Methyl-Donor Micronutrient for Gestating Sows: Effects on Gut Microbiota and Metabolome in Offspring Piglets
- 1Key Laboratory of Animal Nutrition in Jiangxi Province, Jiangxi Agricultural University, Nanchang, China
- 2Key Innovation Center for Industry-Education Integration of High-Quality and Safety Livestock Production in Jiangxi Province, Jiangxi Agricultural University, Nanchang, China
This study aimed to investigate the effects of maternal methyl-donor micronutrient supplementation during gestation on gut microbiota and the fecal metabolic profile in offspring piglets. Forty-three Duroc × Erhualian gilts were assigned to two dietary groups during gestation: control diet (CON) and CON diet supplemented with MET (folic acid, methionine, choline, vitamin B6, and vitamin B12). The body weights of offspring piglets were recorded at birth and weaning. Besides this, fresh fecal samples of offspring piglets were collected at 7, 14, and 21 days. The gut microbiota composition, metabolic profile, and short-chain fatty acid (SCFA) profiles in the fecal samples were determined using 16S rDNA sequencing, liquid chromatography-mass spectrometry metabolomics, and gas chromatography methods, respectively. The results showed that maternal methyl-donor micronutrient supplementation increased the microbiota diversity and uniformity in feces of offspring piglets as indicated by increased Shannon and Simpson indices at 7 days, and greater Simpson, ACE, Chao1 and observed species indices at 21 days. Specifically, at the phylum level, the relative abundance of Firmicutes and the Firmicutes to Bacteroidetes ratio were elevated by maternal treatment. At the genus level, the relative abundance of SCFA-producing Dialister, Megasphaera, and Turicibacter, and lactate-producing Sharpea as well as Akkermansia, Weissella, and Pediococcus were increased in the MET group. The metabolic analyses show that maternal methyl-donor micronutrient addition increased the concentrations of individual and total SCFAs of 21-day piglets and increased metabolism mainly involving amino acids, pyrimidine, and purine biosynthesis. Collectively, maternal methyl-donor micronutrient addition altered gut microbiota and the fecal metabolic profile, resulting in an improved weaning weight of offspring piglets.
Introduction
The gut microbiota and fecal metabolic profile of neonates are closely related to immunity, disease, growth, and development. The colonization of microbial communities in the intestines is a key process in infant development (1); the intestinal microbial establishment is mainly determined and easily affected by diet, mother-to-child transmission, and the environment (2). The indigestible nutrients that are metabolized by bacteria in the neonatal intestines improve energy harvesting for growth but expose developing mammals to a variety of chemicals (3). The stability of gut microbiota systems can adapt to such environmental fluctuations. Therefore, a dysbiosis in the gut microbial community not only results in higher disease risk, but also causes short- and long-lasting adverse effects on neonates' health. Diet is an important determinant of maternal offspring's microbial communities and health outcomes. Maternal nutritional status has a great influence on fetal development, pregnancy outcome, and offspring disease development (4–6). Therefore, a specific diet plan for pregnant women may be a cost-effective intervention to promote intestinal microbiota colonization of offspring.
Methyl-donor micronutrients (MET), including folate, choline, betaine, methionine, cobalamin, pyridoxine, and so forth, are demonstrated to participate in the synthesis of nucleotides, proteins, and lipids via epigenetic mechanisms (7); meanwhile, the epigenetic mechanisms also modify the metabolome (8). However, the intergenerational effect of MET on the physiological metabolism of offspring in the early stage is hardly documented. Maternal diet during pregnancy may have a significant impact on the establishment of the neonatal microbiota, and may play a role in infant development (9). Additionally, there is increasing evidence that microbiota-derived metabolites are key factors regulating animal metabolism, growth, and development (10). Maternal-MET supplementation induces a specific intestinal microenvironment, limiting pathobiont colonization [such as adherent-invasive Escherichia coli (AIEC)] of the offspring gut (11). Conversely, other studies report that maternal-MET supplementation or deficiency leads to an increase in the sensitivity to colitis in the offspring (12, 13), suggesting the central role of MET in the intestinal microenvironment. Therefore, differences in the metabolome of control and MET offspring in the early stages after birth need to be further studied.
In our previous study, we found no differences in the reproduction performance of sows between CON and MET (number of total born, born alive, and weaned piglets per litter, etc.). However, maternal MET supplementation during pregnancy promotes skeletal muscle differentiation and maturity in newborn and weaning pigs and improves the growth performance of the offspring (14). This means that some changes in the suckling piglets may be important for growth and development. Therefore, this study was conducted to test the hypothesis that maternal methyl-donor micronutrient supplementation during gestation could beneficially regulate the gut microbiota and fecal metabolic profile and enhance the growth performance of offspring piglets.
Materials and Methods
Ethics Statement
This study was conducted under the Chinese guidelines for animal welfare. All animal experiments were approved by the Animal Care and Use Committee of Jiangxi Agricultural University (Ethic Approval Code: JXAUA01).
Animals and Experimental Design
A total of 43 crossbred gilts (Duroc × Erhualian, body weight: 102.8 ± 6.3 kg) were artificially inseminated and then allotted by body weight to two dietary treatments. The two experimental diets were supplemented with or without methyl-donor micronutrients in the basal diet. There were 21 and 22 gilts in the control group (CON group) and Methyl-donor micronutrients group (MET group), respectively. The MET-supplemented diet contained 4,700 mg kg−1 methionine (CJ BIO, Malaysia, purity ≥99%), 16.3 mg kg−1 folic acid (Sigma-Aldrich, St. Louis, MO, USA, purity ≥97%), 2,230 mg kg−1 choline (NB GROUP, Shangdong, China, purity = 60%), 0.15 mg kg−1 vitamin B12 (Sigma-Aldrich, St. Louis, MO, USA, purity ≥98%), and 1,180 mg kg−1 vitamin B6 (Jiangxi Tianxin Pharmaceutical, Jiangxi, China, purity ≥98%). The dosage of methyl-donor micronutrients was added according to previous studies (15–18). Dietary treatment started at the last insemination and lasted until parturition. Ingredients and composition of pregnant gilt diets are show in Supplementary Table 1. During gestation, sows were fed 2.28 kg/day during days 1–80, 2.40 kg/day during days 80–90, and 3.00 kg/day of diet from day 91 until farrowing. Sows were fed discretely twice daily at 0800 and 1,400 h with 50% of the daily ration during each feeding. On day 110 of gestation, sows were transferred to farrowing pens. After parturition, all sows received a standard lactation diet (Supplementary Table 2) three times per day (i.e., 0800, 1,200, and 1,500 h). Piglets were weaned at 24 days. All animals were free to drink water. The experiment began with 54 gilts, 27 gilts per treatment. Pregnancy was confirmed by ultrasonic examination 30 days post-mating; 11 gilts were eliminated due to failure of pregnancy.
Data and Sample Collection
Sample Collection
After parturition, body weights (BW) were measured at birth and weaning (24 days). Six litters per group were selected, and one median-birth-weight piglet from each litter were sampled for feces collection at days 7, 14, and 21 and then snap-frozen in liquid nitrogen and stored at −80°C for gut microbiota, short-chain fatty acid (SCFA), and metabolomics analyses. Fecal 16S rDNA sequencing and liquid chromatography-mass spectrometry (LC-MS) metabolomics were performed according to the manufacturer's instructions (Shanghai Applied Protein Technology, Shanghai, China).
Serum S-Adenosylmethionine and Homocysteine
Serum from newborn and weaning offspring was analyzed for S-adenosylmethionine (SAM) and homocysteine (Hcy) using the enzyme-linked immunosorbent assay (ELISA) kits purchased from MLBIO (Shanghai, China).
Fecal DNA Extraction and 16S rDNA Gene Amplicon Sequencing Analysis
The total genomic DNA was extracted from fecal samples with the Cetyltrimethylammonium Bromide (CTAB) method. DNA concentration and purity were monitored on an agarose gel. The DNA was then diluted accordingly using sterile water. The bacterial 16S rDNA gene of various regions (3–4) was amplified by polymerase chain reaction (PCR) using a specific primer (Uni340F: 5′-CCTAYGGGRBGCASCAG-3′, Uni806R: 5′-GGACTACNNGGGTATCTAAT-3′). The PCR product was detected with 2% agarose gel and purified with the Qiagen Gel Extraction Kit (Qiagen, Germany) following the manufacturer's instructions. The library was generated by TruSeq® DNA PCR-Free Sample Preparation Kit (Illumina, USA) and quantified using a Qubit @ 2.0 Fluorometer (Thermo Scientific). Finally, 250 base pair paired-end sequencing was performed using the Illumina HiSeq 2500 platform.
Sequencing data were analyzed using the quantitative insights into microbial ecology (QIIME) (V1.9.1, http://qiime.org/scripts/split_libraries_fastq.html). Paired-end reads were merged using Fast Length Adjustment of Short reads (FLASH, V1.2.7, http://ccb.jhu.edu/software/FLASH/). The clean data were obtained by specific splice and filtering of the raw sequence data. Sequences with ≥97% similarity were assigned to the same operational taxonomic units (OTUs) and the OTU was screened for further annotation; analysis was performed using Uparse software (Uparse v7.0.1001, http://drive5.com/uparse/). The abundance information of OTUs was normalized using the serial number standard corresponding to the sample with the least sequence and both alpha (observed species, Chao1, Shannon, Simpson, ACE) and beta diversity (principal coordinate analysis (PCoA) and unweighted pair-group method with arithmetic means) were performed based on the normalized data of OTU abundance information. The alpha and beta diversity were calculated with QIIME and displayed with R software (Version 2.15.3). PCoA was based on unweighted UniFrac distances using the WGCNA, stat, and ggplot2 packages in R. To determine the significance test of community structure differences between groups, permutational multivariate analysis of variance (PERMANOVA, Adonis procedure with 999 permutations) was performed in R to calculate P-values. Additionally, to further explore the differences in the community structure (phylum and genus) between the two groups of samples, the linear discriminant analysis (LDA) effect size (LEfSe) method was used to compare the differences in the taxonomic levels; the LDA score was set at 2.0 for a biomarker. In LEfSe analysis, the non-parametric factorial Kruskal–Wallis (KW) sum-rank test was used to detect all species with significant differential abundance, and the Wilcoxon rank-sum test was used to investigate biological consistency among subclasses.
Concentration of SCFA in the Fecal
Approximately 1 g of fecal samples were weighed, diluted with 2 mL of ultra-pure water and centrifuged at 12,000 g (4°C) for 15 min to obtain a supernatant. Then 25% of metaphosphoric acid solution was added in a ratio of 9:1 before centrifugation at 3,000 g for 10 min. The supernatant was aspirated with a syringe and filtered through a 0.45 mm filter membrane. Acetic acid, propionic acid, isobutyric acid, butyric acid, isovaleric acid, valeric acid, and total SCFA were determined using capillary column gas chromatography (Shimadzu Gas Chromatography 2014, Japan; capillary column length was 30 m, inner diameter was 0.25 mm, and film thickness was 0.25 μm), column temperature = 120°C, injector temperature = 220°C, detector temperature = 250°C, and each injection volume was 1 μL.
The standard substances of SCFAs are as follows: acetic acid (A116165, Shanghai Aladdin Bio-Chem Technology Co., LTD, Shanghai, China), propionic acid (P110443, Aladdin), isobutyric acid (I103521, Aladdin), butyric acid (B110439, Aladdin), isovaleric acid (I108280, Aladdin), and valeric acid (V108269, Aladdin).
Fecal Untargeted Metabolomic Analysis
Aliquots of 100 mg of fecal samples were homogenized with 200 μL of ultra-pure water and mixed with 800 L methanol/acetonitrile (1:1, v/v) to remove the protein. After centrifugation (14,000 g, 20 min, 4°C), the supernatant was collected for analysis.
LC-MS Analysis and Data Processing
Analyses were performed using liquid chromatography (1,290 infinity liquid, Agilent) coupled with quadrupole time-of-flight (Triple TOF 6600, AB Sciex). For hydrophilic interaction liquid chromatography (HILIC) separation, samples were analyzed using a 2.1 × 100 mm ACQUIY UPLC BEH Amide 1.7 μm column (Waters, Ireland). Chromatographic conditions and Q-TOF mass spectrometry conditions were as outlined by Liu et al. (19), electrospray ionization (ESI) positive mode was used for detection.
The raw MS data were converted to MzXML format using ProteoWizard MSConvert and processed using the XCMS online package (version 1.20.1) for further processing. Metabolites were identified by accuracy mass (<25 ppm) and secondary spectral matching to retrieve a self-built database from the laboratory (Shanghai Applied Protein Technology). After data preprocessing by Pareto-scaling, multidimensional statistical analysis [orthogonal partial least squares discriminant analysis (OPLS-DA)] and Student's t-test analysis were performed. Significantly different metabolites were identified based on the combination of a statistically significant threshold of variable influence on projection (VIP) values obtained from the OPLS-DA model and two-tailed Student's t-test (p-value) from the raw data. VIP > 1.00 and P < 0.05 were considered as significantly different metabolites. VIP > 1.00 and 0.05 < P < 0.10 were regarded as differential metabolites.
Statistical Analysis
Data were tested for normality using the Shapiro–Wilk test before statistical analysis. The growth performance, SCFA, and serum methyl metabolite profile between CON and MET piglets was assessed by independent-samples t-test. The individual sow was considered as the experimental unit for growth performance, one piglet per pen was used as the experimental unit for SCFA and methyl metabolite profile and microbial and metabolite analysis. Statistical analysis was performed on SPSS 24.0 software (SPSS Inc.). Correlations between altered metabolites screened from fecal metabonomic and perturbed gut microbe genera screened from 16S rDNA gene sequencing analysis were assessed by Spearman's correlation analysis. GraphPad Prism 8.0 (San Diego, CA, USA) was used to plot the images. Data are shown as means ± SEM. A value of P < 0.05 was considered statistical significance.
Results
Growth Performance and Serum SAM and Hcy Concentrations of Offspring Piglets
There was no statistical difference in offspring birth weight between dietary treatments (P > 0.05). However, the body weight at weaning was greater for piglets from sows fed the MET vs. CON diet (P < 0.05) (Figure 1).
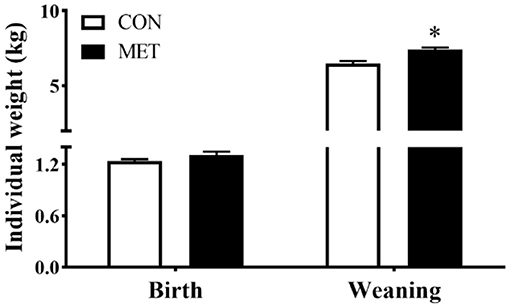
Figure 1. Effect of maternal methyl-donor micronutrient supplementation during gestation on birth weight and weaning weight of offspring piglets (n = 21). No difference was observed for litter size of lactating sows between treatments [data not shown, see reference (14)]. The litter of each sow was considered as the experimental unit to calculate the individual weight of piglets at birth and weanling. CON, the control group; MET, the methyl-donor micronutrients group. *P < 0.05. Values are expressed as means ± standard error of mean (SEM).
Maternal MET supplementation during gestation decreased serum Hcy concentration in the offspring at birth (P = 0.089, Table 1). Likewise, there was a significantly lower concentration of serum Hcy in the weaning offspring of the MET group compared with the CON group (P < 0.05). Moreover, the serum SAM concentration in piglets at birth and weaning was increased in the MET group (P = 0.061, P < 0.05, respectively).
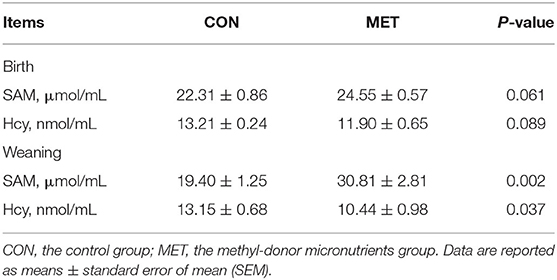
Table 1. Effect of maternal MET supplementation during gestation on the concentration of S-adenosylmethionine (SAM) and homocysteine (Hcy) in the serum of newborn and weaning piglets (n = 6).
Fecal Microbiota Diversity
The alpha diversity indices of fecal microbiota in suckling piglets are shown in Table 2. The Shannon and Simpson indices of the fecal bacterial community were higher in 7-day piglets from sows fed the MET vs. CON diet (P < 0.05). The ACE, Chao1, observed species, and Simpson indices were higher in 21-day piglets from sows fed the MET vs. CON diet (P < 0.05).
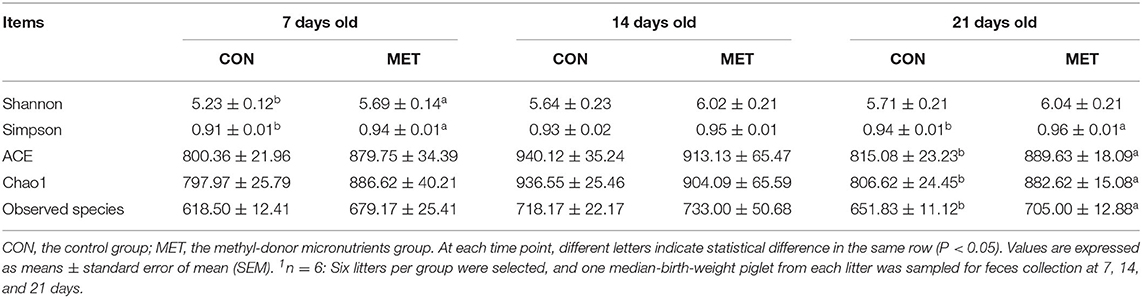
Table 2. Effect of maternal methyl-donor micronutrient supplementation during gestation on the alpha diversity indices of fecal microbiota in suckling piglets (n = 6)1.
Figure 2 presents the PCoA analysis of the fecal microbial community in suckling piglets from sows fed the MET vs. CON diet. PERMANOVA (Adonis procedure with 999 permutations) revealed distinct clustering patterns of feces microbiota in offspring piglets between two treatments at 7 days (R2 = 0.17, P < 0.05) and 21 days (R2 = 0.14, P < 0.05).
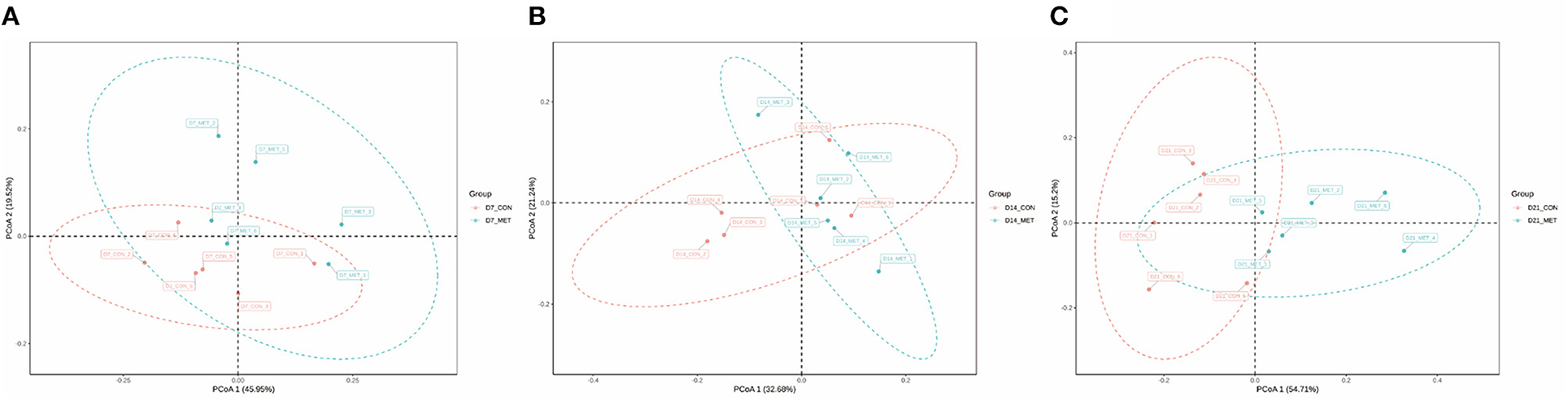
Figure 2. The PCoA analysis of the fecal microbial community in suckling piglets from sows fed the MET vs. CON diet. The PCoA plot was generated by the weighted UniFrac distances. Each dot represents an individual sample of piglet (n = 6). n = 6: Six litters per group were selected, and one median-birth-weight piglet from each litter was sampled for feces collection at 7 (A), 14 (B), and 21 days (C). CON, the control group; MET, the methyl-donor micronutrients group.
Fecal Microbial Composition at Phylum and Genus Level
In both treatment groups, the Bacteroidetes, Firmicutes, Fusobacteria, and Proteobacteria were the dominant microbial phyla in the feces of offspring piglets, followed by Spirochaetes, Euryarchaeota, Actinobacteria, unidentified_Bacteria, Acidobacteria, and Tenericutes (Figure 3A). The relative abundance of Firmicutes and the Firmicutes/Bacteroidetes ratio were increased, and the relative abundance of Bacteroidetes were decreased in the feces of 21-day piglets from the MET group compared with the CON group (P < 0.05) (Figures 3B–D).
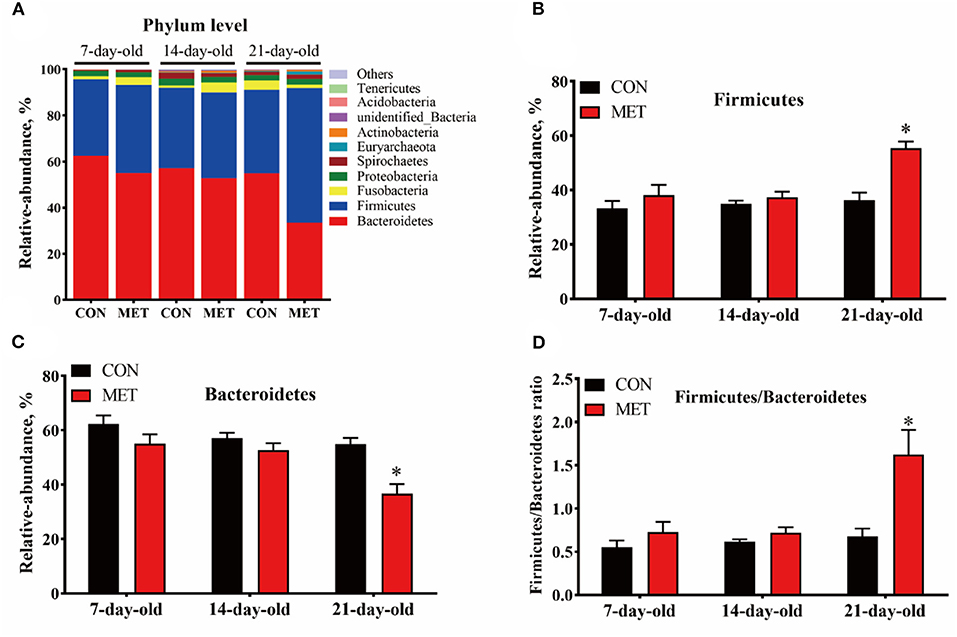
Figure 3. The composition of fecal microbiota in suckling piglets from sows fed the MET vs. CON diet at the phylum level. The relative abundance of the top 10 phyla (A) and the bacterial phyla differed (B–D) between suckling piglets from CON- and MET-fed sow. Each dot represents an individual piglet (n = 6), and * indicates P < 0.05. n = 6: Six litters per group were selected, and one median-birth-weight piglet from each litter was sampled for feces collection at 7, 14, and 21 days. CON, the control group; MET, the methyl-donor micronutrients group.
The 10 most abundant genera in the feces of offspring piglets are unidentified_Prevotellaceae, Lactobacillus, Bacteroides, unidentified_Muribaculaceae, unidentified_Ruminococcaceae, Fusobacterium, Streptococcus, Phascolarctobacterium, Alloprevotella, unidentified_Spirochaetaceae (Figure 4A). The relative abundances of Megasphaera and Dialister were increased in the feces of 7-day piglets, and relative abundances of Sharpea, Weissella, and Pediococcus were increased in the feces of 21-day piglets when their mothers were fed the MET vs. CON diet (P < 0.05) (Figures 4B–F).
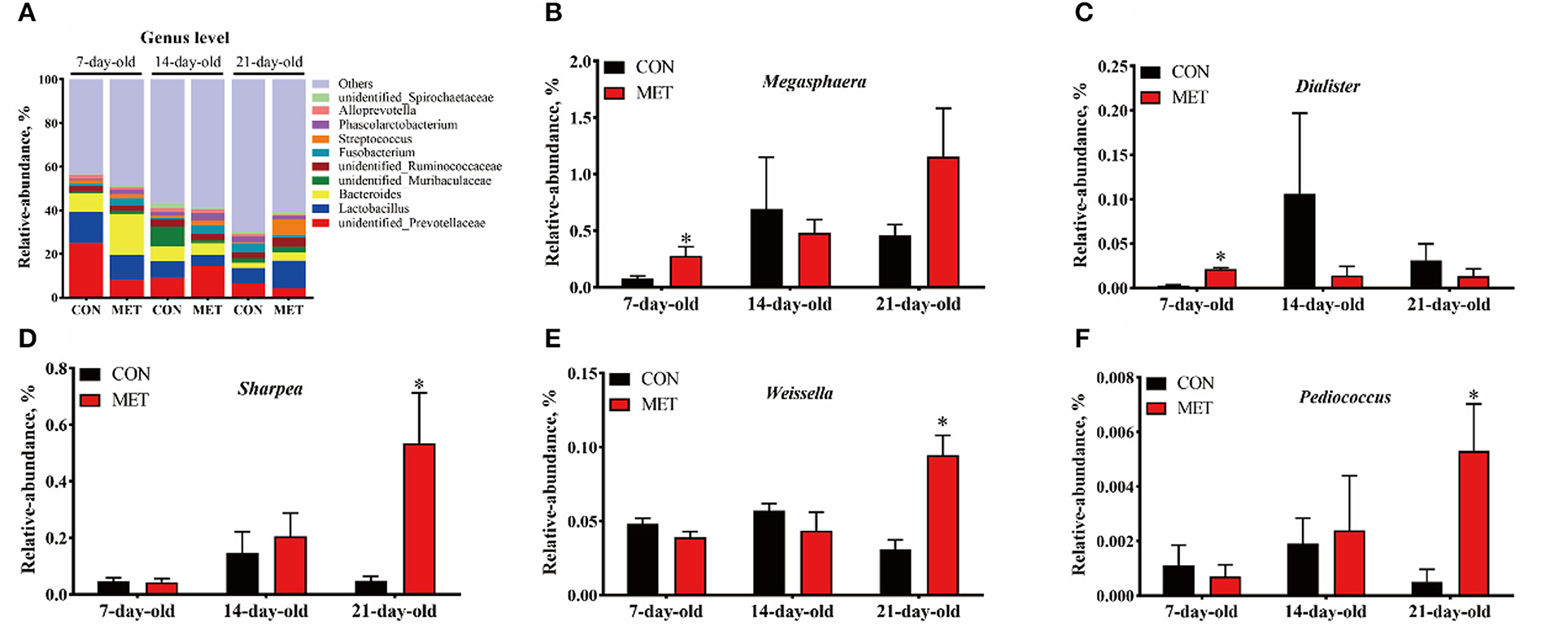
Figure 4. The composition of fecal microbiota in suckling piglets from sows fed the MET vs. CON diet at the genus level. The relative abundance of the top 10 genera (A) and the bacterial genera differed (B–F) between suckling piglets from CON- and MET-fed sow. Each dot represents an individual piglet (n = 6), and * indicates P < 0.05. n = 6: Six litters per group were selected, and one median-birth-weight piglet from each litter was sampled for feces collection at 7, 14, and 21 days. CON, the control group; MET, the methyl-donor micronutrients group.
Differential Fecal Microbial Communities
Differences in the relative abundances of the microbial community components of CON and MET offspring were further analyzed by LEfSe. Compared with the CON group, including unknown bacteria, 14 microbial phlotypes were higher and 14 were lower in the 7-day suckling piglets from MET-fed sows (Figure 5A). At the age of 14 days, compared with the CON group, only one microbial phlotype was higher and two were lower in the MET offspring (Figure 5B). There were 42 higher and 16 lower microbial phlotypes in 21-day piglets from sows fed the MET vs. CON diet (Figure 5C).
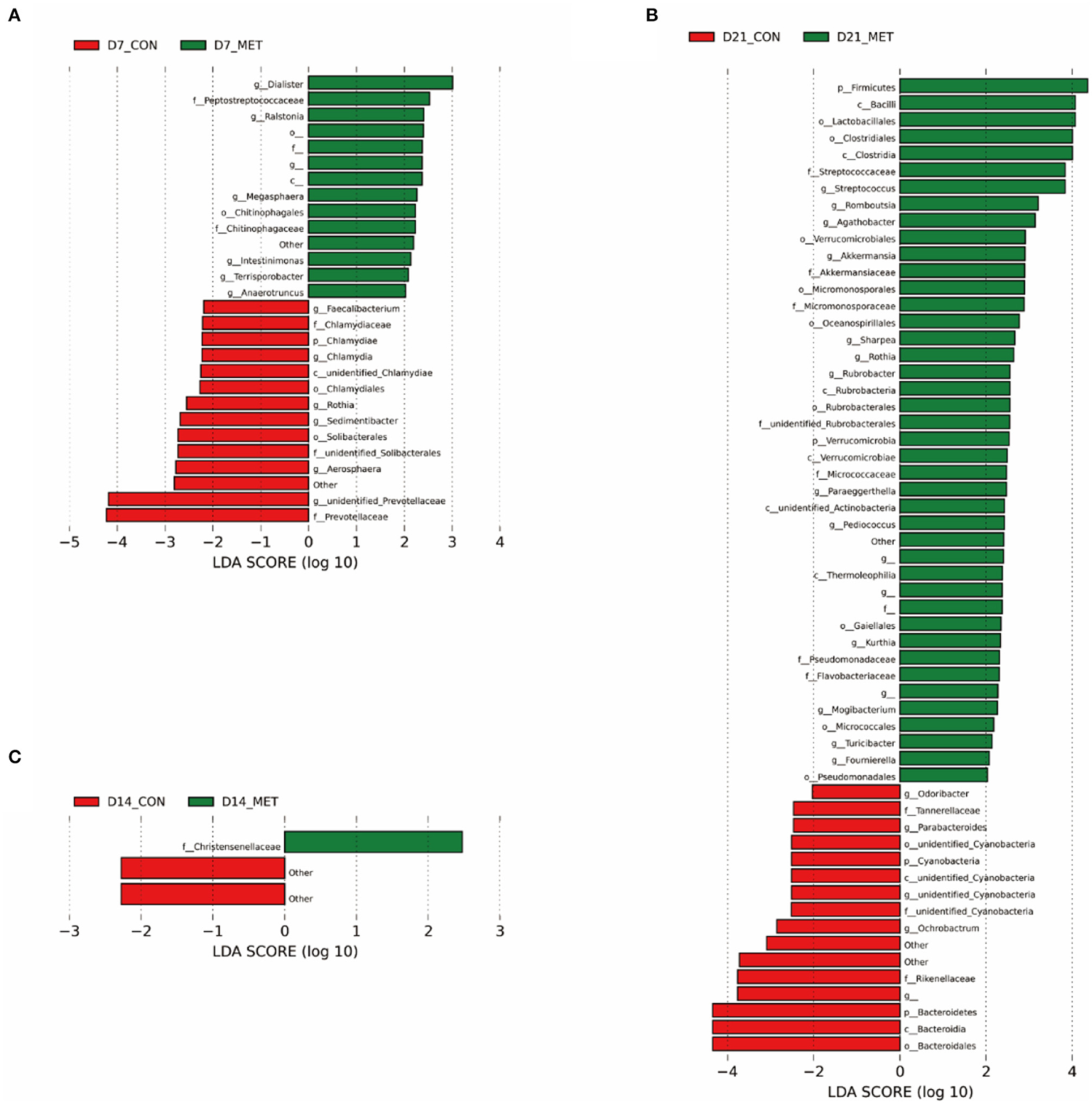
Figure 5. The LEfSe analysis of the fecal bacterial community in 7 (A), 14 (B), and 21- day (C) suckling piglets from sows fed the MET vs. CON diet (n = 6). The default parameter of the LDA score is 4. The bacteria that are not named in the figure are those that have not yet been named. n = 6: Six litters per group were selected, and one median-birth-weight piglet from each litter was sampled for feces collection at 7, 14, and 21 days. CON, the control group; MET, the methyl-donor micronutrients group; LDA, linear discriminant analysis; p_, phylum; c_, class; o_, order; f_, family; g_, genus.
Specific differentiated phylotypes were identified in 7-day suckling piglets from sows fed the MET vs. CON diet: increased genera Dialister, Ralstonia, Megasphaera, Intestinimonas, Terrisporobacter, Anaerotrumcus and families Peptostretococcaceae, Chitinophagales and order Chitinophagaceae (P < 0.05), decreased genera Faecalibacterium, Rothia, Sedimentibacter, Aerosharea, unidentified-Prevotellaceae, Chlamydia and families Chlamydiaceae, Prevotellaceae, unidentified-Solibacterales and order Solibacterales, Chlamydiales and class unidentified-Chlamydiae, phylum Chlamydiae (P < 0.05). At the age of 14 days, the suckling piglets from sows fed MET had significantly higher family Christensenellaceae (P < 0.05); at the age of 21 days, the suckling piglets from sows fed MET had increased genera Streptococcus, Romboutsia, Agathobacter, Akkermansia, Sharpea, Rothia, Rubrobacter, Paraeggerthella, Pediococcus, Kurthia, Mogibacterium, Turicibacter, Foumirerlla and families Streptococcaceae, Akkermansiaceae, Micromonosporaceae, unidentified-Rubrobacterales, Micrococcaceae, Pseudomonadaceae, Flavobacteriaceae and orders Lactobacillales, Clostridiales, Verrucomicrobiales, Micromonosporaceae, Oceanospirillales, Rubrobacterales, Gaiellales, Micrococcales, Pseudomonadales and classes Bacilli, Clostridia, Rubrobacteria, Verrucomicrobia, unidentified-Actinobacteria, Thermoleophilia and phyla Firmicutes, Verrucomicrobia (P < 0.05), decreased genera Odoribater, Parabacteroides, unidentified-Cyanobacteria, Ochrobactrum and families Tanerellaceae, unidentified-Cyanobacteria, Rikenellaceae and order unidentified-Cyanobacteria, Bacteroidales and classes unidentified-Cyanobacteria, Bacteroidia and phyla Cyanobacteria, Bacterioidetes (P < 0.05).
Fecal SCFA Concentration
As displayed in Table 3, no statistical differences were observed in the fecal SCFA concentration of 7-day piglets between maternal dietary treatments (P > 0.05). However, the concentration of acetate, butyrate, and total SCFA were increased in the feces of 14-day piglets from sows fed the MET vs. CON diet (P < 0.05). Besides this, maternal MET supplementation increased the concentration of acetate, propionate, isobutyrate, butyrate, isovalerate, valerate, valerate, and total SCFA in the feces of 21-day piglets (P < 0.05).
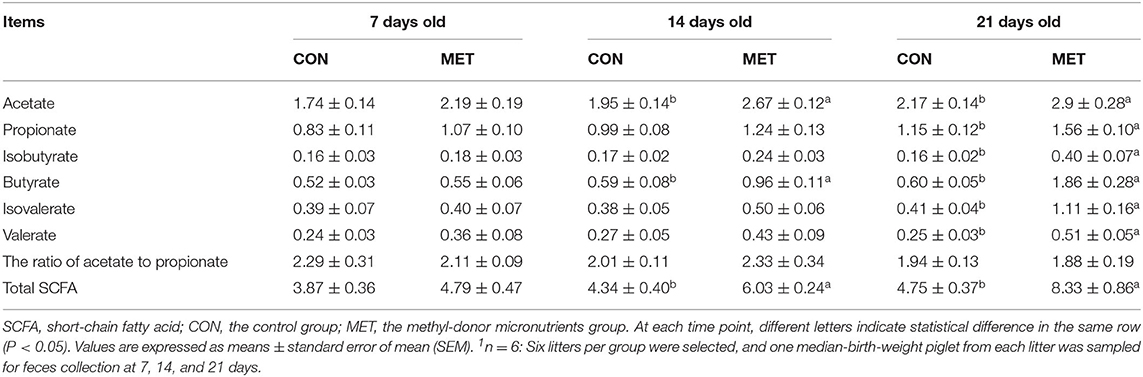
Table 3. Effect of maternal methyl-donor micronutrient supplementation during gestation on the concentration of short-chain fatty acids (SCFA) in feces of suckling piglets (mg/g) (n = 6)1.
Fecal Metabolic Profiling
As graphed in Figure 6, the separation in positive ionization mode between the two groups by the OPLS-DA method was evaluated. OPLS-DA plots of the fecal metabolomics data show a clear separation with no overlap for each group, the parameters for the explanatory and predictive values of the intercepts (R2, Q2), which were stable and good to fitness and prediction (Supplementary Figure 1).
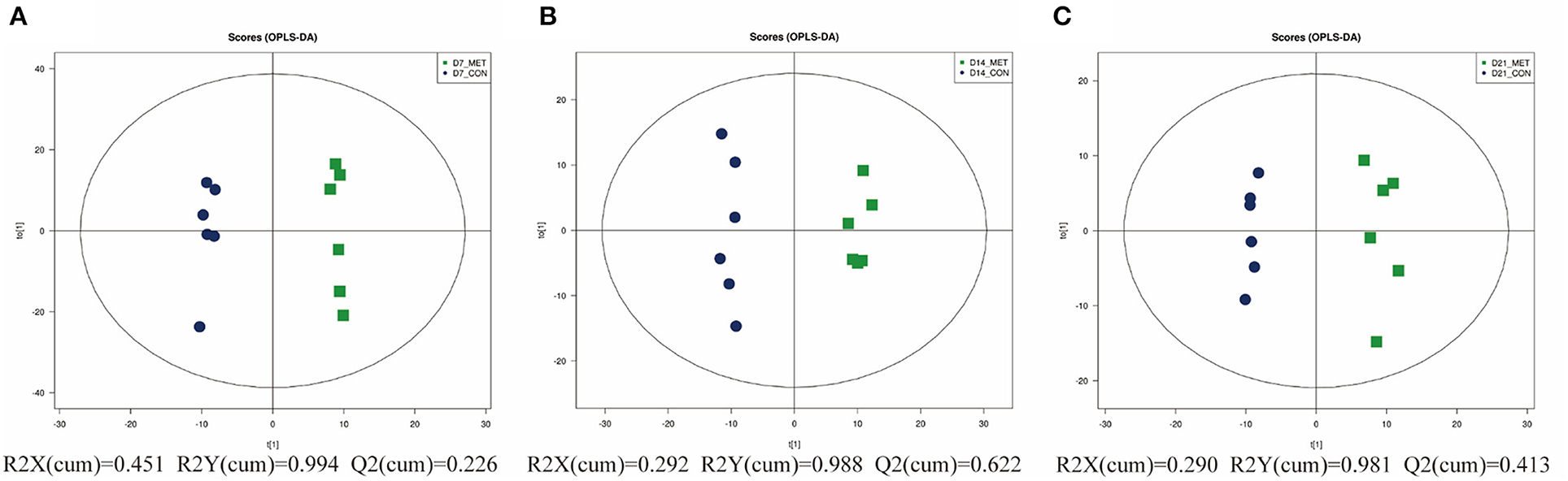
Figure 6. The OPLS-DA analysis (orthogonal partial least squares discriminant analysis) of fecal microbial metabolites in 7 (A), 14 (B), and 21- day (C) suckling piglets from sows fed the MET vs. CON diet (n = 6). n = 6: Six litters per group were selected, and one median-birth-weight piglet from each litter was sampled for feces collection at 7, 14, and 21 days. CON, the control group; MET, the methyl-donor micronutrients group.
Differential Metabolites and Bioinformatics Analysis
Based on the VIP of the OPLS-DA model and the p-value of the Student's t-test, the biologically important difference metabolites were mined. Including significantly differential and differential metabolites, a total of 24 differentiated metabolites were identified from two data sets in 7-day suckling piglets (Figure 7A), 42 differentiated metabolites were identified from two data sets in 14-day suckling piglets (Figure 7B), and 29 differentiated metabolites were identified from two data sets in 21-day suckling piglets (Figure 7C). We subsequently analyzed the significantly metabolite differences in the Kyoto Encyclopedia of Genes and Genomes (KEGG) pathway database (http://www.genome.jp/kegg/) to pathways in offspring that may have been influenced by maternal MET during gestation. These findings revealed that the potential biomarkers contribute to various processes, including biosynthesis of amino acids, amino acid metabolism, pyrimidine metabolism, purine metabolism, amino sugar and nucleotide sugar metabolism, bile secretion, thiamine metabolism, vitamin B6 metabolism, riboflavin metabolism, nicotinate and nicotinamide metabolism, sulfur metabolism, and glutathione metabolism (Tables 4–6).
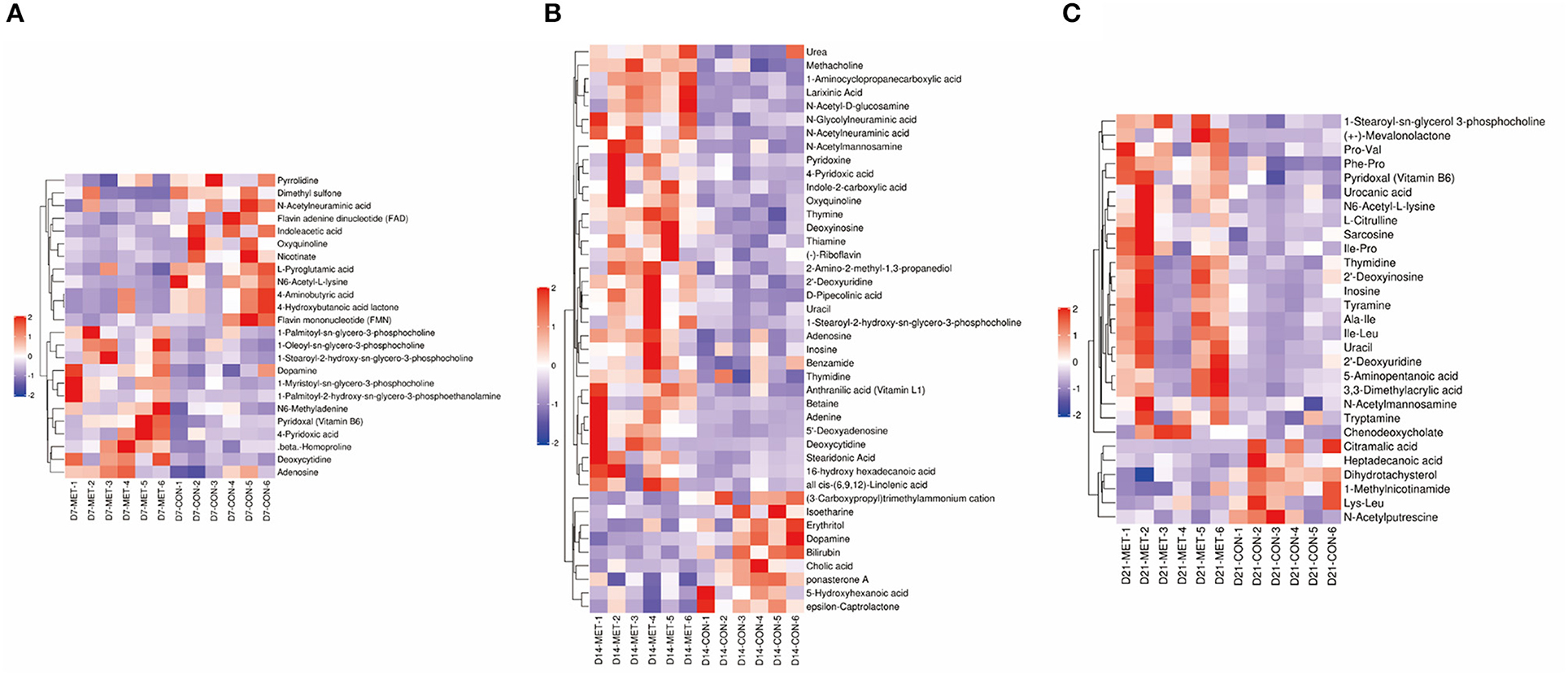
Figure 7. The hierarchical clustering of fecal metabolic profile in 7 (A), 14 (B), and 21- day (C) suckling piglets from sows fed the MET vs. CON diet (n = 6). The color scale ranges from saturated purple (−2) to blank (0) to saturated red (2). Red and purple color represent increased and decreased metabolites, respectively. X axis: sample code, Y axis: metabolite name. n = 6: Six litters per group were selected, and one median-birth-weight piglet from each litter was sampled for feces collection at 7, 14, and 21 days. CON, the control group; MET, the methyl-donor micronutrients group.
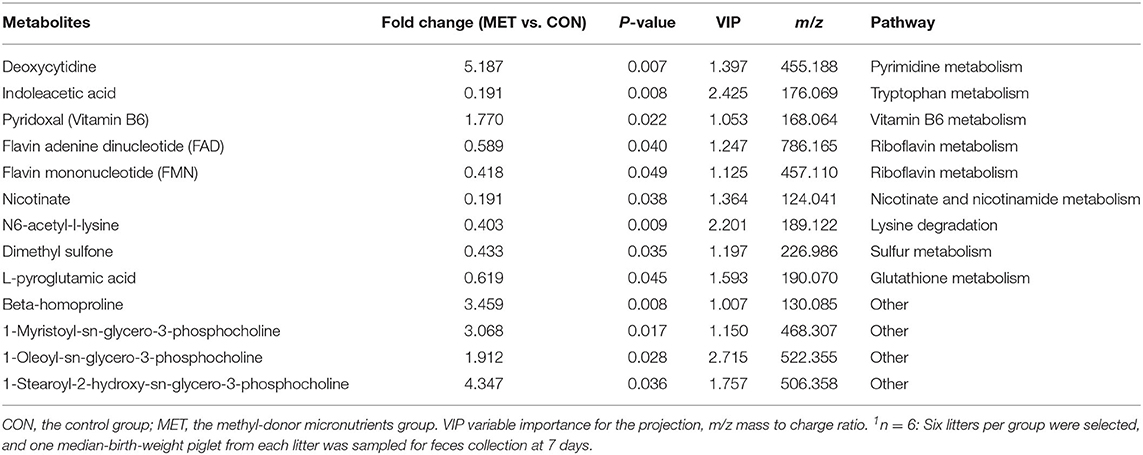
Table 4. The significantly changed fecal metabolites in 7-day suckling piglets from sows fed the MET vs. CON diet (n = 6)1.
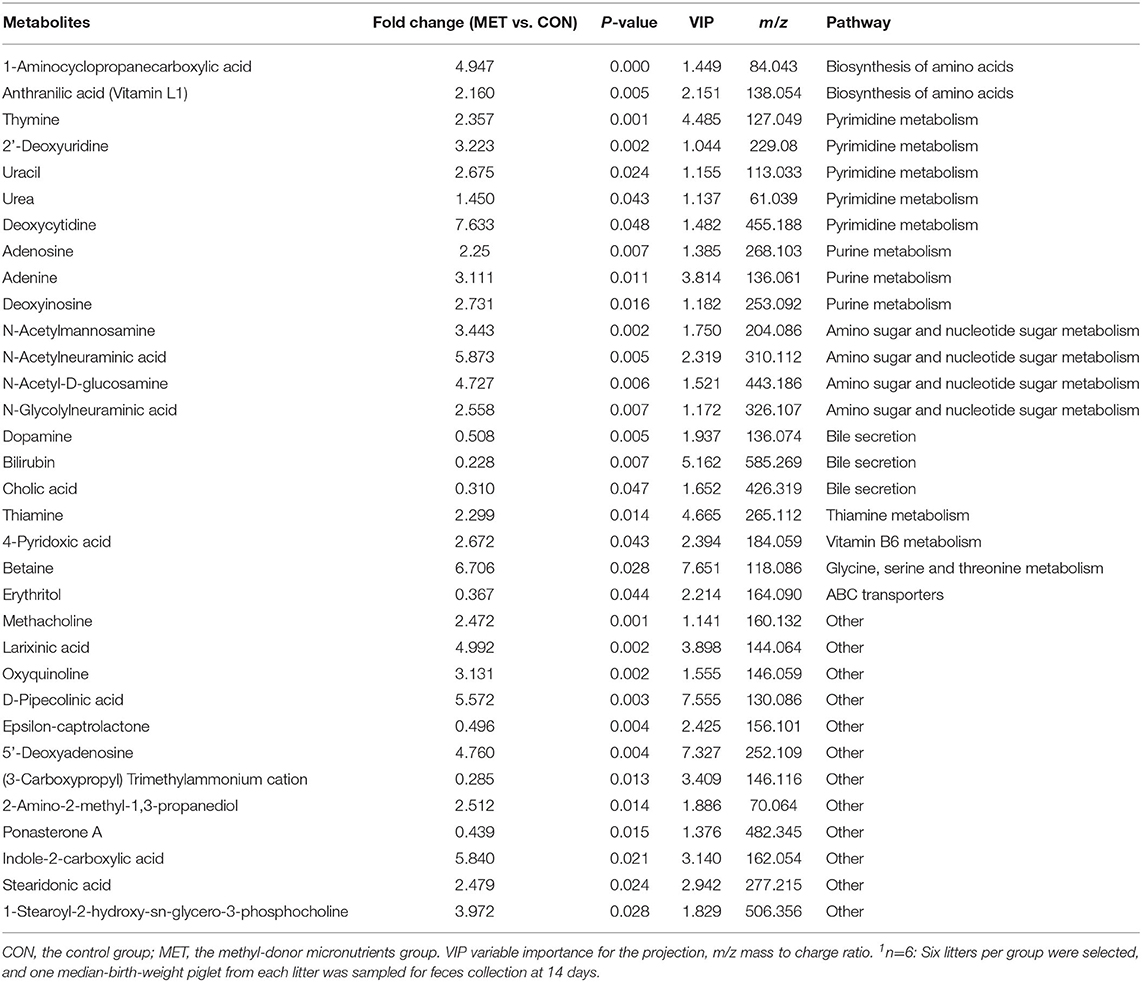
Table 5. The significantly changed fecal metabolites in 14-day suckling piglets from sows fed the MET vs. CON diet (n = 6)1.
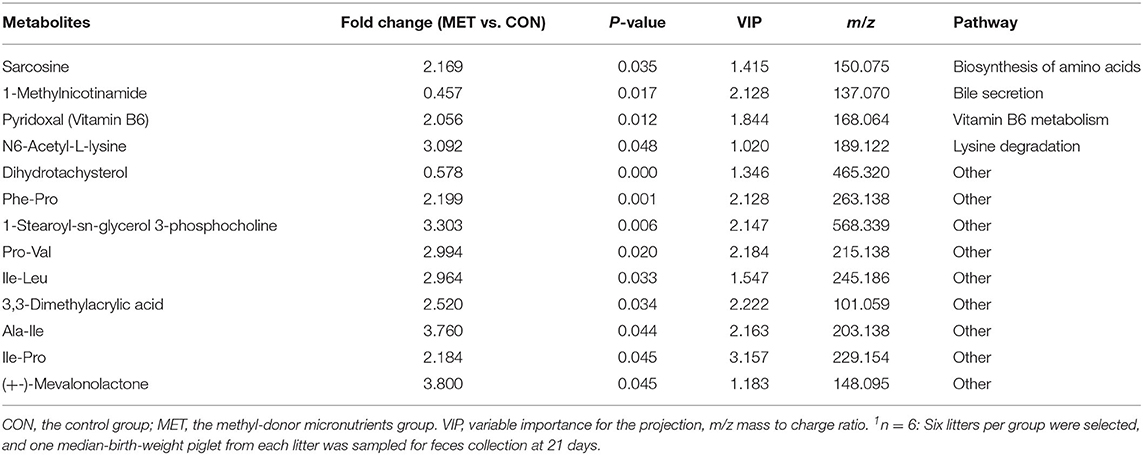
Table 6. The significantly changed fecal metabolites in 21-day suckling piglets from sows fed the MET vs. CON diet (n = 6)1.
Specific differentiated metabolites were identified in 7-day suckling piglets of CON-fed and MET-fed sows: Deoxycytidine closely related to pyrimidine metabolism was increased 5.187-fold in MET piglets compared with CON piglets (P < 0.05). Pyridoxal closely related to vitamin B6 metabolism was increased 1.770- fold in MET compared with the CON group (P < 0.05). Indoleacetic acid closely related to tryptophan metabolism was decreased 0.191-fold in MET piglets compared with CON piglets (P < 0.05). Riboflavin metabolism (flavin adenine dinucleotide and flavin mononucleotide) were decreased 0.59- and 0.42-fold in MET piglets compared with CON piglets (P < 0.05). In the 14-day suckling piglets, critical metabolites (1-aminocyclopropanecarboxylic acid, anthranilic acid) closely related to biosynthesis of amino acids were increased by 4.95- and 2.16-fold in the MET group compared with the CON group, respectively (P < 0.05). Thymine, 2'-deoxyuridine, uracil, urea, and deoxycytidine, which are involved in pyrimidine metabolism, were increased in the MET compared with the CON group by 2.36-, 3.22-, 2.68-, 1.45-, and 7.63-fold, respectively (P < 0.05), and concentrations of adenosine, adenine, and deoxyinosine, which are involved in purine metabolism, were increased in the MET compared with the CON group by 1.39-, 3.81-, and 1.18-fold, respectively (P < 0.05). Critical metabolites (dopamine, bilirubin, and cholic acid) closely related to bile secretion were decreased in the MET group. In addition, critical metabolites closely related to amino acid and vitamin B6 metabolism were increased in the MET group compared with the CON group (P < 0.05). In the 21-day suckling piglets,: critical metabolites related to biosynthesis of amino acids, amino acid metabolism, and vitamin B6 metabolism, were increased in the MET group compared with CON group although bile secretion was decreased in MET piglets (P < 0.05).
Correlation Analysis for Differential Metabolites and Microbes
A Spearman's correlation matrix was generated to explore the correlation between the bacterial genera and candidate compounds that were affected by maternal nutrition. As shown in Figure 8, significant associations could be identified between the gut microbial and the altered metabolite profiles from 7- and 21-day suckling piglets. In the 21-day suckling piglets (Figure 8A), the correlation analysis revealed that deoxycytidine was positively correlated with the genera Dialister and Anaerotrumcus and negatively associated with the genera Rothia, Sedimentibacter, and unidentified-Prevotellaceae (P < 0.05). Indoleacetic acid was negatively associated with the genera Dialister and Terrisporobacter and positively correlated with the genera Sedimentibacter, unidentified-Prevotellaceae, and Chlamydia (P < 0.05). The genera Dialister, Megasphaera, and Terrisporobacter were positively correlated with vitamin B6 (P < 0.05). In addition, the genus Sedimentibacter was positively correlated with flavin adenine dinucleotide and flavin mononucleotide (P < 0.05). In the 21-day suckling piglets (Figure 8B), sarcosine was negatively associated with the genera Parabacteroides and unidentified-Cyanobacterir and positively correlated with the genera Rothia, Rubrobacter, Pediococcus, and Mogibacterium (P < 0.05). 1-Methylnicotinamide was negatively associated with the genera Agathobacter, Paraeggerthella, and Pediococcus and positively correlated with the genera Odoribater and Ochrobactrum (P < 0.05). Nine genera, including Streptococcus, Akkermansia, Rothia, Rubrobacter, Pediococcus, Mogibacterium, Turicibacter, and Foumirerlla were positively correlated with vitamin B6 (P < 0.05).
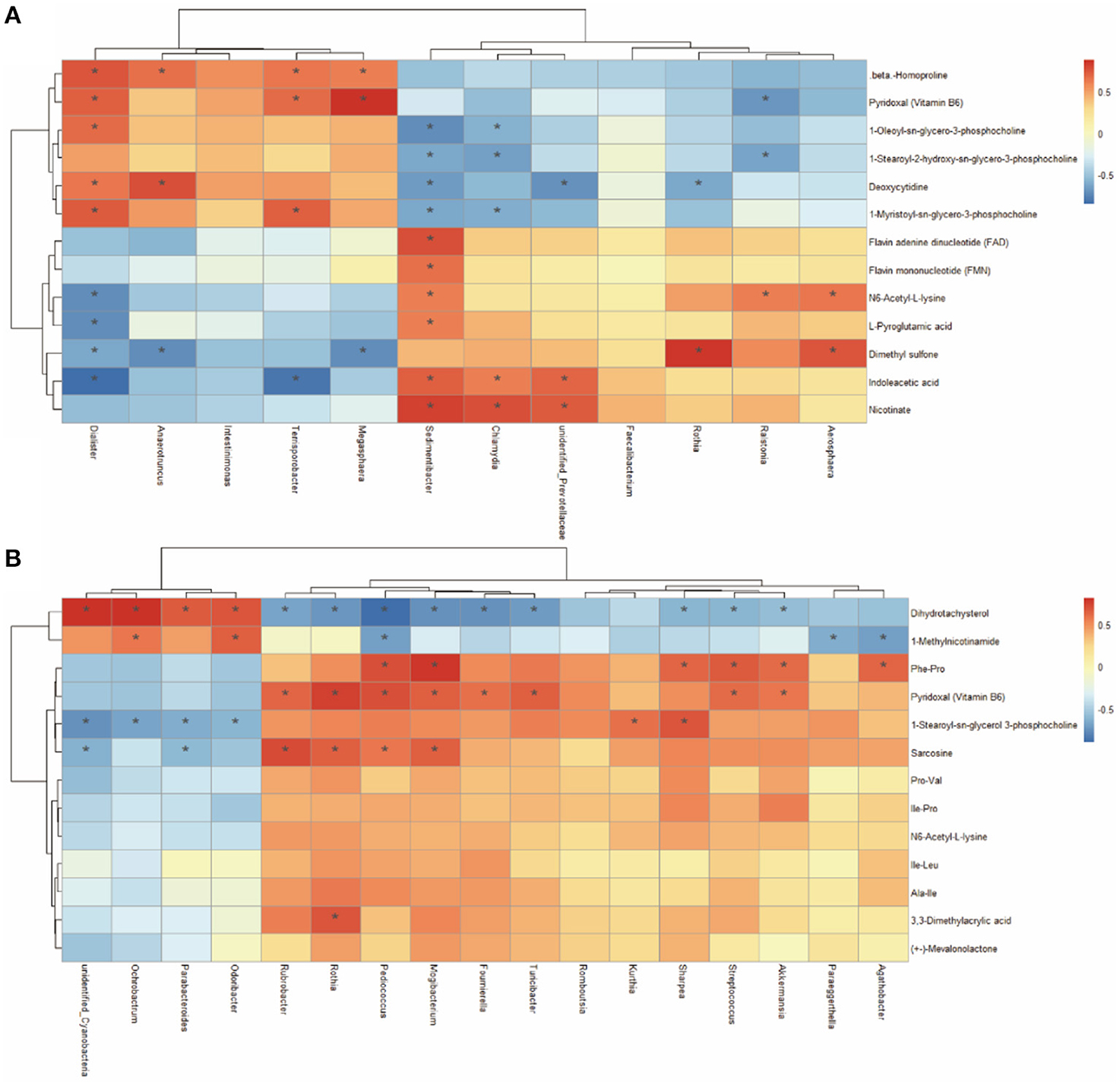
Figure 8. Spearman correlation analysis between genera and metabolite concentrations in 7 (A) and 21- day (B) suckling piglets from sows fed the MET vs. CON diet (n = 6). *Asterisks indicate significant correlations between genera and metabolite concentrations. Cells are colored based on the Spearman correlation coefficient between the significantly altered genera and metabolites; red represents a significantly positive correlation, blue represents a significantly negative correlation (P < 0.05), and white represents no significant correlation (P > 0.05). n = 6: Six litters per group were selected, and one median-birth-weight piglet from each litter was sampled for feces collection at 7, 14, and 21 days.
Discussion
In a previous study, we found no differences in reproductive performance of sows between CON and MET; however, we observed that the offspring of sows fed with the MET diet grew faster than control offspring, and maternal MET exposure can promote skeletal muscle differentiation and maturity and improve the skeletal muscle mass of weaning piglets (14). Previous research reports the intestinal tract of the monogastric animal plays a crucial role in nutrient digestion and absorption and maintains the barrier function against malignant pathogens and antigens (20). In addition, gut microbiota contributes to muscle mass and muscle fiber types through the gut–muscle axis (21). Thus, in the present study, we collected fresh fecal samples from piglets at 7, 14, and 21 days and further analyzed the effects of MET on intestine microbiota colonization and metabolism in offspring. Our findings show that the establishment of the suckling piglets' gut microbiota was strongly affected by maternal MET, and the MET offspring have a higher growth performance, which is concomitant with alterations in the intestinal microbiota alpha diversity, microbiota composition, SCFA, and the biosynthesis of amino acids. Furthermore, we found associations between the differentially abundant genera and metabolites in the CON and MET groups.
Establishment of the Gut Microbial Community in Suckling Piglets
The gut microbiota diversity is highly related to host health and metabolic capacity (22). The low diversity of intestinal microbiota is considered a mark of intestinal dysbiosis, which may lead to autoimmune diseases, inflammatory bowel diseases, diarrhea, and metabolic diseases (23, 24). Increased bacterial richness and diversity is a marker of the establishment of intestinal microbiota (10). Our findings show that bacterial richness and diversity were increased in 7-day piglets from the MET sows compared with those from the CON sows. In addition, bacterial richness and uniformity were significantly higher in MET offspring at 21 days of age, suggesting that MET offspring had a more diversified intestinal microbiota. However, there was no difference in the microbiota diversity of 14-day piglets, this is probably because the gut microbial community is unstable and in a stage of rapid change. The bacterial communities were dominated by Firmicutes and Bacteroidetes in agreement with a previous study (25, 26). The increase in Firmicutes phylum is considered a marker of the establishment of intestinal microbiota (1, 27, 28). Previous studies found that the F/B is proportional to body weight because the increase in Firmicutes is closely related to energy intake (29, 30), and the relative abundance of Bacteroidetes is associated with degradation of proteins and carbohydrates (31). In our study, the microbiota of MET piglets showed an increase in F/B over time, characterized by increases in Firmicutes abundance. This suggesting that the intestinal microbiota of MET offspring may be more efficient than CON offspring at extracting energy from their diet, and consequently, they gain more weight.
Differences in the Gut Microbiota Between CON and MET Offspring During Suckling Period
As is previously reported, an increase in methionine intake from the diet would lead to an increased amount of methionine in the lumen and provide an excellent fuel source for the rapid proliferation of bacteria (32). However, a lack of methyl donors can affect the differentiation and barrier functions of the small intestine and increase the concentration of Hcy, which subsequently promotes oxidative stress and activates interrelated pro-inflammatory mechanisms, ultimately aggravating inflammatory bowel disease in rats (13, 33). MET supplementation through the diet limits pathobiont colonization of the gut via inducing a specific intestinal micro-environment (11). When the harmful bacteria decrease, the beneficial bacteria may increase relatively. In our study, maternal MET supplementation during gestation decreased serum Hcy concentration in the offspring. Moreover, we identified that MET offspring at 7 days had a significantly higher abundance of Dialister and Megasphaera in the feces than CON offspring, and MET offspring at 21 days had a significantly higher abundance of Romboutsia, Akkermansia, Sharpea, Turicibacter weissella, and pediococcus in the feces than CON offspring. Dialister populations utilize succinate and produce propionate, Megasphaera can convert lactic acid to propionate via the acrylate pathway (34), and the Sharpea is beneficial to lactate production (35). Previous studies indicate that Akkermansia is linked with improved metabolic parameters; hosts with low Akkermansia content are susceptible to obesity, inflammation, and type 2 diabetes (36). As putatively beneficial gut microbiota, Akkermansia reinforces the gut barrier function and eventually reduces plasma lipopolysaccharide (37, 38). Previous studies also show that high relative abundances of Romboutsia are associated with decreased risk for infection in kidney transplant recipients (39), the Turicibacter might play a role in inflammatory bowel diseases and was proportional in concentration to that of butyric acid (40, 41). Weissella and pediococcus are recognized probiotic genera, which play a role in improving the health of the host (42, 43). Our data show that the relative abundance of beneficial bacteria is increased in MET piglets, which could play an inhibitory role in the growth of intestinal pathogenic bacteria and relieve metabolic diseases and intestinal inflammation (44, 45).
Changes in Feces SCFA Between CON and MET Offspring During Suckling Period
Our results indicate that maternal MET supplementation during gestation increased the concentrations of individual and total SCFAs of offspring at 21 days of age. As for the reason for the increased SCFAs in the feces of suckling piglets, a previous study reports that acetate, propionate, and butyrate were formed from cysteine, whereas the main products of methionine metabolism were propionate and butyrate (46). Supplementing DL-MHA (a methionine substitute) in the diet increased the concentration of acetic acid, valeric acid, and total SCFAs in the cecum of piglets (47). Due to the sparing effect between methionine and cysteine, the supplementation of MET may lead to quantities of residual Cys in the gut to produce the SCFAs. In addition, the relative abundance of SCFA-producing Dialister, Megasphaera, Turicibacter, and Sharpea were increased in the MET group, which may also explain the increased production of SCFAs in the intestine. As the main source of energy for epithelial cells, SCFA can increase the proliferation of intestinal tissues (48, 49), butyrate can decrease intestinal inflammation, and as it enhances the intestinal barrier function (50), propionate can be used by hepatocyte cells of the liver for gluconeogenesis (51). That beneficial bacteria were increased in the MET offspring may be associated with the increase of SCFAs, which destroy microbial pathogens (52). However, no alteration in the concentrations of individual or total SCFAs was observed when the offspring piglets were 7 days old. Although the butyric- and lactic-producing bacteria were increased, the total intestinal microbiota of 7-day piglets was relatively low, and the amount of SCFA produced was also low, so the difference is not significant.
Changes in Feces Metabolism Between CON and MET Offspring During Suckling Period
Maternal nutrition is clearly an important determinant of offspring gut microbiota, which has in turn linked with host metabolism and health (53, 54). The increase in BW at weaning might result from the increased metabolism of biosynthesis of amino acids, pyrimidine, and purine, which are positively correlated with growth. Previously, it was reported that amino acids not only serve as substances for synthesis of tissue proteins, but also as substrates for the synthesis of many low-molecular-weight substances (55). In our study, we found that maternal exposure to MET increased the concentrations of amino acid metabolism (including anthranilic acid, 1-aminocyclopropanecarboxylic acid, and sarcosine) in the offspring at 14 or 21 days. Meanwhile, the concentrations of metabolites involved in purine and pyrimidine metabolism increased, which indicated an enhanced function in nucleic acid metabolism via one-carbon metabolism (56) mainly because folate metabolism provides building blocks (10-formyl-tetrahydrofolate, methylene-tetrahydrofolate, respectively) for purine and pyrimidine synthesis. Furthermore, the amino and nucleotide sugars were increased in 14-day offspring of MET-supplemented sows. Notably, bile acid metabolites (cholic acid, dopamine, bilirubin, and 1-methylnicotinamide) were increased in 14- or 21-day offspring of sows in the CON group. The increase in bile acid metabolites could be caused by reduced reabsorption in the intestine (57). Bile acids (BAs) play an important role in the intestines, facilitating fat digestion and the absorption of lipids and liposoluble vitamins. Approximately 90% of bile acids return from the intestinal cavity to the liver via the portal vein (58). High concentrations of bile acid are toxic to mammalian cells (59). Cholic acid, as one of the BAs produced by the liver, is involved in the primary BA biosynthesis pathway (60). The elevation of cholic acid is a potential biomarker of liver injury, and extra cholic acid may partly lead to an increased risk of inflammation (61, 62). Total bilirubin is another indirect marker of liver function. Therefore, the decreased levels of cholic acid and bilirubin suggest that maternal exposure to MET during pregnancy may improve liver function of offspring.
The maternal metabolic status during gestation exerts a significant influence on the infant microbiota at the beginning of life (63). Maternal MET exposure can alter serum one-carbon metabolism of sows (14) and offspring. One-carbon metabolism participates in the synthesis of nucleotides, proteins, and lipids by integrating glucose, amino acid status, and vitamins in suckling piglets (8). In addition, there is increasing evidence that microbiota-derived metabolites act as key factors regulating animal metabolism, growth, and development (10). In this study, Spearman's correlation revealed an association between the abundance of specific bacterial genera and metabolites that were significantly influenced by maternal exposure to MET. Particularly, the VB6 and sarcosine were positively correlated with the genera Rothia, Rubrobacter, Pediococcus, and Mogibacterium. Altogether, the establishment of a gut microbial community and metabolic homeostasis could be a major underlying factor that induces improved growth and development of MET suckling piglets.
Conclusion
Collectively, maternal methyl-donor micronutrient addition altered gut microbiota and the fecal metabolic profile, resulting in an improved weaning weight of offspring piglets.
Data Availability Statement
The raw sequences used in this study were stored on the Sequence Read Archive (SRA) of NCBI, and the SRA accession number is PRJNA694233.
Ethics Statement
The animal study was reviewed and approved by the Animal Care and Use Committee of Jiangxi Agricultural University (Ethic Approval Code: JXAUA01).
Author Contributions
JY and TZ designed the study. QH, LJ, and JH performed the animal feeding experiment and sample analysis. FX assisted with SCFAs analysis. QH collected the data and wrote the manuscript. ZW and JC finalized the manuscript. All authors agree to be accountable for the content of the work.
Funding
The work was supported by the National Key Research and Development Program of China (2018YFD0500401).
Conflict of Interest
The authors declare that the research was conducted in the absence of any commercial or financial relationships that could be construed as a potential conflict of interest.
Supplementary Material
The Supplementary Material for this article can be found online at: https://www.frontiersin.org/articles/10.3389/fnut.2021.675640/full#supplementary-material
References
1. Muinck EJ, Trosvik P. Individuality and convergence of the infant gut microbiota during the first year of life. Nat Commun. (2018) 9:2233. doi: 10.1038/s41467-018-04641-7
2. Sonnenburg JL, Bäckhed F. Diet–microbiota interactions as moderators of human metabolism. Nature. (2016) 535:56–64. doi: 10.1038/nature18846
3. Ganal-Vonarburg SC, Hornef MW, Macpherson AJ. Microbial-host molecular exchange and its functional consequences in early mammalian life. Science. (2020) 368:604–7. doi: 10.1126/science.aba0478
4. Steegers-Theunissen RPM, Twigt J, Pestinger V, Sinclair KD. The periconceptional period, reproduction and long-term health of offspring: the importance of one-carbon metabolism. Hum Reprod Update. (2013) 19:640–55. doi: 10.1093/humupd/dmt041
5. Gabbianelli R. Modulation of the epigenome by nutrition and xenobiotics during early life and across the life span: the key role of lifestyle. Lifestyle Genomics. (2018) 11:9–12. doi: 10.1159/000490751
6. Vickers M. Early life nutrition, epigenetics and programming of later life disease. Nutrients. (2014) 6:2165–78. doi: 10.3390/nu6062165
7. Yu W, Wang Z, Zhang K, Chi Z, Xu T, Jiang D, et al. One-Carbon metabolism supports s-adenosylmethionine and histone methylation to drive inflammatory macrophages. Mol Cell. (2019) 75:1147–60. doi: 10.1016/j.molcel.2019.06.039
8. Wu S, Zhang J, Li F, Du W, Zhou X, Wan M, et al. One-Carbon metabolism links nutrition intake to embryonic development via epigenetic mechanisms. Stem Cells Int. (2019) 2019:1–8. doi: 10.1155/2019/3894101
9. Garcia-Mantrana I, Selma-Royo M, Gonzalez S, Parra-Llorca A, Martinez-Costa C, Collado MC. Distinct maternal microbiota clusters are associated with diet during pregnancy: impact on neonatal microbiota and infant growth during the first 18 months of life. Gut Microbes. (2020) 11:962–78. doi: 10.1080/19490976.2020.1730294
10. Li N, Huang S, Jiang L, Wang W, Li T, Zuo B, et al. Differences in the gut microbiota establishment and metabolome characteristics between low- and normal-birth-weight piglets during early-life. Front Microbiol. (2018) 9:1798. doi: 10.3389/fmicb.2018.01798
11. Gimier E, Chervy M, Agus A, Sivignon A, Billard E, Privat M, et al. Methyl-donor supplementation prevents intestinal colonization by Adherent-Invasive E. coli in a mouse model of Crohn's disease. Sci Rep. (2020) 10:12922. doi: 10.1038/s41598-020-69472-3
12. Mir SA, Nagy-Szakal D, Dowd SE, Szigeti RG, Smith CW, Kellermayer R. Prenatal methyl-donor supplementation augments colitis in young adult mice. PLoS ONE. (2013) 8:e73162. doi: 10.1371/journal.pone.0073162
13. Bressenot A, Pooya S, Bossenmeyer-Pourie C, Gauchotte G, Germain A, Chevaux J, et al. Methyl donor deficiency affects small-intestinal differentiation and barrier function in rats. Brit J Nutr. (2013) 109:667–77. doi: 10.1017/S0007114512001869
14. He Q, Zou T, Chen J, Jian L, He J, Xia Y, et al. Maternal methyl-donor micronutrients supplementation during pregnancy promotes skeletal muscle differentiation and maturity in newborn and weaning pigs. Front Nutr. (2020) 30:609022. doi: 10.3389/fnut.2020.609022
15. Oster M, Nuchchanart W, Trakooljul N, Murani E, Zeyner A, Wirthgen E, et al. Methylating micronutrient supplementation during pregnancy influences foetal hepatic gene expression and IGF signalling and increases foetal weight. Eur J Nutr. (2016) 55:1717–27. doi: 10.1007/s00394-015-0990-2
16. Matte JJ, Guay F, Girard CL. Folic acid and vitamin B 12 in reproducing sows: new concepts. Can J Anim Sci. (2006) 86:197–205. doi: 10.4141/A05-059
17. Zhao Y, Jin C, Xuan Y, Zhou P, Fang Z, Che L, et al. Effect of maternal or post-weaning methyl donor supplementation on growth performance, carcass traits, and meat quality of pig offspring. J Sci Food Agr. (2019) 99:2096–107. doi: 10.1002/jsfa.9402
18. Guay F, Matte JJ, Girard CL, Palin MF, Giguere A, Laforest JP. Effect of folic acid and glycine supplementation on embryo development and folate metabolism during early pregnancy in pigs. J Anim Sci. (2002) 80:2134–43. doi: 10.1093/ansci/80.8.2134
19. Liu Y, Wu X, Jiang H. Combined maternal and post-weaning high fat diet inhibits male offspring's prostate cancer tumorigenesis in transgenic adenocarcinoma of mouse prostate model. Prostate. (2018) 79:544–53. doi: 10.1002/pros.23760
20. Azad M, Gao J, Ma J, Li T, Tan B, Huang X, et al. Opportunities of prebiotics for the intestinal health of monogastric animals. Anim Nutr. (2020) 6:379–88. doi: 10.1016/j.aninu.2020.08.001
21. Yan H, Diao H, Xiao Y, Li W, Yu B, He J, et al. Gut microbiota can transfer fiber characteristics and lipid metabolic profiles of skeletal muscle from pigs to germ-free mice. Sci Rep. (2016) 6:31786. doi: 10.1038/srep31786
22. Clarke SF, Murphy EF, O'Sullivan O, Lucey AJ, Humphreys M, Hogan A, et al. Exercise and associated dietary extremes impact on gut microbial diversity. Gut. (2014) 63:1913–20. doi: 10.1136/gutjnl-2013-306541
23. Ott SJ. Reduction in diversity of the colonic mucosa associated bacterial microflora in patients with active inflammatory bowel disease. Gut. (2004) 53:685–93. doi: 10.1136/gut.2003.025403
24. Duca FA, Sakar Y, Lepage P, Devime F, Langelier B, Dore J, et al. Replication of obesity and associated signaling pathways through transfer of microbiota from obese-prone rats. Diabetes. (2014) 63:1624. doi: 10.2337/db13-1526
25. Xiao L, Estellé J, Kiilerich P, Ramayo-Caldas Y, Xia Z, Feng Q, et al. A reference gene catalogue of the pig gut microbiome. Nat Microbiol. (2016) 1:16161. doi: 10.1038/nmicrobiol.2016.161
26. Liu H, Hou C, Li N, Zhang X, Zhang G, Yang F, et al. Microbial and metabolic alterations in gut microbiota of sows during pregnancy and lactation. FASEB J. (2018) 33:4490–501. doi: 10.1096/fj.201801221RR
27. Olivares M, Walker AW, Capilla A, Benitez-Paez A, Palau F, Parkhill J, et al. Gut microbiota trajectory in early life may predict development of celiac disease. Microbiome. (2018) 6:36. doi: 10.1186/s40168-018-0415-6
28. Stewart CJ, Ajami NJ, O'Brien JL, Hutchinson DS, Smith DP, Wong MC, et al. Temporal development of the gut microbiome in early childhood from the TEDDY study. Nature. (2018) 562:583–88. doi: 10.1038/s41586-018-0617-x
29. Zhang W, Ma C, Xie P, Zhu Q, Wang X, Yin Y, et al. Gut microbiota of newborn piglets with intrauterine growth restriction have lower diversity and different taxonomic abundances. J Appl Microbiol. (2019) 127:354–69. doi: 10.1111/jam.14304
30. Singh P, Karimi A, Devendra K, Waldroup PW, Cho KK, Kwon YM. Influence of penicillin on microbial diversity of the cecal microbiota in broiler chickens. Poultry Sci. (2013) 92:272–6. doi: 10.3382/ps.2012-02603
31. Thomas F, Hehemann JH, Rebuffet E, Czjzek M, Michel G. Environmental and gut bacteroidetes: the food connection. Front Microbiol. (2011) 2:93. doi: 10.3389/fmicb.2011.00093
32. Miousse IR, Pathak R, Garg S, Skinner CM, Melnyk S, Pavliv O, et al. Short-term dietary methionine supplementation affects one-carbon metabolism and DNA methylation in the mouse gut and leads to altered microbiome profiles, barrier function, gene expression and histomorphology. Genes Nutr. (2017) 12:22. doi: 10.1186/s12263-017-0576-0
33. Chen M, Peyrin-Biroulet L, George A, Coste F, Bressenot A, Bossenmeyer-Pourie C, et al. Methyl deficient diet aggravates experimental colitis in rats. J Cell Mol Med. (2011) 15, 2486–97. doi: 10.1111/j.1582-4934.2010.01252.x
34. Canfora EE, Meex RCR, Venema K, Blaak EE. Gut microbial metabolites in obesity, NAFLD and T2DM. Nat Rev Endocrinol. (2019) 15:261–73. doi: 10.1038/s41574-019-0156-z
35. Kamke J, Kittelmann S, Soni P, Li Y, Tavendale M, Ganesh S, et al. Rumen metagenome and metatranscriptome analyses of low methane yield sheep reveals a Sharpea-enriched microbiome characterised by lactic acid formation and utilisation. Microbiome. (2016) 4:56. doi: 10.1186/s40168-016-0201-2
36. Plovier H, Everard A, Druart C, Depommier C, Van Hul M, Geurts L, et al. A purified membrane protein from Akkermansia muciniphila or the pasteurized bacterium improves metabolism in obese and diabetic mice. Nat Med. (2017) 23:107–13. doi: 10.1038/nm.4236
37. Depommier C, Everard A, Druart C, Plovier H, Van Hul M, Vieira-Silva S, et al. Supplementation with Akkermansia muciniphila in overweight and obese human volunteers: a proof-of-concept exploratory study. Nat Med. (2019) 25:1096–103. doi: 10.1038/s41591-019-0495-2
38. Liu J, Yue S, Yang Z, Feng W, Meng X, Wang A, et al. Oral hydroxysafflor yellow A reduces obesity in mice by modulating the gut microbiota and serum metabolism. Pharmacol Res. (2018) 134:40–50. doi: 10.1016/j.phrs.2018.05.012
39. Magruder M, Edusei E, Zhang L, Albakry S, Satlin MJ, Westblade LF, et al. Gut commensal microbiota and decreased risk for Enterobacteriaceae bacteriuria and urinary tract infection. Gut microbes. (2020) 12:1805281. doi: 10.1080/19490976.2020.1805281
40. Zhong Y, Nyman M, Fåk F. Modulation of gut microbiota in rats fed high-fat diets by processing whole-grain barley to barley malt. Mol Nutr Food Res. (2015) 59:2066–76. doi: 10.1002/mnfr.201500187
41. Zheng B, Wang T, Wang H, Chen L, Zhou Z. Studies on nutritional intervention of rice starch- oleic acid complex (resistant starch type V) in rats fed by high-fat diet. Carbohydr Polym. (2020) 246:116637. doi: 10.1016/j.carbpol.2020.116637
42. Singh S, Bhatia R, Singh A, Singh P, Kaur R, Khare P, et al. Probiotic attributes and prevention of LPS-induced pro-inflammatory stress in RAW264.7 macrophages and human intestinal epithelial cell line (Caco-2) by newly isolated Weissella cibaria strains. Food Funct. (2018) 9:1254–64. doi: 10.1039/C7FO00469A
43. Chen P, Xu H, Tang H, Zhao F, Yang C, Kwok LY, et al. Modulation of gut mucosal microbiota as a mechanism of probiotics-based adjunctive therapy for ulcerative colitis. Microb Biotechnol. (2020) 13:2032–43. doi: 10.1111/1751-7915.13661
44. Cheng S, Ma X, Geng S, Jiang X, Li Y, Hu L, et al. Fecal microbiota transplantation beneficially regulates intestinal mucosal autophagy and alleviates gut barrier injury. Msystems. (2018) 3:e00137–18. doi: 10.1128/mSystems.00137-18
45. Turroni F, Milani C, Duranti S, Mahony J, van Sinderen D, Ventura M. Glycan utilization and cross-feeding activities by bifidobacteria. Trends Microbiol. (2018) 26:339–50. doi: 10.1016/j.tim.2017.10.001
46. Smith EA, Macfarlane GT. Dissimilatory amino acid metabolism in human colonic bacteria. Anaerobe. (1997) 3:327–37. doi: 10.1006/anae.1997.0121
47. Kaewtapee C, Krutthai N, Bunchasak C. Effects of supplemental liquid DL-methionine hydroxy analog free acid in diet on growth performance and gastrointestinal functions of piglets. Asian-Australas J Anim Sci. (2016) 29:1166–72. doi: 10.5713/ajas.15.0579
48. Hooda S, Boler BMV, Serao MCR, Brulc JM, Staeger MA, Boileau TW, et al. 454 pyrosequencing reveals a shift in fecal microbiota of healthy adult men consuming polydextrose or soluble corn fiber. J Nutr. (2012) 142:1259–65. doi: 10.3945/jn.112.158766
49. Poll BG, Cheema MU, Pluznick JL. Gut microbial metabolites and blood pressure regulation: Focus on SCFAs and TMAO. Physiology. (2020) 35:275–84. doi: 10.1152/physiol.00004.2020
50. Kelly CJ, Zheng L, Campbell EL, Saeedi B, Scholz CC, Bayless AJ, et al. Crosstalk between microbiota-derived short-chain fatty acids and intestinal epithelial HIF augments tissue barrier function. Cell Host Microbe. (2015) 17:662–71. doi: 10.1016/j.chom.2015.03.005
51. He J, Guo H, Zheng W, Xue Y, Zhao R, Yao W. Heat stress affects fecal microbial and metabolic alterations of primiparous sows during late gestation. J Anim Sci Biotechno. (2019) 10:84. doi: 10.1186/s40104-019-0391-0
52. Vinolo MAR, Rodrigues HG, Nachbar RT, Curi R. Regulation of inflammation by short chain fatty acids. Nutrients. (2011) 3:858–76. doi: 10.3390/nu3100858
53. Yin J, Li Y, Han H, Ma J, Liu G, Wu X, et al. Administration of exogenous melatonin improves the diurnal rhythms of the gut microbiota in mice fed a high-fat diet. Msystems. (2020) 5:e00002-20. doi: 10.1128/mSystems.00002-20
54. Li Y, Ma J, Yao K, Su W, Tan B, Wu X, et al. Circadian rhythms and obesity: Timekeeping governs lipid metabolism. J Pineal Res. (2020) 69:e12682. doi: 10.1111/jpi.12682
55. Wu G, Bazer FW, Dai Z, Li D, Wang J, Wu Z. Amino acid nutrition in animals: protein synthesis and beyond. Annu Rev Anim Biosci. (2014) 2:387–417. doi: 10.1146/annurev-animal-022513-114113
56. Brosnan ME, Brosnan JT. Formate: The neglected member of one-carbon metabolism. Annu Rev Nutr. (2016) 36:369–88. doi: 10.1146/annurev-nutr-071715-050738
57. Gu Z, Li L, Tang S, Liu C, Fu X, Shi Z, et al. Metabolomics reveals that crossbred dairy buffaloes are more thermotolerant than holstein cows under chronic heat stress. J Agr Food Chem. (2018) 66:12889–97. doi: 10.1021/acs.jafc.8b02862
58. Hagenbuch B, Dawson P. The sodium bile salt cotransport family SLC10. Pflugers Archiv. (2004) 447:566–70. doi: 10.1007/s00424-003-1130-z
59. Amaral JD, Viana RJS, Ramalho RM, Steer CJ, Rodrigues CMP. Bile acids: regulation of apoptosis by ursodeoxycholic acid. J Lipid Res. (2009) 50:1721–34. doi: 10.1194/jlr.R900011-JLR200
60. Suzuki Y, Kaneko R, Nomura M, Naito H. Simple and rapid quantitation of 21 bile acids in rat serum and liver by UPLC-MS-MS: Effect of high fat diet on glycine conjugates of rat bile acids. Nagoya J Med Sci. (2013) 75:57–72. doi: 10.1002/lary.23585
61. Shimada T, Nakanishi T, Toyama A, Yamauchi S, Kanzaki A, Fujiwake H, et al. Potential implications for monitoring serum bile acid profiles in circulation with serum proteome for carbon tetrachloride-induced liver injury/regeneration model in mice. J Proteome Res. (2010) 9:4490–500. doi: 10.1021/pr1002388
62. Zhang J, Gao Y, Guo H, Ding Y, Ren W. Comparative metabolome analysis of serum changes in sheep under overgrazing or light grazing conditions. BMC Vet Res. (2019) 15:469. doi: 10.1186/s12917-019-2218-9
Keywords: gilts, metabolic profiles, methyl-donor micronutrient, microbial community, offspring piglets
Citation: He Q, Zou T, Chen J, He J, Jian L, Xie F, You J and Wang Z (2021) Methyl-Donor Micronutrient for Gestating Sows: Effects on Gut Microbiota and Metabolome in Offspring Piglets. Front. Nutr. 8:675640. doi: 10.3389/fnut.2021.675640
Received: 03 March 2021; Accepted: 22 April 2021;
Published: 07 June 2021.
Edited by:
Jie Yin, Hunan Agricultural University, ChinaReviewed by:
Hongkui Wei, Huazhong Agricultural University, ChinaYulan Liu, Polytechnic University, China
Copyright © 2021 He, Zou, Chen, He, Jian, Xie, You and Wang. This is an open-access article distributed under the terms of the Creative Commons Attribution License (CC BY). The use, distribution or reproduction in other forums is permitted, provided the original author(s) and the copyright owner(s) are credited and that the original publication in this journal is cited, in accordance with accepted academic practice. No use, distribution or reproduction is permitted which does not comply with these terms.
*Correspondence: Jinming You, youjinm@163.com; Zirui Wang, wangzirui@jxau.edu.cn