humby, J. D., Eckhardt, S., Evangeliou, N., et al. (2024). Sources and seasonal variations of per- and polyfluoroalkyl substances (PFAS) in surface snow in the arctic. Environ. Sci. Technol. 58, 21817–21828. doi:10.1021/acs.est.4c08854
Huang, S., and Jaffé, P. R. (2019). Defluorination of perfluorooctanoic acid (PFOA) and perfluorooctane sulfonate (PFOS) by Acidimicrobium sp. strain A6. Environ. Sci. Technol. 53, 11410–11419. doi:10.1021/acs.est.9b04047
Interstate Technology and Regulatory Council (ITRC) (2023). Per- and Polyfluoroalkyl Substances (PFAS) technical and regulatory guidance document. Available online at: https://itrcweb.org/pfas-guidance/ (Accessed February 24, 2025).
Jansson, F. (2019). Occurrence of per- and polyfluorinated alkyl substances (PFAS), including ultra-short-chain compounds. Seasonal variation in rainwater from the Swedish west coast. Available online at: https://urn.kb.se/resolve?urn=urn:nbn:se:oru:diva-76989 (Accessed May 2, 2024).
Jin, B., Liu, H., Che, S., Gao, J., Yu, Y., Liu, J., et al. (2023). Substantial defluorination of polychlorofluorocarboxylic acids triggered by anaerobic microbial hydrolytic dechlorination. Nat. Water 1, 451–461. doi:10.1038/s44221-023-00077-6
Joerss, H., Xie, Z., Wagner, C. C., Von Appen, W.-J., Sunderland, E. M., and Ebinghaus, R. (2020). Transport of legacy perfluoroalkyl substances and the replacement compound HFPO-da through the atlantic gateway to the Arctic Ocean—is the arctic a sink or a source? Environ. Sci. Technol. 54, 9958–9967. doi:10.1021/acs.est.0c00228
Johansson, J. H., Salter, M. E., Acosta Navarro, J. C., Leck, C., Nilsson, E. D., and Cousins, I. T. (2019). Global transport of perfluoroalkyl acids via sea spray aerosol. Environ. Sci. Process. Impacts 21, 635–649. doi:10.1039/c8em00525g
Jones, B. M., Grosse, G., Farquharson, L. M., Roy-Léveillée, P., Veremeeva, A., Kanevskiy, M. Z., et al. (2022). Lake and drained lake basin systems in lowland permafrost regions. Nat. Rev. Earth Environ. 3, 85–98. doi:10.1038/s43017-021-00238-9
Jouanneau, W., Léandri-Breton, D.-J., Herzke, D., Moe, B., Nikiforov, V. A., Pallud, M., et al. (2023). Does contaminant exposure disrupt maternal hormones deposition? A study on per- and polyfluoroalkyl substances in an Arctic seabird. Sci. Total Environ. 868, 161413. doi:10.1016/j.scitotenv.2023.161413
Ju, X., Jin, Y., Sasaki, K., and Saito, N. (2008). Perfluorinated surfactants in surface, subsurface water and microlayer from Dalian coastal waters in China. Environ. Sci. Technol. 42, 3538–3542. doi:10.1021/es703006d
Kang, S. B., Wang, Z., Zhang, W., Kim, K.-Y., and Won, S. W. (2023). Removal of short- and long-chain PFAS from aquatic systems using electrostatic attraction of polyethylenimine-polyvinyl chloride electrospun nanofiber adsorbent. Sep. Purif. Technol. 326, 124853. doi:10.1016/j.seppur.2023.124853
Kannan, K., Koistinen, J., Beckmen, K., Evans, T., Gorzelany, J. F., Hansen, K. J., et al. (2001). Accumulation of perfluorooctane sulfonate in marine mammals. Environ. Sci. Technol. 35, 1593–1598. doi:10.1021/es001873w
Kärrman, A., Wang, T., Kallenborn, R., Langseter, A. M., Ræder, E. M., Lyche, J. L., et al. (2019). PFASs in the Nordic environment. Copenhagen: Nordic Council of Ministers, 515.
Kelly, B. C., Ikonomou, M. G., Blair, J. D., Surridge, B., Hoover, D., Grace, R., et al. (2009). Perfluoroalkyl contaminants in an arctic marine food web: trophic magnification and wildlife exposure. Environ. Sci. Technol. 43, 4037–4043. doi:10.1021/es9003894
European Union (2019). Regulation (EU) 2019/1021 of the European Parliament and of the council of 20 June 2019 on persistent organic Pollutants (consolidated version: 22 February 2021). Available online at: https://eur-lex.europa.eu/legal-content/EN/TXT/PDF/?uri=CELEX:02019R1021-20210222 (Accessed March 25, 2025).
Khan, B., Burgess, R. M., and Cantwell, M. G. (2023). Occurrence and bioaccumulation patterns of per- and polyfluoroalkyl substances (PFAS) in the marine environment. ACS Est. Water 3, 1243–1259. doi:10.1021/acsestwater.2c00296
Kim, S.-K., and Kannan, K. (2007). Perfluorinated acids in air, rain, snow, surface runoff, and lakes: relative importance of pathways to contamination of urban lakes. Environ. Sci. Technol. 41, 8328–8334. doi:10.1021/es072107t
Kim, Y., Pike, A., Gray, R., Faust, J., and Edmiston, P. (2023). Non-targeted identification and semi-quantitation of emerging per- and polyfluoroalkyl substances (PFAS) in US rainwater. Environ. Sci. Process. and Impacts 25, 1771–1787. doi:10.1039/D2EM00349J
Kirchgeorg, T., Dreyer, A., Gabrielli, P., Gabrieli, J., Thompson, L. G., Barbante, C., et al. (2016). Seasonal accumulation of persistent organic pollutants on a high altitude glacier in the Eastern Alps. Environ. Pollut. 218, 804–812. doi:10.1016/j.envpol.2016.08.004
Kwok, K. Y., Yamazaki, E., Yamashita, N., Taniyasu, S., Murphy, M. B., Horii, Y., et al. (2013). Transport of Perfluoroalkyl substances (PFAS) from an arctic glacier to downstream locations: implications for sources. Sci. Total Environ. 447, 46–55. doi:10.1016/j.scitotenv.2012.10.091
Lamon, L., von Waldow, H., MacLeod, M., Scheringer, M., Marcomini, A., and Hungerbühler, K. (2009). Modeling the global levels and distribution of polychlorinated biphenyls in air under a climate change scenario. Environ. Sci. Technol. 43, 5818–5824. doi:10.1021/es900438j
Léandri-Breton, D.-J., Jouanneau, W., Legagneux, P., Tarroux, A., Moe, B., Angelier, F., et al. (2024). Winter tracking data suggest that migratory seabirds transport per- and polyfluoroalkyl substances to their arctic nesting site. Environ. Sci. Technol. 58, 12909–12920. doi:10.1021/acs.est.4c02661
Letcher, R. J., Morris, A. D., Dyck, M., Sverko, E., Reiner, E. J., Blair, D. A. D., et al. (2018). Legacy and new halogenated persistent organic pollutants in polar bears from a contamination hotspot in the Arctic, Hudson Bay Canada. Sci. Total Environ. 610–611, 121–136. doi:10.1016/j.scitotenv.2017.08.035
Li, Z.-M., Roos, A., Serfass, T. L., Lee, C., and Kannan, K. (2024). Concentrations of 45 per- and polyfluoroalkyl substances in North American river otters (lontra canadensis) from West Virginia, USA. Environ. Sci. Technol. 58, 2089–2101. doi:10.1021/acs.est.3c09467
Liou, J. S.-C., Szostek, B., DeRito, C. M., and Madsen, E. L. (2010). Investigating the biodegradability of perfluorooctanoic acid. Chemosphere 80, 176–183. doi:10.1016/j.chemosphere.2010.03.009
Lohmann, R., Abass, K., Bonefeld-Jørgensen, E. C., Bossi, R., Dietz, R., Ferguson, S., et al. (2024). Cross-cutting studies of per- and polyfluorinated alkyl substances (PFAS) in Arctic wildlife and humans. Sci. Total Environ. 954, 176274. doi:10.1016/j.scitotenv.2024.176274
Lu, X., Ouyang, K., Su, G., Li, Q., Shi, B., Meng, J., et al. (2024). Environmental occurrence, bioaccumulation and human risks of emerging fluoroalkylether substances: insight into security of alternatives. Sci. Total Environ. 922, 171151. doi:10.1016/j.scitotenv.2024.171151
Ma, T., Ye, C., Wang, T., Li, X., and Luo, Y. (2022). Toxicity of per- and polyfluoroalkyl substances to aquatic invertebrates, planktons, and microorganisms. Int. J. Environ. Res. Public Health 19, 16729. doi:10.3390/ijerph192416729
MacInnis, J., Silva, A. O. D., Lehnherr, I., G. Muir, D. C., Pierre, K. A. S., Louis, V. L. S., et al. (2022). Investigation of perfluoroalkyl substances in proglacial rivers and permafrost seep in a high Arctic watershed. Environ. Sci. Process. and Impacts 24, 42–51. doi:10.1039/D1EM00349F
MacInnis, J. J., Lehnherr, I., Muir, D. C. G., Quinlan, R., and De Silva, A. O. (2019a). Characterization of perfluoroalkyl substances in sediment cores from High and Low Arctic lakes in Canada. Sci. Total Environ. 666, 414–422. doi:10.1016/j.scitotenv.2019.02.210
MacInnis, J. J., Lehnherr, I., Muir, D. C. G., St. Pierre, K. A., St. Louis, V. L., Spencer, C., et al. (2019b). Fate and transport of perfluoroalkyl substances from snowpacks into a lake in the high arctic of Canada. Environ. Sci. Technol. 53, 10753–10762. doi:10.1021/acs.est.9b03372
Magazine, S. (2024). PFAS legislation or not, brands must phase out “forever chemicals.”. Available online at: https://sustonmagazine.com/2024/04/03/pfas-legislation-or-not-brands-must-phase-out-forever-chemicals/(Accessed June 7, 2024).
Mahinroosta, R., and Senevirathna, L. (2020). A review of the emerging treatment technologies for PFAS contaminated soils. J. Environ. Manag. 255, 109896. doi:10.1016/j.jenvman.2019.109896
Manojkumar, Y., Pilli, S., Rao, P. V., and Tyagi, R. D. (2023). Sources, occurrence and toxic effects of emerging per- and polyfluoroalkyl substances (PFAS). Neurotoxicology Teratol. 97, 107174. doi:10.1016/j.ntt.2023.107174
Martinez Alvarez, L., Bolhuis, H., Mau, G. K., Kok-Gan, C., Sing, C. C., Mac Cormack, W., et al. (2022). Identification of key bacterial players during successful full-scale soil field bioremediation in Antarctica. Int. Biodeterior. and Biodegrad. 168, 105354. doi:10.1016/j.ibiod.2021.105354
Martinez-Varela, A., Cerro-Gálvez, E., Auladell, A., Sharma, S., Moran, M. A., Kiene, R. P., et al. (2021). Bacterial responses to background organic pollutants in the northeast subarctic Pacific Ocean. Environ. Microbiol. 23, 4532–4546. doi:10.1111/1462-2920.15646
McGovern, M., Warner, N. A., Borgå, K., Evenset, A., Carlsson, P., Skogsberg, E., et al. (2022). Is glacial meltwater a secondary source of legacy contaminants to arctic coastal food webs? Environ. Sci. Technol. 56, 6337–6348. doi:10.1021/acs.est.1c07062
Meegoda, J. N., Kewalramani, J. A., Li, B., and Marsh, R. W. (2020). A review of the applications, environmental release, and remediation technologies of per- and polyfluoroalkyl substances. Int. J. Environ. Res. Public Health 17, 8117. doi:10.3390/ijerph17218117
Merino, N., Qu, Y., Rula, A., Mahendra, S., and Hoffmann, M. R. (2016). Degradation and removal methods for perfluoroalkyl and polyfluoroalkyl substances in water. Environ. Eng. Sci. 33, 615–649. Available online at: https://home.liebertpub.com/ees.doi:10.1089/ees.2016.0233
Miner, K. R., Blais, J., Bogdal, C., Villa, S., Schwikowski, M., Pavlova, P., et al. (2017). Legacy organochlorine pollutants in glacial watersheds: a review. Environ. Sci. Process. Impacts 19, 1474–1483. doi:10.1039/C7EM00393E
Miner, K. R., Campbell, S., Gerbi, C., Liljedahl, A., Anderson, T., Perkins, L. B., et al. (2018). Organochlorine pollutants within a polythermal glacier in the interior eastern Alaska range. Water 10, 1157. doi:10.3390/w10091157
Miner, K. R., Clifford, H., Taruscio, T., Potocki, M., Solomon, G., Ritari, M., et al. (2021). Deposition of PFAS ‘forever chemicals’ on Mt. Everest. Sci. Total Environ. 759, 144421. doi:10.1016/j.scitotenv.2020.144421
Muir, D., Bossi, R., Carlsson, P., Evans, M., De Silva, A., Halsall, C., et al. (2019). Levels and trends of poly- and perfluoroalkyl substances in the Arctic environment – an update. Emerg. Contam. 5, 240–271. doi:10.1016/j.emcon.2019.06.002
Müller, V., Costa, L. C. A., Rondan, F. S., Matic, E., Mesko, M. F., Kindness, A., et al. (2023). Per and polyfluoroalkylated substances (PFAS) target and EOF analyses in ski wax, snowmelts, and soil from skiing areas. Environ. Sci. Process. Impacts 25, 1926–1936. doi:10.1039/D3EM00375B
Munoz, G., Budzinski, H., Babut, M., Lobry, J., Selleslagh, J., Tapie, N., et al. (2019). Temporal variations of perfluoroalkyl substances partitioning between surface water, suspended sediment, and biota in a macrotidal estuary. Chemosphere 233, 319–326. doi:10.1016/j.chemosphere.2019.05.281
Nguyen, T. M. H., Bräunig, J., Thompson, K., Thompson, J., Kabiri, S., Navarro, D. A., et al. (2020). Influences of chemical properties, soil properties, and solution pH on soil–water partitioning coefficients of per- and polyfluoroalkyl substances (PFASs). Environ. Sci. Technol. 54, 15883–15892. doi:10.1021/acs.est.0c05705
Nizovoy, P., Bellora, N., Haridas, S., Sun, H., Daum, C., Barry, K., et al. (2021). Unique genomic traits for cold adaptation in Naganishia vishniacii, a polyextremophile yeast isolated from Antarctica. FEMS Yeast Res. 21, foaa056. doi:10.1093/femsyr/foaa056
NOAA (2024). What is the cryosphere? Available online at: https://oceanservice.noaa.gov/facts/cryosphere.html (Accessed May 3, 2024).
OECD (2021). Reconciling terminology of the universe of per- and polyfluoroalkyl substances: recommendations and practical guidance. OECD Environment Health and Safety Publications.
OECD (2022). Synthesis report on understanding side-chain fluorinated polymers and their life cycle. OECD.
OECD (2024). Synthesis report on understanding Perfluoropolyethers (PFPEs) and their life cycle. OECD. Available online at: https://www.oecd.org/en/publications/synthesis-report-on-understanding-perfluoropolyethers-pfpes-and-their-life-cycle_99ee2d3e-en.html (Accessed February 21, 2025).
Okazoe, T. (2009). Overview on the history of organofluorine chemistry from the viewpoint of material industry. Proc. Jpn. Acad. Ser. B Phys. Biol. Sci. 85, 276–289. doi:10.2183/pjab.85.276
Olney, S., Jones, M., Rockwell, C., Collins, R. D., Bryant, J. D., and Occhialini, J. (2023). Influence of convective and stratiform precipitation types on per- and polyfluoroalkyl substance concentrations in rain. Sci. Total Environ. 890, 164051. doi:10.1016/j.scitotenv.2023.164051
Orellana, R., Macaya, C., Bravo, G., Dorochesi, F., Cumsille, A., Valencia, R., et al. (2018). Living at the frontiers of life: extremophiles in Chile and their potential for bioremediation. Front. Microbiol. 9, 2309. doi:10.3389/fmicb.2018.02309
Papale, M., Giannarelli, S., Francesconi, S., Di Marco, G., Mikkonen, A., Conte, A., et al. (2017). Enrichment, isolation and biodegradation potential of psychrotolerant polychlorinated-biphenyl degrading bacteria from the Kongsfjorden (Svalbard Islands, High Arctic Norway). Mar. Pollut. Bull. 114, 849–859. doi:10.1016/j.marpolbul.2016.11.011
Papale, M., Lo Giudice, A., Rappazzo, A. C., Azzaro, M., and Rizzo, C. (2022). A first glimpse on cold-adapted PCB-oxidizing bacteria in edmonson point lakes (northern victoria land, Antarctica). Water 14, 109. doi:10.3390/w14010109
Pearce, D. A. (2012). “Extremophiles in Antarctica: life at low temperatures,” in Adaption of microbial life to environmental extremes: novel research results and application. Editors H. Stan-Lotter, and S. Fendrihan (Vienna: Springer), 87–118.
Persaud, D., Criscitiello, A. S., Spencer, C., Lehnherr, I., Muir, D. C. G., Silva, A. O. D., et al. (2024). A 50 year record for perfluoroalkyl acids in the high arctic: implications for global and local transport. Environ. Sci. Process. Impacts 26, 1543–1555. doi:10.1039/D4EM00219A
Pickard, H. M., Criscitiello, A. S., Spencer, C., Sharp, M. J., Muir, D. C. G., De Silva, A. O., et al. (2018). Continuous non-marine inputs of per- and polyfluoroalkyl substances to the High Arctic: a multi-decadal temporal record. Atmos. Chem. Phys. 18, 5045–5058. doi:10.5194/acp-18-5045-2018
REACH (2024). REACH regulation - European commission. Available online at: https://environment.ec.europa.eu/topics/chemicals/reach-regulation_en (Accessed November 18, 2024).
Ren, J., Point, A. D., Baygi, S. F., Fernando, S., Hopke, P. K., Holsen, T. M., et al. (2022). Bioaccumulation of polyfluoroalkyl substances in the Lake Huron aquatic food web. Sci. Total Environ. 819, 152974. doi:10.1016/j.scitotenv.2022.152974
Renfrew, D., and Pearson, T. W. (2021). The social life of the “forever chemical.”. Environ. Soc. (N.Y.). 12, 146–163. doi:10.3167/ares.2021.120109
Reth, M., Berger, U., Broman, D., Cousins, I. T., Nilsson, E. D., and McLachlan, M. S. (2011). Water-to-air transfer of perfluorinated carboxylates and sulfonates in a sea spray simulator. Environ. Chem. 8, 381–388. doi:10.1071/EN11007
Rigét, F., Bossi, R., Sonne, C., Vorkamp, K., and Dietz, R. (2013). Trends of perfluorochemicals in Greenland ringed seals and polar bears: indications of shifts to decreasing trends. Chemosphere 93, 1607–1614. doi:10.1016/j.chemosphere.2013.08.015
Roberts, K. E., Lamoureux, S. F., Kyser, T. K., Muir, D. C. G., Lafrenière, M. J., Iqaluk, D., et al. (2017). Climate and permafrost effects on the chemistry and ecosystems of High Arctic Lakes. Sci. Rep. 7, 13292. doi:10.1038/s41598-017-13658-9
Ross, I., McDonough, J., Miles, J., Storch, P., Thelakkat Kochunarayanan, P., Kalve, E., et al. (2018). A review of emerging technologies for remediation of PFASs. Remediat. J. 28, 101–126. doi:10.1002/rem.21553
Routti, H., Aars, J., Fuglei, E., Hanssen, L., Lone, K., Polder, A., et al. (2017). Emission changes dwarf the influence of feeding habits on temporal trends of per- and polyfluoroalkyl substances in two arctic top predators. Environ. Sci. Technol. 51, 11996–12006. doi:10.1021/acs.est.7b03585
Saritha, V. K., Krishnan, K. P., and Mohan, M. (2023). Perfluorooctanoic acid in the sediment matrices of Arctic fjords, Svalbard. Mar. Pollut. Bull. 192, 115061. doi:10.1016/j.marpolbul.2023.115061
Schrenk, D., Bignami, M., Bodin, L., Chipman, J. K., del Mazo, J., Grasl-Kraupp, B., et al. (2020). Risk to human health related to the presence of perfluoroalkyl substances in food. EFSA J. 18, e06223. doi:10.2903/j.efsa.2020.6223
Schuur, E. A. G., McGuire, A. D., Schädel, C., Grosse, G., Harden, J. W., Hayes, D. J., et al. (2015). Climate change and the permafrost carbon feedback. Nature 520, 171–179. doi:10.1038/nature14338
Schwanz, T. G., Llorca, M., Farré, M., and Barceló, D. (2016). Perfluoroalkyl substances assessment in drinking waters from Brazil, France and Spain. Sci. Total Environ. 539, 143–152. doi:10.1016/j.scitotenv.2015.08.034
Senevirathna, S. T. M. L. D., Krishna, K. C. B., Mahinroosta, R., and Sathasivan, A. (2022). Comparative characterization of microbial communities that inhabit PFAS-rich contaminated sites: a case-control study. J. Hazard. Mater. 423, 126941. doi:10.1016/j.jhazmat.2021.126941
Sha, B., Johansson, J. H., Tunved, P., Bohlin-Nizzetto, P., Cousins, I. T., and Salter, M. E. (2022). Sea spray aerosol (SSA) as a source of perfluoroalkyl acids (PFAAs) to the atmosphere: field evidence from long-term air monitoring. Environ. Sci. Technol. 56, 228–238. doi:10.1021/acs.est.1c04277
Shan, G., Xiang, Q., Feng, X., Wu, W., Yang, L., and Zhu, L. (2021). Occurrence and sources of per- and polyfluoroalkyl substances in the ice-melting lakes of Larsemann Hills, East Antarctica. Sci. Total Environ. 781, 146747. doi:10.1016/j.scitotenv.2021.146747
Sherman-Bertinetti, S. L., Gruber, K. J., and Remucal, C. K. (2024). Preferential partitioning of per- and polyfluoroalkyl substances in freshwater ice. Environ. Sci. Technol. 58, 15214–15223. doi:10.1021/acs.est.4c04636
Shi, Y.-L., Pan, Y.-Y., Liang, L.-N., and Cai, Y.-Q. (2015). An on-line solid phase extraction–liquid chromatography tandem mass spectrometry method for the determination of perfluoroalkyl substances in the Antarctic ice core samples. Chin. Chem. Lett. 26, 1073–1078. doi:10.1016/j.cclet.2015.05.038
Singh, K., Kumar, N., Kumar Yadav, A., Singh, R., and Kumar, K. (2023). Per-and polyfluoroalkyl substances (PFAS) as a health hazard: current state of knowledge and strategies in environmental settings across Asia and future perspectives. Chem. Eng. J. 475, 145064. doi:10.1016/j.cej.2023.145064
Skaar, J. S., Ræder, E. M., Lyche, J. L., Ahrens, L., and Kallenborn, R. (2019). Elucidation of contamination sources for poly- and perfluoroalkyl substances (PFASs) on Svalbard (Norwegian Arctic). Environ. Sci. Pollut. Res. 26, 7356–7363. doi:10.1007/s11356-018-2162-4
Smorada, C. M., Sima, M. W., and Jaffé, P. R. (2024). Bacterial degradation of perfluoroalkyl acids. Curr. Opin. Biotechnol. 88, 103170. doi:10.1016/j.copbio.2024.103170
Sonne, C., Jenssen, B. M., Rinklebe, J., Lam, S. S., Hansen, M., Bossi, R., et al. (2023). EU need to protect its environment from toxic per- and polyfluoroalkyl substances. Sci. Total Environ. 876, 162770. doi:10.1016/j.scitotenv.2023.162770
Stemmler, I., and Lammel, G. (2010). Pathways of PFOA to the Arctic: variabilities and contributions of oceanic currents and atmospheric transport and chemistry sources. Atmos. Chem. Phys. 10, 9965–9980. doi:10.5194/acp-10-9965-2010
Sulbaek Andersen, M. P., Nielsen, O. J., Hurley, M. D., Ball, J. C., Wallington, T. J., Ellis, D. A., et al. (2005). Atmospheric chemistry of 4:2 fluorotelomer alcohol (n-C4F9CH2CH2OH): products and mechanism of Cl atom initiated oxidation in the presence of NOx. J. Phys. Chem. A 109, 1849–1856. doi:10.1021/jp045672g
Sun, M., Arevalo, E., Strynar, M., Lindstrom, A., Richardson, M., Kearns, B., et al. (2016). Legacy and emerging perfluoroalkyl substances are important drinking water contaminants in the cape fear river watershed of North Carolina. Environ. Sci. Technol. Lett. 3, 415–419. doi:10.1021/acs.estlett.6b00398
Tartu, S., Bourgeon, S., Aars, J., Andersen, M., Lone, K., Jenssen, B. M., et al. (2017). Diet and metabolic state are the main factors determining concentrations of perfluoroalkyl substances in female polar bears from Svalbard. Environ. Pollut. 229, 146–158. doi:10.1016/j.envpol.2017.04.100
Teymourian, T., Teymoorian, T., Kowsari, E., and Ramakrishna, S. (2021). A review of emerging PFAS contaminants: sources, fate, health risks, and a comprehensive assortment of recent sorbents for PFAS treatment by evaluating their mechanism. Res. Chem. Intermed. 47, 4879–4914. doi:10.1007/s11164-021-04603-7
Thomas, D. N., and Dieckmann, G. S. (2002). Antarctic Sea ice--a habitat for extremophiles. Science 295, 641–644. doi:10.1126/science.1063391
Tomy, G. T., Budakowski, W., Halldorson, T., Helm, P. A., Stern, G. A., Friesen, K., et al. (2004). Fluorinated organic compounds in an eastern arctic marine food web. Environ. Sci. Technol. 38, 6475–6481. doi:10.1021/es049620g
UNEP (2022). National environmental action Plan 2022-2030. Available online at: https://leap.unep.org/en/countries/lk/national-legislation/national-environmental-action-plan-2022-2030 (Accessed July 28, 2024).
U.S. EPA (2022). Drinking water health advisory: hexafluoropropylene oxide (HFPO) dimer acid (CASRN 13252-13-6) and HFPO dimer acid ammonium salt (CASRN 62037-80-3), also known as “GenX chemicals.” EPA/822/R-22/005.
US EPA (2024). PFAS national primary drinking water regulation. Federal Register, 89(82), 31592–31681. Available online at: https://www.federalregister.gov/documents/2024/04/26/2024-07773/pfas-national-primary-drinking-water-regulation (Accessed February 20, 2025).
US EPAO (2019). Addition of certain PFAS to the TRI by the national Defense authorization act. Available online at: https://www.epa.gov/toxics-release-inventory-tri-program/addition-certain-pfas-tri-national-defense-authorization-act (Accessed February 24, 2025).
US EPAO (2021). Per- and polyfluoroalkyl substances (PFAS). Available online at: https://www.epa.gov/sdwa/and-polyfluoroalkyl-substances-pfas (Accessed February 20, 2025).
US EPAO (2025). EPA adds nine additional PFAS to the toxics release inventory. Available online at: https://www.epa.gov/newsreleases/epa-adds-nine-additional-pfas-toxics-release-inventory (Accessed February 24, 2025).
Valdersnes, S., Nilsen, B. M., Breivik, J. F., Borge, A., and Maage, A. (2017). Geographical trends of PFAS in cod livers along the Norwegian coast. PLoS ONE 12, e0177947. doi:10.1371/journal.pone.0177947
Van de Vijver, K. I., Hoff, P. T., Das, K., Van Dongen, W., Esmans, E. L., Jauniaux, T., et al. (2003). Perfluorinated chemicals infiltrate ocean waters: link between exposure levels and stable isotope ratios in marine mammals. Environ. Sci. Technol. 37, 5545–5550. doi:10.1021/es0345975
Villanger, G. D., Kovacs, K. M., Lydersen, C., Haug, L. S., Sabaredzovic, A., Jenssen, B. M., et al. (2020). Perfluoroalkyl substances (PFASs) in white whales (Delphinapterus leucas) from Svalbard – a comparison of concentrations in plasma sampled 15 years apart. Environ. Pollut. 263, 114497. doi:10.1016/j.envpol.2020.114497
von Appen, W.-J. (2018). The expedition PS114 of the research vessel POLARSTERN to the Fram Strait in 2018. Bremerhaven, Germany: Berichte zur Polar- und Meeresforschung.
Vonk, J. E., Tank, S. E., Bowden, W. B., Laurion, I., Vincent, W. F., Alekseychik, P., et al. (2015). Reviews and syntheses: effects of permafrost thaw on Arctic aquatic ecosystems. Biogeosciences 12, 7129–7167. doi:10.5194/bg-12-7129-2015
Wackett, L. P., and Robinson, S. L. (2020). The ever-expanding limits of enzyme catalysis and biodegradation: polyaromatic, polychlorinated, polyfluorinated, and polymeric compounds. Biochem. J. 477, 2875–2891. doi:10.1042/BCJ20190720
Wang, X., Chen, M., Gong, P., and Wang, C. (2019). Perfluorinated alkyl substances in snow as an atmospheric tracer for tracking the interactions between westerly winds and the Indian Monsoon over western China. Environ. Int. 124, 294–301. doi:10.1016/j.envint.2018.12.057
Wang, X., Halsall, C., Codling, G., Xie, Z., Xu, B., Zhao, Z., et al. (2014). Accumulation of perfluoroalkyl compounds in Tibetan mountain snow: temporal patterns from 1980 to 2010. Environ. Sci. Technol. 48, 173–181. doi:10.1021/es4044775
Wang, Y., Li, X., Zheng, Z., Shi, Y., and Cai, Y. (2021a). Chlorinated polyfluoroalkyl ether sulfonic acids in fish, dust, drinking water and human serum: from external exposure to internal doses. Environ. Int. 157, 106820. doi:10.1016/j.envint.2021.106820
Wang, Z., Buser, A. M., Cousins, I. T., Demattio, S., Drost, W., Johansson, O., et al. (2021b). A new OECD definition for per- and polyfluoroalkyl substances. Environ. Sci. Technol. 55, 15575–15578. doi:10.1021/acs.est.1c06896
Wang, Z., Cousins, I. T., Scheringer, M., and Hungerbuehler, K. (2015a). Hazard assessment of fluorinated alternatives to long-chain perfluoroalkyl acids (PFAAs) and their precursors: status quo, ongoing challenges and possible solutions. Environ. Int. 75, 172–179. doi:10.1016/j.envint.2014.11.013
Wang, Z., Xie, Z., Mi, W., Möller, A., Wolschke, H., and Ebinghaus, R. (2015b). Neutral poly/per-fluoroalkyl substances in air from the atlantic to the Southern Ocean and in antarctic snow. Environ. Sci. Technol. 49, 7770–7775. doi:10.1021/acs.est.5b00920
Wania, F. (2007). A global mass balance analysis of the source of perfluorocarboxylic acids in the Arctic Ocean. Environ. Sci. Technol. 41, 4529–4535. doi:10.1021/es070124c
Wee, S. Y., and Aris, A. Z. (2023). Revisiting the “forever chemicals”. PFOA PFOS Expo. Drink. water. npj Clean Water 6, 1–16. doi:10.1038/s41545-023-00274-6
Wild, S., McLagan, D., Schlabach, M., Bossi, R., Hawker, D., Cropp, R., et al. (2015). An antarctic research station as a source of brominated and perfluorinated persistent organic pollutants to the local environment. Environ. Sci. Technol. 49, 103–112. doi:10.1021/es5048232
Xie, Z., and Kallenborn, R. (2023). Legacy and emerging per- and poly-fluoroalkyl substances in polar regions. Curr. Opin. Green Sustain. Chem. 42, 100840. doi:10.1016/j.cogsc.2023.100840
Xie, Z., Wang, Z., Mi, W., Möller, A., Wolschke, H., and Ebinghaus, R. (2015). Neutral poly-/perfluoroalkyl substances in air and snow from the arctic. Sci. Rep. 5, 8912. doi:10.1038/srep08912
Xin, X., Kim, J., Ashley, D. C., and Huang, C.-H. (2023). Degradation and defluorination of per- and polyfluoroalkyl substances by direct photolysis at 222 nm. ACS Est. Water 3, 2776–2785. doi:10.1021/acsestwater.3c00274
Yamashita, N., Taniyasu, S., Petrick, G., Wei, S., Gamo, T., Lam, P. K. S., et al. (2008). Perfluorinated acids as novel chemical tracers of global circulation of ocean waters. Chemosphere 70, 1247–1255. doi:10.1016/j.chemosphere.2007.07.079
Yeung, L. W. Y., Dassuncao, C., Mabury, S., Sunderland, E. M., Zhang, X., and Lohmann, R. (2017). Vertical profiles, sources, and transport of PFASs in the Arctic Ocean. Environ. Sci. Technol. 51, 6735–6744. doi:10.1021/acs.est.7b00788
Yi, L., Peng, Q., Liu, D., Zhou, L., Tang, C., Zhou, Y., et al. (2019). Enhanced degradation of perfluorooctanoic acid by a genome shuffling-modified Pseudomonas parafulva YAB-1. Environ. Technol. 40, 3153–3161. doi:10.1080/09593330.2018.1466918
Yin, S., and Villagrán, D. (2022). Design of nanomaterials for the removal of per- and poly-fluoroalkyl substances (PFAS) in water: strategies, mechanisms, challenges, and opportunities. Sci. Total Environ. 831, 154939. doi:10.1016/j.scitotenv.2022.154939
Young, C. J., Furdui, V. I., Franklin, J., Koerner, R. M., Muir, D. C. G., and Mabury, S. A. (2007). Perfluorinated acids in arctic snow: new evidence for atmospheric formation. Environ. Sci. Technol. 41, 3455–3461. doi:10.1021/es0626234
Yu, Y., Zhang, K., Li, Z., Ren, C., Chen, J., Lin, Y.-H., et al. (2020). Microbial cleavage of C–F bonds in two C6 per- and polyfluorinated compounds via reductive defluorination. Environ. Sci. Technol. 54, 14393–14402. doi:10.1021/acs.est.0c04483
J. Zalasiewicz, C. N. Waters, M. Williams, and C. P. Summerhayes (2019). The Anthropocene as a geological time unit: a guide to the scientific evidence and current debate (Cambridge: Cambridge University Press).
Zhang, D., Li, J., Li, X., Wang, M., Zhong, Y., Chen, G., et al. (2023). Phytoremediation of fluoroalkylethers (ether-PFASs): a review on bioaccumulation and ecotoxilogical effects. Sci. Total Environ. 865, 161260. doi:10.1016/j.scitotenv.2022.161260
Zhang, X., Lohmann, R., and Sunderland, E. M. (2019). Poly- and perfluoroalkyl substances in seawater and plankton from the northwestern atlantic margin. Environ. Sci. Technol. 53, 12348–12356. doi:10.1021/acs.est.9b03230
Zhang, X., Zhang, Y., Dassuncao, C., Lohmann, R., and Sunderland, E. M. (2017). North Atlantic Deep Water formation inhibits high Arctic contamination by continental perfluorooctane sulfonate discharges. Glob. Biogeochem. Cycles 31, 1332–1343. doi:10.1002/2017GB005624
Zhang, Y., Qv, Z., Wang, J., Yang, Y., Chen, X., Wang, J., et al. (2022a). Natural biofilm as a potential integrative sample for evaluating the contamination and impacts of PFAS on aquatic ecosystems. Water Res. 215, 118233. doi:10.1016/j.watres.2022.118233
Zhang, Z., Sarkar, D., Biswas, J. K., and Datta, R. (2022b). Biodegradation of per- and polyfluoroalkyl substances (PFAS): a review. Bioresour. Technol. 344, 126223. doi:10.1016/j.biortech.2021.126223
Zhao, Z., Xie, Z., Möller, A., Sturm, R., Tang, J., Zhang, G., et al. (2012). Distribution and long-range transport of polyfluoroalkyl substances in the Arctic, Atlantic Ocean and Antarctic coast. Environ. Pollut. 170, 71–77. doi:10.1016/j.envpol.2012.06.004
Zhou, Y., Wang, X., Chen, M., Fu, J., Zhu, T., Wang, C., et al. (2024a). Proglacial river sediments are a substantial sink of perfluoroalkyl substances released by glacial meltwater. Commun. Earth Environ. 5, 68–11. doi:10.1038/s43247-024-01223-y
Keywords: PFAS, cryosphere, bioaccumulation, biomagnification, bioremediation, PFAS-phaseout, transformation
Citation: Arulananthan A, Vilhelmsson OÞ, Karsten U, Grossart H-P, Sigurbjörnsdóttir A, Rolfsson Ó, Joerss H and Scholz B (2025) Per- and polyfluoroalkyl substances (PFAS) in the cryosphere – occurrence, organismic accumulation, ecotoxicological impacts, transformation, and management strategies. Front. Environ. Sci. 13:1559941. doi: 10.3389/fenvs.2025.1559941
Received: 13 January 2025; Accepted: 17 March 2025;
Published: 08 April 2025.
Edited by:
Nsikak U. Benson, Topfaith University, NigeriaReviewed by:
Manavi Yadav, New Jersey Institute of Technology, United StatesDuwage Charitha Perera, New Jersey Institute of Technology, United States
Copyright © 2025 Arulananthan, Vilhelmsson, Karsten, Grossart, Sigurbjörnsdóttir, Rolfsson, Joerss and Scholz. This is an open-access article distributed under the terms of the Creative Commons Attribution License (CC BY). The use, distribution or reproduction in other forums is permitted, provided the original author(s) and the copyright owner(s) are credited and that the original publication in this journal is cited, in accordance with accepted academic practice. No use, distribution or reproduction is permitted which does not comply with these terms.
*Correspondence: Ashani Arulananthan, YXNoYW5pQHVuYWsuaXM=; Bettina Scholz, YmV0dGluYUBiaW9wb2wuaXM=