- 1Tea Research Institute, Zhejiang University, Hangzhou, China
- 2Tea Science Society of China, Hangzhou, China
- 3Development Center of Liubao Tea Industry, Cangwu, China
Catechins are a cluster of polyphenolic bioactive components in green tea. Anticarcinogenic effects of tea catechins have been reported since the 1980s, but it has been controversial. The present paper reviews the advances in studies on the anticarcinogenic activities of tea and catechins, including epidemiological evidence and anticarcinogenic mechanism. Tea catechins showed antagonistic effects on many cancers, such as gynecological cancers, digestive tract cancers, incident glioma, liver and gallbladder cancers, lung cancer, etc. The mechanism underlying the anticarcinogenic effects of catechins involves in inhibiting the proliferation and growth of cancer cells, scavenging free radicals, suppressing metastasis of cancer cells, improving immunity, interacting with other anticancer drugs, and regulating signaling pathways. The inconsistent results and their causes are also discussed in this paper.
Introduction
Cancer or carcinoma is a leading contributor to the global disease burden and the number of its deaths is next only to cardiovascular diseases (1). There were 23.6 million cases of various cancers [95% confidence interval (95% CI) = 22.2∼24.9 million] and 10.0 million deaths (95% CI = 9.36∼10.6 million) in 2019 across 204 countries and territories (1). Projections forecasted that the worldwide cancer burden might continue to increase for the next two decades (2–4) and it is supposed to have 28.4 million cancer cases in 2040 (5). It is considered that diet will be an effective pathway for preventing some cancers (6, 7).
Catechins are a cluster of bioactive polyphenolic components in fresh tea leaves or green tea and they are major contributors to the health benefits of tea (6, 8). There are usually four epi-type catechins found in fresh leaves of normal tea cultivars, i.e., (−)-epicatechin (EC), (−)-epigallocatechin (EGC), (−)-epicatechin gallate (ECG), and (−)-epigallocatechin gallate (EGCG). Their isomers, however, are detected in made teas due to heat-induced epimerization from the epi-type catechins in tea manufacture (9, 10). The most abundant individual of catechins in fresh tea leaves is EGCG which is more than 40% of the total content of catechins (8). Many studies showed the anticarcinogenic effects of tea catechins (11–13). However, the effects of tea catechins and tea consumption on the risk of cancers is still inconclusive and controversial (14, 15). Though there were dozens of review papers focusing on this topic in the last decade, the controversial or inconsistent results, which are very important for the future studies, have not been involved. This review not only summarizes the progresses on the anticarcinogenic effects of catechins or tea and underlying mechanism, but also highlights the controversial or inconsistent results and their causes, which will be provide helpful references for future studies.
Original research papers or epidemiological study reports were researched on the Web of Science using key words “catechins (topic) and anticarcinogenic (topic) or anticancer (topic)” and those with no relation to catechins from tea source were excluded.
Epidemiological evidence of anticarcinogenic effects of tea and tea catechins
There have been epidemiological studies showing the relationship of tea consumption to the decreased risk of cancer incidences since the end of the last century. A 9-year study among 8,552 Japanese adults revealed that frequent consumption of green tea in large amounts showed a potentially beneficial effect on cancer prevention. The cancer onset was delayed by 8 years in females, but by 3 years in males who consumed ≥10 cups of green tea daily, compared to those who consumed ≤3 cups daily (16). Meta-analysis including 18 prospective cohort and 25 case-control studies showed a significant inverse association between intake of tea catechins and risk of various cancers, with a relative risk (RR) being 0.935 (95% CI = 0.891∼0.981). Catechins consumption showed significantly protective effect on breast cancer (BC) (RR = 0.885, 95% CI = 0.790∼0.991), rectal cancer (RR = 0.838, 95% CI = 0.733∼0.958), oropharyngeal and laryngeal cancer (RR = 0.759, 95% CI = 0.581∼0.993), and stomach cancer (RR = 0.633, 95% CI = 0.468∼0.858) (17).
Gynecological cancers
Tea drinking is found to be related to the reduced risk of gynecological cancers. A case-control study on Asian-American women in Los Angeles, USA revealed that green tea intake was significantly and negatively correlated to the BC risk (18). Furthermore, a study involving 472 patients with stage I, II, and III BC in Japan showed that green tea consumption was negatively correlated to numbers of axillary lymph node metastases among pre-menopausal patients with stage I and II BC, but positively correlated to the expression of estrogen receptor (ER) and progesterone receptor (PgR) among post-menopausal patients. A 7-year follow-up study on these patients showed that an increase in green tea consumption was negatively correlated to the recurrence of stage I and II BC (p < 0.05), with a recurrence rate being 16.7% among patients who consumed ≥5 cups/day, but being 24.3% among those who consumed ≤4 cups/day. After adjustment for other lifestyle factors, the RR of recurrence among patients with stage I and II BC was 0.564 (95% CI = 0.350∼0.911) (19). In a population-based case-control study involving in 501 BC patients and 594 control subjects among Japanese, Chinese, and Filipino-American women, which was carried out during 1995–1998, showed that the risk of BC decreased with increase in intake of green tea [odds ratio (OR) = 1.00, 95% CI = 0.71 (0.51∼0.99)] in non-tea drinkers (0–85.7 mL/d) and OR = 0.53 (95% CI = 0.35∼0.78) in tea drinkers (>85.7 mL/d) (20). A meta-analysis based on data through literature search in the MEDLINE database from January 1966 to August 2004, which revealed the relationship of black tea or green tea consumption to BC risk among populations from eight countries, showed that high intake of green tea reduced the risk of BC with OR = 0.78 (95% CI = 0.61∼0.98). Black tea consumption showed weakly positive correlation to the risk of BC, with OR = 0.91 (95% CI = 0.84∼0.98), in which the negative correlation was higher in hospital-based studies (OR = 0.77, 95% CI = 0.50∼1.19) than the population-based case-control studies (OR = 0.94, 95% CI = 0.81∼1.09) (21). An investigation on 3,315 women in Singapore showed that mammographic density (PMD) of daily green tea drinkers (19.5%) was significantly lower than that of non-tea drinkers (21.7%, p = 0.002) after the relevant covariates were adjusted, however, the intake of black tea was not associated with the risk of PMD (22). A meta-analysis including 3,323,288 participants showed that heavy tea consumption has a preventing effect against ER-BC, especially in the post-menopausal women. Therefore, tea was considered to be a potentially useful dietary protectant for preventing BC and a recommended dose was ≥5 cups/day (23).
Ovarian cancer risk was found to be associated with tea drinking. Black tea consumption showed linearly suppressive effects on the risk of ovarian cancer (p = 0.03), in which 30% decline in the risk was observed (adjusted OR = 0.70, 95% CI = 0.51∼0.97) among those who consumed ≥2 cups daily (24). Green tea drinking was found significantly to decrease the risk of ovarian tumors (OR = 0.81, 95% CI = 0.73∼0.89, p < 0.0001) in a meta-analysis study including 3,842 cases and 5,271 control cases retrieved from Wanfang, CNKI, CBMdisc, EMbase, and PubMed databases during 2000–2010 (25).
Tea drinking was found to be beneficial to endometrial cancer. A meta-analysis involving 6,797 cases of endometrial cancer and 858,780 normal controls showed that the pooled RR of endometrial cancer for the highest consumption of tea was 0.99 (95% CI = 0.94∼1.04, p = 0.005), compared with the lowest level. Further study showed that the pooled RR was 0.83 (95% CI = 0.73∼0.95) for case-control study but 1.02 (95% CI = 0.96∼1.08) for cohort study, in terms of study design. For various regions, however, the pooled RR was 0.80 (95% CI = 0.69∼0.93) for Asia, 1.06 (95% CI = 0.94∼1.20) for USA/Canada and 1.01 (95% CI = 0.95∼1.08) for Europe. For different kinds of tea, inverse associations of reduced risk of endometrial cancer were found with black tea (RR = 0.65, 95% CI = 0.46∼0.92) and green tea (RR = 0.73, 95% CI = 0.64∼0.84), respectively (26).
Digestive tract cancers
Epidemiological investigations showed that tea drinking was correlated with a reduced risk of digestive tract cancers. An 8-year follow-up cohort study on association between tea consumption and cancer incidence involving 35,369 post-menopausal women in Iowa, USA showed that increasing frequency of tea drinking was inversely associated with digestive tract cancer (p = 0.04, RR = 0.68, 95% CI = 0.47∼0.98) for females who drank ≥2 cups (474 mL/cup) daily, in comparison with the women who occasionally or never drank tea (27). A population-based case-control study including 931 colon cancer, 884 rectum cancer, 451 pancreas cancer patients, and 1,552 control residents which was conducted in Shanghai, China during 1990–1993, showed that the amount of green tea consumption was inversely associated with colorectal and pancreatic cancers (28). Green tea drinking reduced by 39, 78, and 81% in the risk of esophageal cancer, liver cancer and gastric cancer were, respectively observed among alcohol drinkers according to a population-based case-control study conducted in Taixing, China (29). Those with a high level of pre-diagnostic urinary epigallocatechin (EGC) (a component of tea catechins) had a lower risk of colon cancer. The ORs for colon cancer in populations with the lowest, intermediate, and highest tertile of EGC detected were 0.64 (95% CI = 0.33∼1.24), 0.60 (95% CI = 0.30∼1.20), and 0.40 (95% CI = 0.19∼0.83) respectively (p < 0.02) in comparison to those without EGC detected. Methylated EGC showed a similar effect on colon cancer (30). A 6-year population-based prospective cohort study involving 14,001 elderly inhabitants with ages ranging from 65 to 84 years in Shizuoka, Japan showed that green tea drinking was negatively correlated with colorectal cancer (CRC) mortality in a mediate dose dependent manner, with hazard ratio (HR) = 0.24 (95% CI = 0.14∼0.40) for total participants, HR = 0.30 (95% CI = 0.15∼0.61) for men, and HR = 0.18 (95% CI = 0.08∼0.40) for women who consumed ≥7 cups/day, compared to those who drank more than one cup daily (31). A pooled analysis of six cohort studies including 219,080 subjects, among which 3,577 cases were gastric cancer, showed a significant risk reduction for gastric cancer for women with consumption of more than 5 cups daily (multivariate-adjusted pooled HR = 0.79, 95% CI = 0.65∼0.96), but no significant risk reduction for gastric cancer in men (32).
Green tea intake was shown to be negatively correlated with stomach cancer risk (RR/OR = 0.86, 95% CI = 0.74∼1.00). The group with green tea consumption >5 cups/day showed significantly preventive effect on stomach cancer (RR/OR = 0.68, 95% CI = 0.53∼0.87) (33). An 11-year follow-up study involving 1,255 persons suffering from digestive system cancers including esophagus, stomach, liver, pancreas, colorectal and gallbladder/bile duct cancers from 69,310 non-alcohol-drinking and non-smoking women in Shanghai, China showed that normal tea drinking (more than three times per week for more than 6 months) was showed suppressive effects on the overall digestive system cancers (HR = 0.86, 95% CI = 0.74∼0.98, p = 0.01). In comparison with women who never drank tea, and the risk decreased with increase in duration and amount of tea drinking (p < 0.01) (34). A cohort study involving a total of 65,042 Japanese residents aging from 40 to 79 years revealed that green tea consumption showed a protective effect against hematologic neoplasms, with multivariate HR = 0.65 (95% CI = 0.42∼1.00) for leukemia among those who consumed 2 cups of green tea daily or less, 0.73 (95% CI = 0.47∼1.13) for those who drank 3–4 cups of green tea daily, and 0.63 (95% CI = 0.42∼0.96) for those who drank 5 cups of green tea daily (35). An investigation involving 7,355 eligible subjects who were classified into polyp-free, low-risk colorectal adenomas and high-risk colorectal adenomas based on health check-ups with colonoscopies showed that tea drinking was inversely associated with low-risk colorectal adenomas. A larger cumulative dose (≥42 cup-year) was negatively associated with high-risk colorectal adenomas, especially adenomas with villous-rich pathology and when three or more adenomas were present (36).
Incident glioma
Tea consumption was revealed to be negatively correlated to the risk of glioblastoma (HR = 0.93, 95% CI = 0.89∼0.98) in a prospective cohort study involving 379,259 UK Biobank participants among which 487 were incident glioma cases. Consuming ≥4 cups/day was decreased the risk of glioma in comparison of no tea consumption (HR = 0.69, 95% CI = 0.51∼0.94) (37). A study on relationship of recent (up to 12 years) or average long-term (up to 30 years) dietary flavonoid intake to the risks of incident glioma based on data from the male Health Professionals Follow-Up Study (1986–2014, n = 49,885), Nurses’ Health Study II (1991–2017, n = 95,228) and the female Nurses’ Health Study (1984–2014, n = 81,688) showed that long-term tea catechins intake was associated with reduced risks of glioma in pooled analysis with comparison of the highest quintile to the lowest quintile of tea consumption (HR = 0.76, 95% CI = 0.57∼1.01, p = 0.04). The association with recent intake was weaker (38). Meta-regression analysis showed that higher tea consumption was related to a reduced glioma risk (RR = 0.84, 95% CI = 0.71∼0.98, p = 0.030), and sensitivity study through removing case-control studies revealed that more consumption of tea was associated with lower risk of glioma (RR = 0.81, 95% CI = 0.70∼0.93, p = 0.004). Daily 1 cup of tea reduced the glioma risk by 3% (RR = 0.97, 95% CI = 0.94∼1.00, p = 0.048) (39).
Liver and gallbladder cancers
A population-based case-control study including 1,037 cases with biliary stones, 627 incident cases with biliary tract cancer (BTC) and 959 randomly selected controls of biliary tract disease in Shanghai, China, showed that female tea drinkers have significantly decreased gallbladder cancer risk (OR = 0.56, 95% CI = 0.38∼0.83) and biliary stone risks (OR = 0.73, 95% CI = 0.54∼0.98). However, these risk assessments among male tea drinkers who were more likely to be cigarette smokers were not significantly lower than the non-tea drinkers (40). A 9-year follow-up cohort study involving in 41,761 Ohsaki residents with ages from 40- to 79-year old in Japan showed that green tea drinking was negatively correlated to the liver cancer risk. The multivariate-adjusted HRs for the risk of liver cancer among women were 0.68 (95% CI = 0.35∼1.31) for those who drank 2 cups or less daily, 0.79 (95% CI = 0.44∼1.44) for those who drank 3–4 cups daily, and 0.50 (95% CI = 0.27∼0.90) for those who drank >5 cups daily, compared with the group drinking less than 1 cup/day. The corresponding HRs among men were 0.83 (95% CI = 0.53∼1.30) for 1–2 cups/day, 1.11 (95% CI = 0.73∼1.68) for 3–4 cups/day, and 0.63 (95% CI = 0.41∼0.98) for >5 cups/day (41). Studies on tea consumption in relation to primary liver cancer from 1979 to 2009 in China, which included 13 epidemiological investigations consisting of seven prospective cohort and six case-control studies, showed that consumption of polyphenols abundant tea was negatively correlated with the risk of primary hepatic cell carcinoma (HCC) (RR = 0.77, 95% CI = 0.57∼1.03). Tea drinking showed preventive effects on the development of primary liver HCC both among women (RR = 0.54, 95% CI = 0.37∼0.79) and men (RR = 0.86, 95% CI = 0.77∼0.95) (42). Larger quantities and longer duration of green tea drinking were negatively correlated with primary HCC, among which individuals who consumed green tea more than 30 years showed the lowest risk (adjusted OR = 0.44, 95% CI = 0.19∼0.96) in comparison with non-tea drinkers (43).
Lung cancer
Heavy drinking of tea (more than 2 cups per day) was found to be negatively correlated with the risk of lung cancer in a case-control study including 428 hospitalized controls and 427 lung cancer cases in Uruguay (RR = 0.34, 95% CI = 0.14∼0.84) (44). Green tea drinking was found to have a reduced primary lung cancer risk in a case-control study including 649 female patients with primary lung cancer based on the data from Shanghai Cancer Registry from February 1992 to January 1994. However, the effect was dependent on smoking. The risks reduced with the increase in green tea consumption among non-smoking women (OR = 0.65, 95% CI = 0.45∼0.93), but little association was found among female smokers (OR = 0.94, 95% CI = 0.40∼2.22) (45). An investigation on the relationship of diet and physical activity to the risk of lung cancer in the Czechia revealed that black tea drinking had preventive effect on lung cancer among non-smokers (OR = 0.67, 95% CI = 0.46∼0.99) (46). The frequency of black tea drinking (daily or several times per week) showed a reduced risk of lung cancer female non-smokers (OR = 0.65, 95% CI = 0.43∼0.99) (47).
Other cancers
Consumption of hot black tea was found to significantly reduce skin squamous cell carcinoma (SCC) risk (OR = 0.22; 95% CI = 0.10∼0.51) in a population-based case-control study conducted by the Southeastern Arizona Health Study (SEAHS) from 1994 to 1996 (48). Prostate cancer risk was significantly decreased with an increase in quantity, duration and frequency of green tea drinking in a case-control study including 130 incidental prostate cancer cases confirmed histologically and 274 cases without cancer matched by age in southeast China (49). A study in Italy revealed that orally administrating green tea catechins (600 mg/day for 1 year) showed cancer chemoprevention effect on prostate cancer among patients who were at high risk to develop prostate cancer and had pre-malignant lesions (50). A study based on 3 nutrient-specific databases developed by the United States Department of Agriculture which included 466 cases of non-Hodgkin lymphoma (NHL) and 390 controls showed that higher intakes of green tea components including epicatechin, flavonols, proanthocyanidins and anthocyanidins significantly reduced NHL risk (51).
It was found in a case-control study including 107 leukemia cases and 110 orthopedic controls in China mainland that the risk of leukemia decreased with the increase in frequency, quantity and duration of green tea consumption (52). An investigation including 252 cases and 637 controls in China Taiwan revealed that green tea consumption showed significantly negative correlation with leukemia risk among persons from 16- to 29-year-old (OR = 0.49, 95% CI = 0.27∼0.91). The adjusted OR for heavy tea drinking group (more than 550 cups annually) was 0.47 (95% CI = 0.23∼0.97), compared with non-tea drinking group. Large amounts of tea catechins intake showed a reduced risk of leukemia (OR = 0.49, 95% CI = 0.27∼0.91) after adjusting for smoking status and medical irradiation exposure (53). A prospective UK Biobank cohort study involving more than 500,000 participants with ages ranging from 38 to 73 years at enrollment (2006–2010) revealed that tea drinking showed significantly negative correlation to cancer mortality in both male and female (54).
Anticarcinogenic mechanism of tea catechins
Inhibition of cancer cell proliferation and growth
Major components of green tea polyphenols (GTPs) are catechins which include at least eight compounds, i.e., EC, ECG, EGC, EGCG, (+)-catechin-3-gallate (CG), (+)-gallocatechin-3-gallate (GCG), (+)-gallocatechin (GC), and (+)-catechin (GC). The content of EGCG accounts for more than 40% of total content of catechins and so it is the most abundant one (8–10). The research on anticarcinogenic activity of catechins has started since the 1980s (55). EGCG showed a dose-dependent inhibitory effect on human papillomavirus type 16 (HPV-16) induced cervical cancer cell CaSki, in which the inhibitory dose (ID) was 35 μM approximately. Cell cycles were arrested at the G1 phase when incubated with 35 μM EGCG and EGCG-induced apoptosis was occurred after incubation at 100 μM EGCG for 24 h (56). When non-small cell lung cancer A549 cells were incubated with catechin at 600 μmol/L for 24 h, inhibition rate of the cell proliferation was 19.76% (57). The inhibitory effects of catechins on proliferation of cancer cells depend on the components of catechins and the types of cancer cells. For the HSC-2 carcinoma cells, ECG, CG, and EGCG were grouped as highly toxic, EGC as moderately toxic, and C and EC as least toxic. For the HGF-2 fibroblasts, ECG and CG were grouped as highly toxic, EGCG as moderately toxic, and EGC, C, and EC as least toxic (58).
The mechanism inhibiting cancer cell proliferation involves in several aspects, such as cancer cell growth arrest and cancer cell death (Figure 1). Catechin inhibits A549 cells by regulating its cell cycle arrest, increasing the expressions of p21 and p27 and inhibiting the expressions of p-AKT (phosphorylated protein kinase B) and cyclin E1 in a dose-dependent manner in the cancer cells, which also contributed to the suppression of cancer cell proliferation (57). EGCG can trigger cell growth arrest pathways at the G1 stage of cell cycle by regulating p27/KIP1, p21/WAF1/CIP1, cdk4, cdk6, and cyclin D1 (58, 59). Catechin pyrogallol inhibits G2-M transition of human lung cancer cells in cell cycling, resulting in tumor growth suppression (60). In BC T47D cell line, catechin activates the phosphorylation of p38 and JNK/SAPK, and the former suppresses the phosphorylation of cell division control protein 2 (CDC2) and regulates the expression of cyclin dependent kinase (CDK), cyclin A and cyclin B1 proteins, leading to G2 arrest (61). EGCG inhibited the proliferation of human lung cancer cells through targeting the epidermal growth factor receptor (EGFR) signaling pathway (62). EGCG displays anticarcinogenic effects in human BTC cell lines by activating caspase and inducing cell arrest in the sub-G1 phase in cell cycling (63).
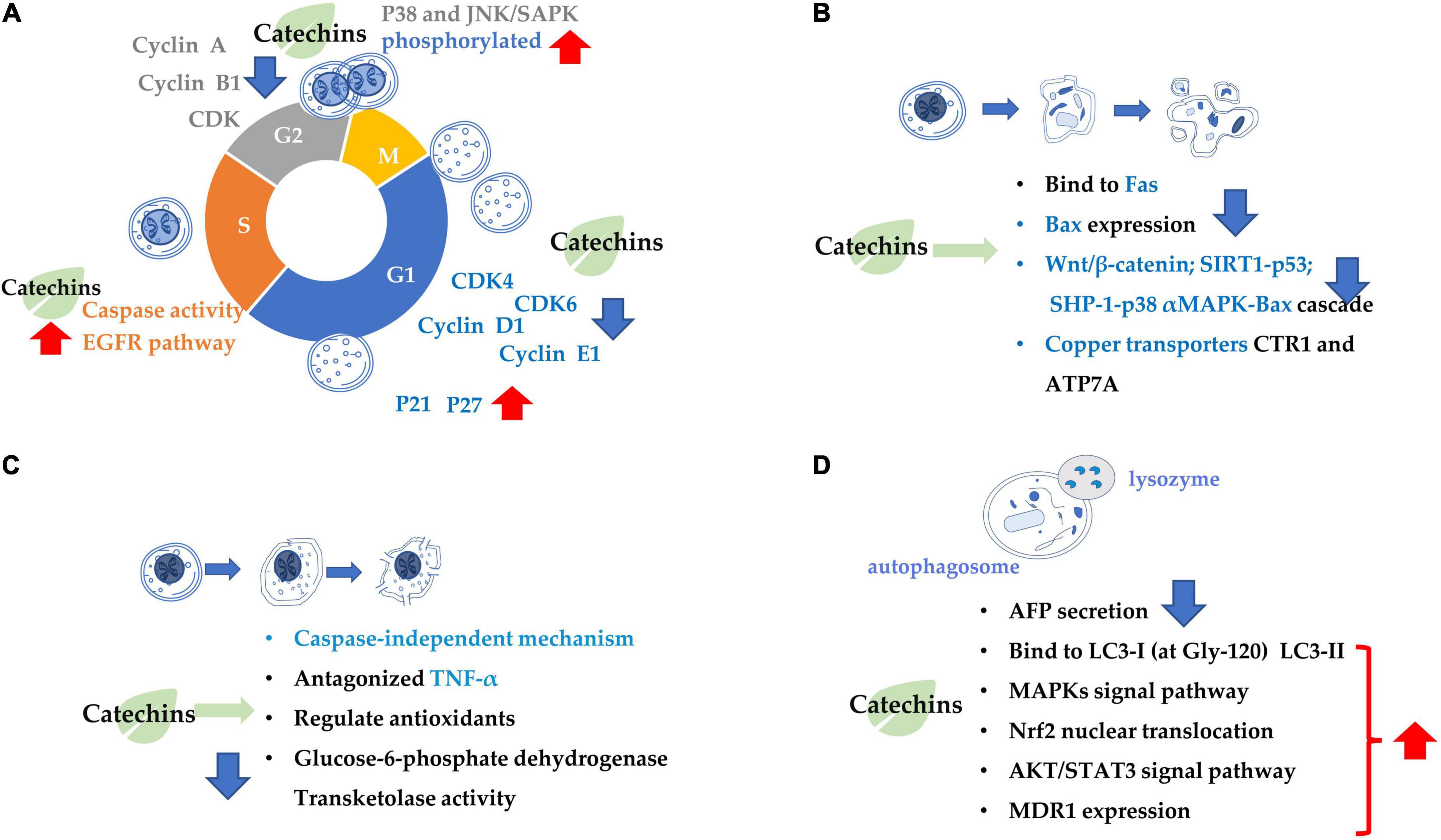
Figure 1. Summary of the target of tea catechins inhibiting cancer cell proliferation and growth. This is mainly achieved through four processes: (A) cell cycle arrest; (B) cell apoptosis; (C) cell necrosis; and (D) cell autophagy. Blue arrows indicate downregulation and red arrows indicate upregulation.
Catechins anticarcinogenic effects by inducing apoptosis of various types of tumor cells. EGCG inhibited the growth and induced apoptosis (programmed cell death) of human stomach cancer cell line KATO III (64). EGCG triggers the Fas-mediated apoptosis in LNCaP cells by reducing Fas activity, presumably on the cell surface (65). EGCG induces apoptosis in human prostate cancer cells via changing nuclear morphology and DNA fragmentation (66). The EGCG-induced apoptosis and inhibition of EGCG-mediated Bcl2-associated x protein (Bax) expression were partially blocked by pre-treatment with PD169316 [a p38 mitogen-activated protein kinase (MAPK) inhibitor] (67). Similarly, the EGCG-induced apoptosis, and inhibition of EGCG-mediated expression of p-p38 alpha MAPK and Bax were partially blocked by pre-treatment with SHP-1 inhibitor NSC87877. Therefore, it is considered that EGCG induced apoptosis in NB4 cells via the SHP-1-p38 alpha MAPK-Bax cascade (68). EGCG promoted the growth inhibition and apoptosis of nasopharyngeal carcinoma (NPC) cell lines CNE-2 and 5-8F through inhibition of the SIRT1-p53 signaling pathway (69). EGCG induced inhibition of growth migration, invasion and apoptosis of human osteosarcoma OS cells through regulating the Wnt/beta-catenin pathway (70). Copper dynamics and mobilization played an important role in the EGCG-induced cancer cell apoptosis through regulating cellular copper transporters CTR1 and ATP7A (71).
The necrosis is thought to be a result of ATP depletion to a level unsuitable for cell survival (72). EGCG induced necrosis-like cell apoptosis through a caspase-independent mechanism in C2F8, K562, and CML cell lines. Furthermore, EGCG promoted cell death induced by imatinib (p < 0.01), leading to increased K562 cell death and suppressing the imatinib-resistant viability of cell line K562 (p < 0.01) (72). Green tea extract induced necrosis of cancer cell lines K-562U-937, Clone E6-1, and Jurkat, with half maximal inhibitory concentrations, with IC50 = 88 ± 1.89, 98 ± 1.96, and 205 ± 2.23 μg/mL, respectively (73). Topical (+)-catechin prevents DMBA/TPA-induced carcinoma of the skin squamous cells by modulating antioxidants and tumor necrosis factor α (TNF-α) in BALB/c mice (74). ECG reduced tumor viability and induced necrosis in HT29 cells by inactivating glucose-6-phosphate dehydrogenase and transketolase, the key pentose phosphate pathway enzymes (75).
Combination treatment of EGCG with a low energy ultrasound and a low strength pulsed electric field (PEF) for 72 h induced autophagy of human pancreatic cancer cell PANC-1 (76). Autophagy and apoptosis of glioblastoma cells were strongly induced by 500 μM EGCG treatment (77). Also, EGCG induced autophagy by activating light chain 3 (LC3) and generating reactive oxygen species (ROS) in tumor cells. By enhancing autophagy, the sensitivity of cancer cells had been improved, that’s how EGCG can improve the efficacy of anti-cancer drugs (78).
(−)-Epigallocatechin gallate is crucial factor in governing secretion of α-fetal protein (AFP) modulating autophagic activity of cells HepG(2). EGCG reduced AFP secretion and simultaneously induce AFP aggregation in human HCC HepG(2) cells. EGCG-stimulated autophagy induces the degradation of AFP aggregates in HepG(2) cells, and it directly interacted with protein LC3-I, leading to exposure of the eminent Gly-120 site of LC3-I to the other pivotal binding partners such as promoting the synthesis of LC3-II and 1,2-distearoyl-sn-glycero-3-phosphoethanolamine (79). EGCG induced cisplatin-resistant oral cancer CAR cell autophagy in a time- and concentration-dependent manner, during which the AKT/signal transducer and activator of transcription 3 (STAT3) pathway was stimulated and the expression of multidrug resistance 1 (MDR1) was dose-dependently inhibited, suggesting the alteration of AKT/STAT3 signaling and downregulation of MDR1 were partially responsible for the EGCG-induced CAR cell autophagy and apoptosis (80). EGCG promoted nuclear factor-erythroid 2-related factor 2 nuclear translocation and autophagy, enhanced the sensitivity of CRC cells to radiation and inhibited CRC cell proliferation (81).
Antioxidant and free radical scavenging
Reactive oxygen species is a crucial signaling substance that play important roles in carcinogenesis. ROS is a group of highly sensitive molecules containing oxygen which are continually generated by metabolizing organelles such as endoplasmic reticulum, peroxisomes and mitochondria (82). ROS appears to elicit both pro-malignant and anti-malignant effects with an abnormal spectrum of actions. Excessive accumulation of ROS in cells activates the signaling pathways precipitating DNA damage or inducing mutagenesis, resulting in carcinogenicity. Regulation of ROS levels in cells seems to be an encouraging therapeutic measure, especially for screening anticarcinogenic effects of natural products with strong antioxidant properties (83).
Antioxidants are substances that protect interior molecules from oxidative damages (84). Catechins play a role as reducing agent antioxidants in many reactions owing to their abundant hydroxyl groups (85), and have been attracting enormous attention as possible compounds to be used in prevention and treatment of ROS-targeted cancers. The mechanisms underlying the anticarcinogenic potential of catechins include chelating trace metals, inhibiting oxidases responsible for producing the superoxide anion, scavenging ROS, and activating antioxidant enzymes (86, 87). During the DPPH scavenging behavior, an electron on B-ring in catechins molecules is oxidized by DPPH radical, which generates a catechin phenoxyl radical to be tautomerized to a corresponding o-quinone, then comes the nucleophilic attack by the reactive C-8 or C-6 carbon of other catechins molecules in a Michael-type addition reaction to a quinone on B-ring. Both B-ring and A-ring, but not the gallate moiety, can be the antioxidant site of catechins (87–89). The oligomers of C, EC, and resveratrol have shown anticarcinogenic properties on T24 human urinary bladder cancer cells due to scavenge ROS and chelate metal ions Fe2+ and Cu2+, the antioxidant capacity of which is significantly higher than their preceding monomers (90). In vitro study showed catechin increased manganese superoxide dismutase (MnSOD) gene expression after being incubated with pheochromocytoma cells (PC-12) for 2 days, which can lead to growth inhibition of the cancer cells (91). EGCG can also covalently interact with cysteinyl thiol residues in protein molecules via autoxidation, resulting in functional modulation which may be related to the anticancer effects (92).
There was evidence showing EGCG attenuates DNA damage mediated by ROS in pre-malignant cells, resulting in inhibition of tumor initiation (93). These effects were confirmed in cells hepatocytes, lymphocytes, and colonocytes (94, 95). Carcinogen-induced activation of NOX1/ROS is a signaling pathway in malignant and pre-malignant cells, and treating these cells using EGCG markedly suppressed NOX1/ROS signaling and interrupted the carcinogenesis (96). ROS inhibited MAPK phosphatases, which negatively regulate MAPK, it can be speculated that decreasing ROS levels and subsequent phosphorylation blocking of extracellular signal-regulated ERK1/2 (kinases 1 and 2) contribute partially to the antioxidation activity of EGCG in treating and preventing fibrosarcoma (97).
Inhibition of metastasis of cancer cells
The invasion and metastasis of cancer cells are the main reasons for cancer recurrence and treatment failure. The metastatic process of cancer cells includes loss of cell adhesion, increased cell motility and invasiveness, entry into the blood circulation, and spread to distant tissues (98). Several in vitro cell experiments and in vivo animal experiments have shown that catechins, especially EGCG, could negatively regulate these steps to effectively suppressed the metastasis and invasion of various cancer cells (Figure 2). Specifically, they included inhibiting metastasis by regulating proteolytic enzyme activity, inhibiting the epithelial-mesenchymal transition (EMT) process, and inhibiting the formation of blood vessels or lymphatic vessels.
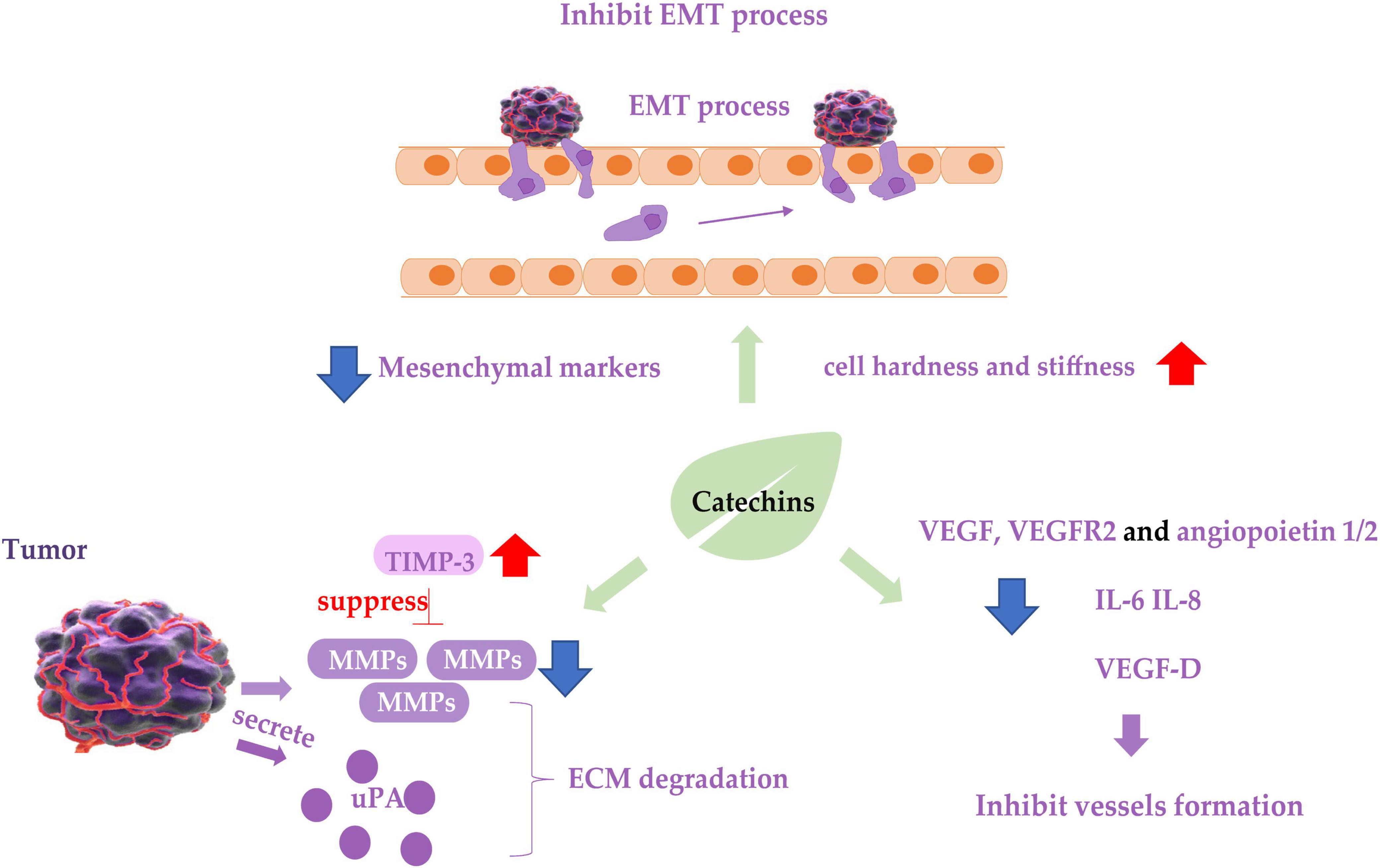
Figure 2. Diagram of catechins inhibiting cancer cell metastasis. Blue arrows indicate downregulation and red arrows indicate upregulation. EMT, epithelial-mesenchymal transition process; TIMP-3, tissue inhibitor of metalloproteinase-3; MMP, matrix metalloproteinases; ECM, extracellular matrix; uPA, urokinase plasminogen activator; VEGF, vascular endothelial growth factor.
The anti-metastatic activity of catechins has been confirmed by in vivo experiments in mouse models. Different doses of EGCG given by gavage administration inhibited liver and lung metastasis of colon tumors implanted orthotopically in the cecum of nude mice to different degrees, high-dose EGCG had a potent inhibitory effect (99). A study using an in vivo mouse model of lung metastasis showed that EGCG treatment significantly reduced the size and number of metastatic nodules in the lungs (100).
Matrix metalloproteinases (MMPs) degrade various protein components in the extracellular matrix (ECM) and inhibit the histological barrier of tumor cell invasion, which plays a key role in tumor invasion and metastasis. Studies on renal carcinoma cells 786-0 and ACHN (101), bladder cancer cell SW780 (102), oral cancer cell OC2 (103), three types of NPC cancer cells (104), hepatocellular carcinoma cell LM6 (105), fibrosarcoma cell HT1080 (106), lung cancer cell CL1-5 (107) showed that EGCG treatment downregulated mRNA or protein expression of MMP-2 or MMP-9, and decreased the ability of the cancer cells to metastasize. Tissue inhibitor of metalloproteinase-3 (TIMP-3) downregulates MMPs activity. Abnormal epigenetic silencing of the TIMP-3 has been associated with the carcinogenesis and metastasis of BC. GTPs or EGCG mediated epigenetic induction of TIMP-3 activity and played a crucial role in blocking invasiveness and gelatinolytic activity of MMP-9 and MMP-2 in BC cells (108). The same effect was found in polycystic ovary syndrome (PCOS) mice treated with oolong tea (109).
Urokinase plasminogen activator (uPA) and its inhibitor plasminogen activator inhibitor-1 (PAI-1) are also associated with ECM degradation and are considered biomarkers for metastasis of cancer cells. GTPs inhibited the uPA secretion and suppressed the metastatic behavior of BC cell malondialdehyde (MDA)-MB-231 by inhibiting activator protein-1 (AP-1) and nuclear factor κB (NF-κB) (110), in which an EGCG derivative 3e was able to inhibit the expression of both uPA and PAI-1, resulting in inhibition of the cancer cell metastasis (111). The inhibitive effect of EGCG on the metastasis of prostate cancer cell LNCaP (112) and the above-mentioned oral cancer cell OC2 (103) is also involved in the inhibition of uPA expression.
Epithelial-mesenchymal transition of cancer cells is considered a prerequisite for the acquisition of an invasive/migratory phenotype and subsequent metastasis. EGCG inhibited the in vitro proliferation, migration, and invasion of pancreatic cancer cells, and suppressed mouse xenograft pancreatic cancer in vivo. EGCG halted the “Cadherin switch” and suppressed the expression of mesenchymal markers vimentin and β-catenin (113). Similarly, EGCG suppressed the expression of vimentin in non-small cell lung cancer H1299 cells (114), decreased TGF-β1-induced EMT in anaplastic thyroid carcinoma 8505C cells (115), downregulated the expression of EMT phenotypes of cancer stem cells (CSCs) (116), and inhibited these cancer cells invasion and migration. Catechins containing galloyl moiety, or gallated catechins (such as EGCG, GCG, ECG, and CG) potently suppressed the EMT and migration of ovarian cancer cell ES-2 induced by TGF-β (117, 118), indicating they play a role in inhibiting the metastasis of cancer cells by regulating EMT markers and inhibiting EMT. In addition to the molecular phenotypes of EMT mentioned above, cell motility and cell stiffness are considered to be the mechanical phenotypes of EMT and are also closely related to the metastatic activity of cancer cells. Lower stiffness of cancer cells is connected with stronger metastatic potential in many types of cancers. EGCG and green tea extract increased the mean level of Young’s modulus of cancer cells, resulting in increased cell hardness and stiffness and enhanced inhibition of cell motility (118, 119), leading to the suppression of cancer cell metastasis.
Cancer cell metastasis requires angiogenesis and lymphangiogenesis, which involve hematogenous and lymphatic metastasis of cancer cells, respectively. The inhibitory effect of EGCG in synergy with TNF-related apoptosis-inducing ligand (TRAIL) on migration and invasion of prostate cancer LNCaP cells was achieved by inhibiting the protein expression of vascular endothelial growth factor (VEGF) and angiopoietin 1 and 2 (112). VEGF-D is one of the major lymphangiogenic secretory factors, and it induced lymphatic invasion and metastatic spread of cancer cells (119). EGCG treatment reduced VEGF-D secretion in inflammatory breast cancer cells SUM-149 and SUM-190, resulting in reduction of lymphatic endothelial cell migration and tube formation, leading to inhibition of metastasis of cancer cells (120). EGCG has also been shown to reduce the expression of vascular endothelial growth factor receptor 2 (VEGFR2) in CRC cell line RKO (121). Interleukin IL-6 and IL-8 are confirmed to promote angiogenesis in tumor tissues, and treatment of tumor-bearing mice using EGCG suppressed the expression of IL-6 and IL-8 in a concentration-dependent manner, suggesting that IL-6 and IL-8 might mediate the anti-metastatic activity of EGCG (122). EGCG inhibited the angiogenesis of human thyroid cancer xenograft tumors in nude mice (123).
Regulation of immunity
The immune response to abnormal cells in the body is crucial for maintaining homeostasis. The development of cancer usually triggers a series of immune responses. Tumors can escape immune system attack and migrate by regulating the production of cytokines. Therefore, bioactive substances that target the immune system often have unexpected effects on cancer control, among which catechins are included. Meanwhile, catechins have a significant regulatory effect on multiple sites in the immune system, which has an innate advantage in tumor immunotherapy (Figure 3).
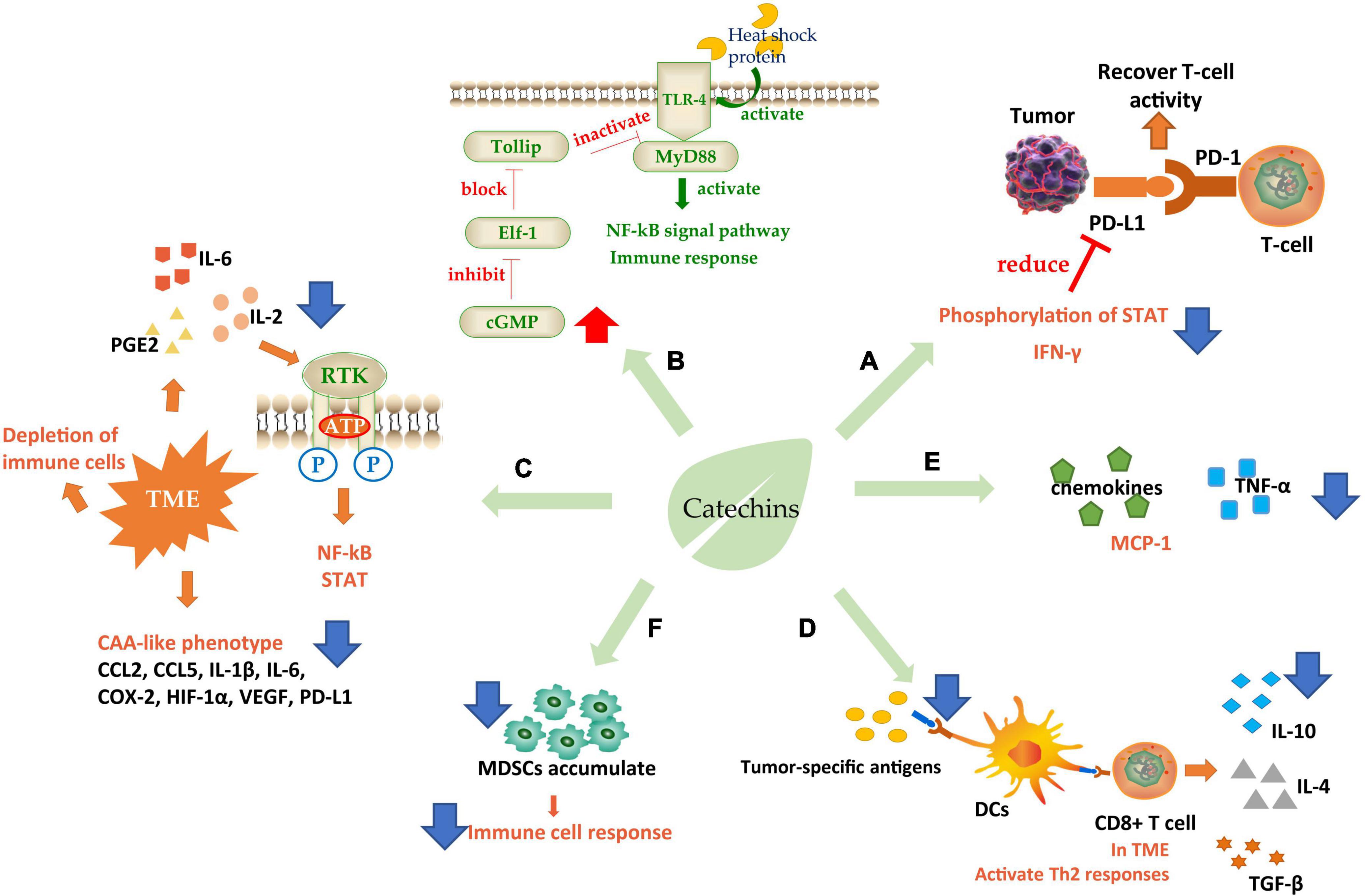
Figure 3. Schematic diagram of catechins regulating immunity. (A) Increase immune checkpoint function; (B) interaction with TLR-4; (C) ameliorate TME; (D,E) regulates immune cell response; (F) regulated immune cytokines. TLR-4, toll-like receptor 4; TME, tumor microenvironment; DCs, dendritic cells; CAA, cancer-associated adipocyte; MDSCs, myeloid-derived suppressor cells. Blue arrows indicate downregulation and red arrows indicate upregulation.
The attack on immune checkpoint is one of the main strategies of tumor cells to escape. The immune checkpoint consists of programmed death factor 1 (PD-1), a receptor molecule secreted by T-cells, and programmed death factor ligand (PD-L1) secreted by tumor cells on the cell membrane. The binding of PD-1/PD-L1 can inhibit the activity of cytotoxic T-cells (CTLs) and even cause T-cell failure, thus protecting tumor cells from immune system attack (124). PD-L1 was induced by epidermal growth factor (EGF) and interferon γ (IFN-γ). Studies were showing that EGCG could regulate the expression of PD-L1 by inhibiting the phosphorylation of STAT and preventing the Janus kinase (JAK)-STAT signaling pathway from activating the transcription factor of PD-L1/PD-L2 (125). PD-L1 mRNA level can also be controlled through the EGFR-Akt signaling pathway (124). IFN-γ is an important factor to stimulate cell expression of PD-L1, so the inhibition of EGCG on IFN-γ could effectively reduce PD-L1 (126). These effects eventually relieve the inhibition of T-cells and improve their attack effect on tumors (Figure 3A).
In addition to PD-1, toll-like receptor 4 (TLR-4) is also a catechin-acting receptor molecule, which may be a target for inhibiting chronic inflammation. Studies have shown that 20% of cancer-related deaths were directly due to TLR-induced cancer cachexia, in which cancer cells released heat shock proteins that acted as TLR-4 agonists in macrophages, skeletal muscle, and fat cells, causing downstream signal transduction. EGCG could effectively downregulate the TLR-4 signal pathway (127). Toll interaction protein (Tollip) is a strong suppressor of this signaling pathway and can directly interact with the ligand IL-1R or TLR4 to inhibit the TLR-induced immune response and negatively regulate signal transduction. Elf-1 is the blocking protein that can inhibit Tollip expression. EGCG achieved the inhibitory regulation of TLR by increasing the level of cGMP in PP2A/cGMP pathway in macrophages to inhibit Elf-1 expression (128). It has also been found that EGCG could inhibit TLR-4/MyD88-mediated NF-κB activation by downregulating both mRNA and protein levels of MyD88 (129) (Figure 3B).
There is an abnormal tumor microenvironment (TME) in cancer patients that causes different immune responses. This low-grade inflammatory state, activated under long-term, low-dose stimulation of specific antigens, often leads to the depletion of immune cells and induces immune tolerance of cancer cells (130). Therefore, some inflammatory factors such as interleukin-2 (IL-2), interleukin-6 (IL-6), and PGE2 are needed to initiate immunosuppression, antagonize this low-grade inflammation and improve the attack of lymphocytes on tumors. EGCG could effectively inhibit inflammation by interrupting the binding of pro-inflammatory factors to receptors and blocking signal transduction such as NF-κB and STAT (131). And the cancer-associated adipocyte (CAA)-like phenotype generated by cells in human triple-negative breast cancer (TNBC) in response to TME. CAA induced cells to secrete many pro-inflammatory factors including CCL2, CCL5, IL-1β, IL-6, and some immune modulators such as COX-2, HIF-1α, VEGF, and PD-L1, which caused carcinogenic through pro-inflammatory effects. EGCG could effectively ameliorate CAA by inhibiting the expression of CAA-related genes and blocking the activation of Smad2 and NF-κB, to alleviate these pro-inflammatory factors and further induces cancer progression (132) (Figure 3C).
Another target of catechins is to regulate immune cell response. First, dendritic cells (DCs), the largest antigen-presenting cells in the immune system, recognize tumor-specific antigens and present them to T-cells (Figure 3D). T-cells initiate a series of responses, typically a Th1 response. Th1-polarized CD4+ T-cells secreted IL-2, IFN-γ, and TNF-α. Activated by IFN-γ and TLRs, macrophages transformed into M1-like macrophages, which can secrete ROS, TNF-α, IL-12, and Nitric oxide (NO) to kill the abnormal cells. At the same time, the cytokines secreted by CD4+ T could further enhance the cytotoxic activity of macrophages and the antigen presentation function of DCs. However, in the TME, Th1 responses were converted to Th2 responses. In this case, Th2 cells secreted IL-4 and IL-10, meanwhile, TGF-β was expressed in large quantities. Simultaneously Foxp3+ B regulatory (Breg) cells and T regulatory (Treg) cells were recruited to initiate humoral immunity. At the same time, m2-polarized macrophages participated in the response to secrete VEGF, IL-10, and TGF-β, which promotes angiogenesis and migration of cancer cells (133, 134). A clinical grade catechin mixture, Polyphenon E, containing about 50% EGCG interacts with the 67 kD laminin receptor (67LR) and promotes the release of granulocyte colony-stimulating factor (G-CSF). The G-CSF then induces the molecular and phenotypic maturation of myeloid-derived suppressor cells (MDSCs) and suppresses their migratory capacity, leading to markedly suppression of their immunosuppressive functions. It was considered that the Polyphenon E could potentially be beneficial to cancer patients through antagonizing cells which interfere with immunotherapy induced antitumor immune responses (135).
(−)-Epigallocatechin gallate plays an efficient regulatory role in this process (Figures 3D,F). EGCG to downregulate the expression of genes necessary for DC presenting antigen, induce the secretion of immunosuppressive factor IL-10, prevent cell surface molecules from binding to receptors, inhibit the low-grade inflammation and prevent the consumption of immune cells (134). Furthermore, EGCG could upregulate the G-CSF secreted by mononuclear MDSCs, a cytokine stimulating the directed proliferation and differentiation of hematopoietic stem cells (133). It also significantly inhibited the accumulation of MDSCs, leading to restoration of the IFN-γ level inhibited by MDSCs, enhancement the activity of CD8+ T-cells, and improvement of the ratio of CD4(+) to CD8(+) T-cells (136), which is beneficial to the improvement of the immune system’s attack on tumor cells. In addition, a phytochemical mixture including EGCG could exert anti-tumor activity by repolarization of m2-polarized macrophages and induce the production of IL12, which could recruit CTLs and natural killer cells (NK) inside tumor cells (137). The combination of EGCG and vitamin A/E increased the adaptive B-cell activity by enhancing adaptive antibody responses and improving CD4(+) and CD8(+) T-cell responses, in which TNF, Il-6, and IL-17 were decreased but IL-15 increased (138). There were studies revealing that EGCG could induce lymphocyte migration in the presence of macrophages, suggesting that the anticancer effect of EGCG depended on the existence of the immune system to a certain extent (139).
It is shown that catechins could act on most of the pathways related to immune regulation (140), among which the star molecules NF-κB and STATS were the targeting signaling pathway of EGCG. EGCG contributes to immunosuppression by inhibiting NF-κB and STATS phosphorylation activation and blocking nuclear translocation to inhibit downstream gene expression (129, 136, 141). EGCG targeting Arg-1/iNOS/NOX2/NF-κB/STAT3 signaling pathway has also been demonstrated in detail, and its activation can enhance immune function (136). Molecular docking experiments proved that catechin has high affinity hydrogen binding ability with NF-κB p52 and TNF-α active site, but has no H-binding effect with anti-inflammatory factor IL 10 (142). Interestingly, EGCG may inhibit the ERK/JUK pathway rather than the p38 MAPK pathway by inhibiting the AP-1 in stimulated human T-cells. EGCG selectively activates signaling pathways (143).
In addition, EGCG also regulated many immune cytokines. For example, TNF-α, an important pro-inflammatory factor in the body, which activates endogenous inflammatory cascades. EGCG antagonized inflammation induced by TNF-α by suppressing the expression of pro-inflammatory cytokines IL-12, IL-6, IL-1β, IL-1α, inflammatory enzymes COX-2 and iNOS, and stimulating anti-inflammatory cytokines IL-10 and IL-4 expression (144). It has also been proved that tea polyphenols could reduce the destruction of the keratinocyte tight junction barrier induced by TNF-α (145). Tea catechins could significantly reduce the expression of TNF-α (142, 143, 146). Chemokines are also a kind of cytokines related to immune cell metastasis. After receiving the antigen information, the immune cells with a specific response will make directional movement along the concentration gradient of the chemical stimulus, which is the chemotaxis of immune cells, and the chemical stimulus that plays locating role is called chemokine. EGCG reduced the migration of neutrophils induced by the chemokine IL-8, inhibited neutrophils recruitment at inflammatory sites, and reduced the expression of pro-inflammatory factors such as MCP-1 (chemokine monocyte chemotactic protein 1) and its receptor (CCR2) (147). Other catechin components have been shown to have immunosuppressive activity. EC could inhibit cell proliferation by inhibiting the AMPK pathway, reducing Akt phosphorylation and mechanistic target of rapamycin (mTOR) expression (148). Like EGCG, EC reduced NF-κB p65 phosphorylation and blocked nuclear translocation, as well as inhibitor levels. By lowering the phosphorylation levels of MAPK members such as P38, JNK, and ERK1/2, EC blocked the signaling pathway (149). EC could inhibit the upregulation of TLR4 and activation of NOX to inhibit downstream events and achieve a preventive effect (150). Immunoactivity of ECG has also been reported, suggesting that ECG inhibited lymphocyte proliferation in an apoptosis-specific manner (151).
In general, catechins maintain the balance of the immune system and have a significant antagonistic effect against most abnormal responses caused by tumors. Catechins have bilateral regulatory effects, i.e., inhibiting the overreaction of the immune system and restoring the immune tolerance caused by tumor cells, in which secretion of cytokines is regulated by their targeting key signaling pathways to maintain a stable and orderly operation of the intracellular immune system.
Interaction with anticancer drugs
The advent of a variety of anticancer drugs can be beneficial to controlling cancers to a certain extent, resulting in an improvement in life quality for the patients. However, problems in clinical chemotherapy still exist, such as low bioavailability, cell resistance, and strong toxic and side effects, which greatly limit the use of the drugs. Studies were showing that catechins would play a role in alleviating these side effects induced by chemotherapy, owing to their diversely biological activities including antagonistic effects on oxidative stress. Due to their effects on the important signaling pathways in vivo, catechins are often used as sensitizing agents in combination with drugs. The multiple phenolic hydroxyl groups in catechins can bind with various proteins through hydrogen and ionic bonds, regulating the functions of the proteins. The combination of anti-cancer drugs with catechins, whether before or after drug administration, would alleviate the toxic and side effects induced by the drugs to a certain extent, resulting in apoptosis acceleration of cancer cells and efficacy enhancement of the drugs.
Interaction with cisplatin
Cisplatin is a platiniferous anticancer drug, which induces apoptosis of cancer cells by inhibiting their mitosis via binding directly to DNA and interfering with DNA replication and repair processes. But this is accompanied by extensive drug toxicity.
The combination of cisplatin with EGCG will improve the chemotherapeutic sensitivity of cells to the drug, leading to a reduction of cisplatin dosage. Copper transporter 1 (CTR1) is a transporter related to the intake of cisplatin. EGCG improved the sensitivity of cancer cells to cisplatin by stimulating the expression of CTR1. Specifically, EGCG inhibited ERK1/2, accompanied by enhancement of expression Nuclear enriched abundant transcript 1 (NEAT1) and CTR1 through ROS initiation (152). NEAT1 is a key factor inducing CSCs, and it causes cellular resistance to cisplatin. CTR1 and NETA1 are mutually regulated. EGCG-induced upregulation of expression in NEAT1 would increase CTR1 expression (153), which in turn inhibited CSCs regulated by NEAT1, leading to the reverse stemness induced by NEAT1 (154). Also, EGCG inhibited the CSCs phenotypes and reduced the resistance of cancer cells to drugs via increasing the expression of miRNA 485, an upstream gene for CD44 in cisplatin-resistant cells (155).
(−)-Epigallocatechin gallate is an inhibitor of some enzymes, including 5′-3′ structure-specific endonuclease excision repair cross-complementation group 1/xeroderma pigmentosum group F (ERCC1/XPF). ERCC1/XPF is an enzyme involved in repairing DNA damage induced by cisplatin. EGCG enhanced the sensitivity of cancer cells to cisplatin via targeting ERCC1/XPF, leading to the inhibition of DNA repair of the cancer cells (156).
(−)-Epigallocatechin gallate promoted cisplatin-induced cancer cell apoptosis and enhanced the therapeutic effect of cisplatin. Specifically, EGCG played the synergistic action through upregulation of the p19Arf-p53-p21Cip1 signaling pathway that plays a crucial role in regulating cell proliferation. Murine double minute 2 (MDM2) is a tumor suppressor which promotes ubiquitination and degradation of p53 whose expression is activated via inhibition of MDM2.
P19 inhibits MDM2 activity by binding to it. Compared with cisplatin alone, the combination of cisplatin with EGCG increased the expression of P19, P53, and P21 at both mRNA and protein levels, leading to cell cycle arrest in G1 phase and promoting apoptosis of cancer cells (157). Furthermore, EGCG-induced inactivation of NF-κB could prevent migration and self-renewal of cancer cells by coordinating the cell localization of NF-κB p65 and the transcriptional level of TWIST 1 (158).
(−)-Epigallocatechin gallate, with its excellent anti-inflammatory and antioxidant activity, can effectively ameliorate the toxic and side effects of cisplatin. The oral administration of EGCG could not only resist cisplatin-induced inflammation, reduce the production of apoptotic proteins and inhibit cell damage, but also improve the toxic effects of cisplatin-induced oxidative and nitrosative stress by enhancing the total antioxidant capacity (159, 160). EGCG downregulated NF-κB and upregulated nuclear factor-erythroid related factor 2 (Nrf2) and heme oxygenase 1 (HO-1), resulting in enhancement of anti-inflammatory effect. Meanwhile, EGCG itself could increase the activity of Caspase3/9, downregulate MDR1, regulate the AKT/STAT signaling pathway, and induce apoptosis and autophagy, which increases the cisplatin drug potency (80). When cisplatin was used with EGCG, acidic vesicular organelles and intracellular microtubule-associated protein 1 light chain 3 (LC3-II) in the cytoplasm were accumulated, accompanying an increase in autophagosomes, indicating that EGCG synergically inhibited cell proliferation and induced cell apoptosis by increasing autophagy (161).
Interaction with doxorubicin
Doxorubicin (DOX) is a widely used antibiotic drug that can kill multiple tumors, but it has serious toxic effects on healthy cells, such as DOX-induced ROS oxidative stress. EGCG scavenged ROS by improving the activity of antioxidant enzymes such as MnSOD, suppressing the depletion of the antioxidant glutathione and inhibiting MDA level, resulting in increased vitality of the healthy cells exposed to DOX (162). Administration of EGCG after DOX treatment could enhance the expression of EGFR family ErbB2 to promote cell growth, but reduce the activity of caspase 12 and calpain 2, downregulating the expression of NF-κB p65 subunit and the downstream genes in the apoptosis pathway, during which the anticancer efficacy of DOX was increased and its IC50 on cancer cells decreased (162). EGCG administration prior to DOX could effectively reduce pro-inflammatory factors TNF-α, NF-Kappa B, and iNOS induced by DOX (163).
In addition, EGCG can also reduce cancer cell resistance to DOX. Studies have shown that EGCG significantly suppressed the p-AKT and ERK by downregulating the expression of downstream STAT, making the cells more sensitive to the growth inhibition and apoptosis induced by DOX (164). Meanwhile, EGCG could inhibit DOX-induced overexpression of P-gp (165), an ABC transporter acting as an energy-dependent “drug pump” that excludes the drugs from the cells, reducing intracellular drug concentrations and resulting in drug resistance. EGCG could maintain the drug level in cancer cells by binding to P-gp (166) and promote apoptosis by reducing DOX-induced pro-survival autophagy (167). Further studies showed that EGCG interacted with DOX by reducing LncRNA SOX2OT variant 7, inactivating the Notch3/DLL3 signaling pathway targeting this RNA, leading to a reduction in the stemness of cancer cells and inhibition of their drug resistance (168).
Interaction with erlotinib
Erlotinib is an EGFR tyrosine kinase inhibitor that inhibits EGFR phosphorylation. Studies have shown that EGFR kinase domain mutations are happened in cancer cells, which induces ligand-independent phosphorylation and activates the downstream uncontrolled signal transmission. Both Erlotinib and EGCG could bind to the ATP binding pocket of EGFR, resulting in inhibition of the phosphorylation (169) and a synergistic effect with Erlotinib. Erlotinib stabilizes EGF on the plasmalemma, however, EGCG induced internalization and ubiquitination of EGF, resulting in disruption of EGFR signal transduction (170).
(−)-Epigallocatechin gallate synergistically inhibited Erlotinib-induced inhibition of cell cycle suppressors p21 and p27, leading to enhancement of the cell cycle arrest, during which the expression of apoptosis regulatory protein Bim was not affected. Protein p53 is a factor influencing the EGCG and Erlotinib induced growth inhibition by mediating the NF-κB signaling pathway and its downstream transcription target B-cell lymphoma-2 (Bcl-2). A combination of EGCG and Erlotinib could improve the expression of p53 (171). Further study showed that EGCG and its combination with Erlotinib had no significant effects on the mRNA expression of Bcl-2, p27, p21, or Bim, suggesting they are post-transcriptional regulation (172). The two drugs’ combination facilitated normal cell proliferation and suppressed tumor growth rate (173). Clinical trials on phase IB patients showed that the combination of GTPs and Erlotinib showed a high rate of pathological reaction and good cancer-free survival (CFS), suggesting that the adjuvant therapy of EGCG has a certain clinical promotion value (174).
Interaction with docetaxel
Docetaxel (DOC), a derivative of Taxanes, is a class of anticancer drugs targeting hormonal tumors. The hormonal tumors, such as prostate and BC, are largely caused by the uncontrolled binding of hormones to corresponding receptors. DOC produces aromatase inhibitors, leading to changes in transcriptional activity and nuclear localization of androgen receptors, and tumor inhibition. EGCG strengthened the inhibitive effect of DOC on the PISK/Akt signaling pathway, the transduction and activation of STAT3, and the expression of MDR protein, leading to inhibition of cancer cell invasion and metastasis (175). EGCG and DOC bound to different locations of tubulin, showing synergistically suppressive effects on the targeted tubulin, causing cell cycle arrest (176). Low-dose metronomic (LDM) chemotherapy showed that EGCG targeted the angiogenesis of normal cells and reduced the side effects of DOC (177). Furthermore, a combination of DOC in the EGCG significantly improved the absorption and transport of the anticancer drug DOC (178).
Interaction with irinotecan
Irinotecan (IRN) is a topoisomerase I inhibitor that inhibits tumor progression by targeting topoisomerase to damage DNA structure. The combination of IRN with EGCG could simultaneously improve the bioavailability of IRN and reduce its side effects. EGCG collaborated with IRN to cause more extensive DNA damage to arrest cancer cell cycle in the S/G2 phase and promote autophagy (179). Furthermore, EGCG enhanced the bioavailability of IRN by inhibiting the ATPase activity of drug pump P-gp, resulting in significant suppression of the bile efflux of IRN and SN-38, the active metabolite of IRN that prevented the drug efflux into the biliary elimination, leading to significantly prolong the drug’s half-life in plasma (180). Due to the antioxidant properties of catechins, the combination could effectively reduce IRN-induced toxicity, including ameliorating symptoms such as diarrhea and leukopenia, along with reduction of adenoma and non-alcoholic fatty liver disease (NAFLD) (181). EGCG could also reduce IRN-induced inflammatory factors and inhibit ROS production, and restore MMP-2 and MMP-9 reduction induced by IRN, protecting the oral mucosa against deleterious effects (182).
Interaction with daunorubicin
Daunorubicin (DNR) is a powerful anticancer drug, but it has serious cardiotoxicity. Carbonyl reductase 1 (CBR1) metabolizes DNR into Daunorubicinol, which reduces the anticancer activity of DNR and increases its cardiotoxicity. Catechins, especially EGCG, inhibited the catalytic activity of CBR1 by directly binding to its active site, reducing the side effects of DNR (183). The transport of DNR is P-gp dependent, and catechins would interact with the allosteric site of P-gp to improve its holistic function and transport efficiency, and ultimately inhibited DNR outflow, improving the intracellular DNR level (184). EGCG could bind to 67LR and activate PP2A through EGCG/67LR/PKA/PP2A pathway, leading to the dephosphorylation of subunit MYPT1 of myosin phosphatase, making THP-1 leukemic cells more sensitive to DNR and promoting apoptosis (185).
Interaction with gemcitabine
Gemcitabine is a cytosine nucleoside derivative, which is activated by deoxycytosine kinase and metabolized by cytosine nucleoside deaminase after entering the body. It is a drug that directly acts on genes through the incorporation of major metabolites into DNA and influences the G1/S phase. EGCG played a synergistic role with Gemcitabine through the inhibition of signaling pathways. EGCG decreased the phosphorylation levels of signal transduction factors such as AKT, ERK, JNK, etc., which weakened cell stress response to stimulation caused by treatment and improved cell sensitivity, leading to an increase in drug effects (186). STAT3 seemed to be a key acting site that enhanced the inhibition of cell viability by EGCG, while Gemcitabine could inhibit the target gene of STAT, showing a synergistic effect with EGCG (164, 187).
Epithelial-mesenchymal transition is a marker of cancer metastasis. The combination of Gemcitabine with EGCG inhibited the growth, invasion, and migration of pancreatic cancer cells partially via the suppression of EMT and Akt pathway, resulting in enhancement of Gemcitabine efficacy (114). Tumor suppressor factors such as DAPK2 and decoy receptor of RANK ligand OPG were upregulated by EGCG. The increased expression of OPG suppressed the activation of NF-κB induced by the RANK receptor, making cells more prone to apoptosis (188).
Interaction with 5-fluorouracil
The 5-fluorouracil (5-FU) is a thymidylate synthase inhibitor that inhibits the methylation of deoxyuridine acid (dUMP) into deoxythymidylate (dTMP), affecting DNA synthesis. Green tea extract or EGCG could improve the half-life of 5-FU (189), enhancing its growth inhibition, and reducing the viability and migration of cancer cells. Moreover, studies have shown that EGCG increased the number of cells in G2/M phase, while 5-FU blocked the cell cycle in the S phase (190). On the other hand, EGCG could enhance 5-FU resistance by inhibiting the expression of P-GP and receptor VEGF (191). Furthermore, EGCG targeted the ATP binding domain of glucose-regulated protein 78 (GRP78), preventing it from exercising its protective function and increasing the sensitivity of cells to drugs (192). Inhibition of GRP78 activates the NF-κB pathway, leading to a series of downstream responses, for example, which increase mir-155-5p targeted MDR1 expression which suppresses efflux of 5-FU, leading to the accumulation of 5-FU in cancer cells, activation of Caspase-3 and PARP, and decrease in Bcl-2 but increase in Bad, which in turn causes the cancer cells apoptosis (193).
Interaction with sulindac
Sulindac is a non-steroidal anti-inflammatory drug, which can inhibit inflammatory factors in vivo. EGCG could prevent lipid peroxidation, downregulate the expression of iNOS, and effectively reduce intracellular oxidative damage. When combined with Sulindac, EGCG improved the anti-inflammatory activity of Sulindac by increasing the activity of caspase-3, and so induced cell apoptosis, promoting therapeutic effects of Sulindac (194). The combination of Sulindac and green tea catechins upregulated RARα1, but downregulated MAP3KI4 (NF-κB induced kinase), death-related protein kinase I (DAPK1), and tyrosine protein kinase (SKY), inhibiting the NF-κB pathway and blocking important signaling in cancer development, though GADD153, a key transcription factor inducing apoptosis, and P21, a protein blocking cell cycle and inhibiting proliferation, were not significantly upregulated when the two drugs were used individually (195).
Aberrant crypt foci (ACF) is a new precancerous lesion that occurs in the early stage of CRC. The combination of EGCG and Sulindac could synergistically promote cancer cell apoptosis by inhibiting the formation of ACF and alleviating the side effects caused by Sulindac (196).
Interaction with gefitinib
Gefitinib is another tyrosine kinase inhibitor, and its combination with EGCG could enhance the inhibition of tumor migration and invasion by decreasing enzymatic activity of tyrosine kinase and the expression of MMP-2, and suppressing the phosphorylation of ERK, JNK, p38, and AKT. Most importantly, EGCG administration inhibited the phosphorylation of EGFR, making the cancer cells more sensitive to Gefitinib (197). An epigallocatechin gallate derivative (EGCGD) isolated from Anhua dark tea presented cancer cells from resistance to Gefitinib through suppressing EMT and PI3K/mTOR signal pathway (198).
Interaction with temozolomide
Temozolomide (TMZ) is a DNA-methylating drug. O-6-methylguanine DNA-methyltransferase (MGMT) is the key factor for cell resistance, thus maintaining a low level of MGMT expression is more conducive to the efficacy of TMZ. EGCG could inhibit the expression of MGMT. EGCG alleviated the resistance of MGMT to TMZ and then enhanced TMZ cytotoxicity to cancer cells by inhibiting β-catenin transfer into the nucleus and blocking the expression of downstream genes (199). EGCG degraded PARP by downregulating P-Akt, Bcl-2, and P-gp, suppressing the vitality of drug-resistant cancer cells and decreasing the stemness of tumor cells, resulting in more sensitivity of cancer cells to TMZ and apoptosis (200).
Glucose-regulated protein 78 plays an important role in protein folding and assembly. Cell surface GRP78 is found to act as a receptor or co-receptor for numerous ligands, promoting signaling cascades relating to tumor cell survival and proliferation. EGCG could directly act on the ATP binding domain of GRP78, decreasing the protective function of GRP78, and making cancer cells more sensitive to TMZ (192). The combination of TMZ with EGCG increased the therapeutic efficacy of TMZ in orthotopic mouse glioblastoma models by inhibiting the expression level of GRP78 (201).
Synergistic effects of EGCG were found in many other anticancer drugs. EGCG could significantly enhance the inhibitory effect of Safingol (a competitive inhibitor of SPHK1) on CLL cancer cells. EGCG/SPHK1 inhibitor combinations would be a novel therapeutic strategy for CLL patients with 67LR and SPHK1 overexpression (202). Tegafur, a chemotherapy drug for gastrointestinal tumors, reduces the expression of α-defensin, an important antibacterial peptide in the intestinal innate immune system, and induces the production of ROS. EGCG, when used with Tegafur, inhibited the production of ROS induced by Tegafur and prevented α-defensin expression decline caused by Tegafur, reducing the side effects of drugs (203).
Dacarbazine is an anti-tumor drug that induces cancer cell growth arrest or apoptosis by causing nucleic acid methylation or direct DNA damage. Combination of EGCG and Dacarbazine enhanced the efficacy of Dacarbazine by inhibiting activities of FAK and MMP-9, as well as proliferation and/or metastasis of cancer cells, in which the effective dosage of Dacarbazine was reduced, resulting in less potential cytotoxicity to the healthy cells (204).
Much attention has been paid to the clinical adjuvant therapy of catechins (40). For EGCG, administered after Sunitinib, showed synergistic effects by acting on the IRS/MAPK pathway, in which the effects of Sunitinib on inhibition of proliferation and VEGF secretion were increased. In vivo experiments showed that EGCG injection at the 4th hour after Sunitinib administration reduced angiogenesis and inhibited tumor growth, accompanying significant downregulation of IRS-1 levels.
Regulation of signaling pathways
The signaling pathway is the core system in which cells regulate various physiological processes and respond to external stimuli. Normally, cells have a complete set of regulatory mechanisms for initiating and/or inhibiting signal reception, cascade transmission, and ultimately gene expression, but in cancer cells, the signaling pathway is usually overactivated, and the balance is broken. Catechins played an anticarcinogenic role by promoting and/or inhibiting signal transmission through the targeted regulation of multiple links in the signal pathways.
(−)-Epigallocatechin gallate regulates signaling pathways by interacting with membrane receptors. Signal transduction usually begins when an external stimulus activates a receptor on the cell membrane. Membrane target protein 67LR, an important membrane glycoprotein to communicate with the ECM, is widely expressed in tumor cells, which in turn can affect tumor metastasis and regulate many other signal pathways. EGCG matched to 67LR at residues 161–170 (205, 206) and bound to 67LR via lipid rafts (207), blocking the activation of downstream pathways by preventing the combination of the receptors and ligands. In the PKA/PP2A pathway, EGCG binding to 67LR induced the activation of PKA, which dephosphorylates related proteins such as the tumor suppressor Merlin and inhibited the proliferation of cancer cells (208) (Figure 4A). VEGF receptor is a high affinity receptor binding specifically to VEGF. Inactivation of the VEGF signaling pathway suppresses angiogenesis, a common strategy for inhibiting carcinogenesis. EGCG significantly inhibited the expression of VEGF and reduced VEGF receptors (209).
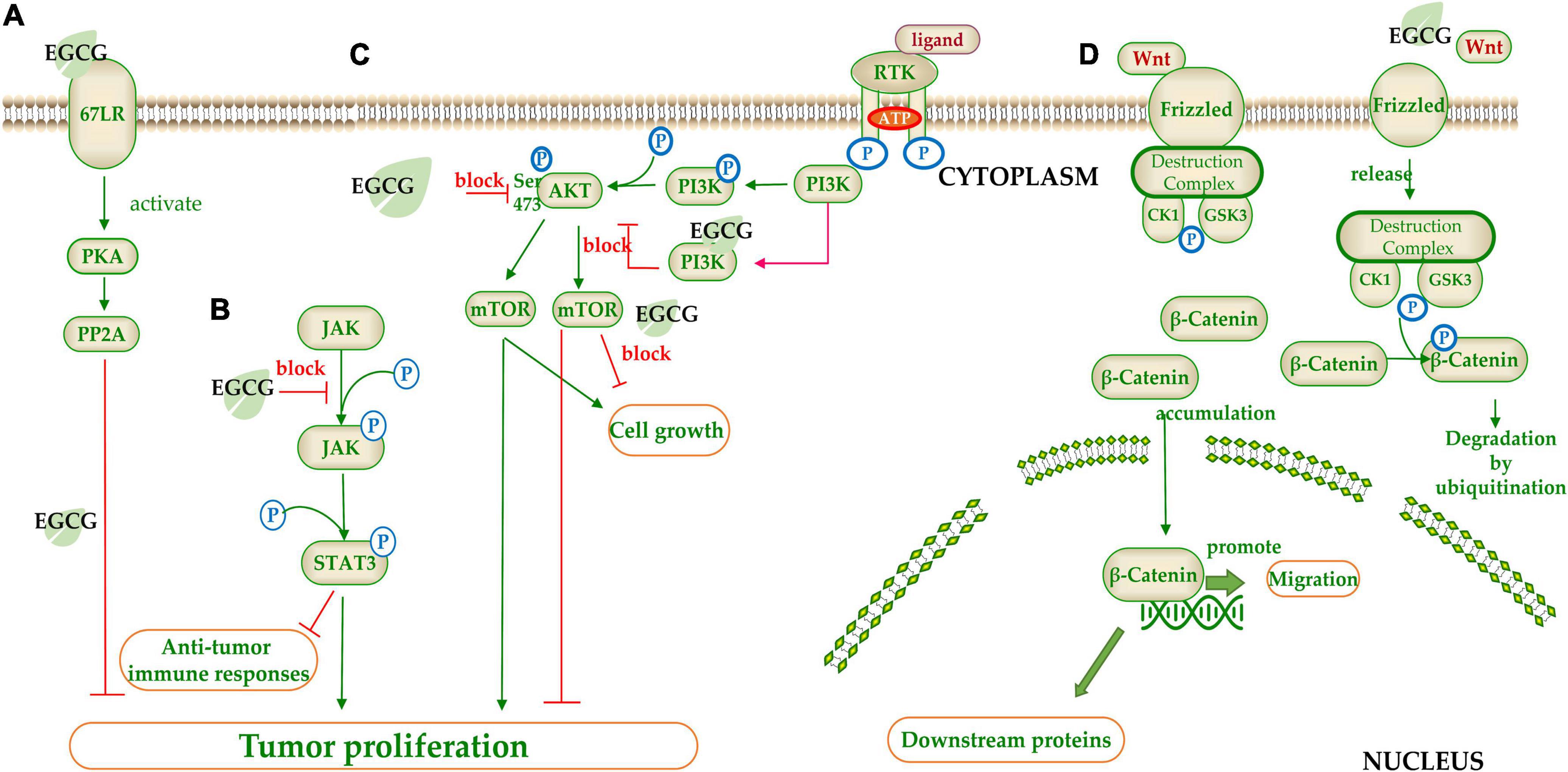
Figure 4. The target of catechins in the signaling pathway. (A) 67LR/PKA/PP2A signal pathway; (B) JAK/STAT signal pathway; (C) mTOR/AKT signal pathway; (D) Wnt/β-Catenin signal pathway. The green arrow represents the signal transmission, red arrow represents inhibition.
(−)-Epigallocatechin gallate regulates signaling pathways by inhibiting phosphorylation of signaling molecules (Figures 4B–D). STAT is a transcription factor family that combines with DNA to initiate downstream genes transcript. STAT3 suppresses anti-tumor immune responses and promotes the proliferation and migration of cancer cells. EGCG inhibited STAT3 phosphorylation by blocking JAK2 phosphorylation (210) (Figure 4B). The mTOR is a serine/threonine kinase belonging to the PI3K related kinase (PIKK) family, which can regulate cell growth and proliferation (211). EGCG blocked AKT phosphorylation at Ser473, and also acted as an ATP competition inhibitor competing for ATP binding sites of PI3K and mTOR to block the mTOR signal pathway (212) (Figure 4C). MAPK signaling pathway is a core pathway in the cellular regulatory network. EGCG inhibited the MAPK signaling by competing for the phosphorylation sites of downstream proteins (213). Wnt (Wingless-type mice mammary tumor virus integration site family) pathway is a highly conserved signal pathway in species evolution, which plays an important role in early embryo development, organogenesis, and tissue regeneration. The key factor in this pathway is β-catenin, a class of transcription factors that activate the cell division related gene expression. β-catenin binds to the destruction complex when the Wnt ligand does not bind to Frizzled family of proteins (FZD). CK1 and GSK3 in destruction complex can phosphorylate β-catenin, leading to its degradation by E3 ubiquitin ligase. When Wnt is combined with FZD, destruction complex will be confined to the cell membrane and cannot degrade β-catenin, resulting in accumulation of β-catenin in the cytoplasm (214). EGCG could inhibit the Wnt pathway by phosphorylating β-catenin and promoting its degradation (215) (Figure 4D).
(−)-Epigallocatechin gallate suppressed some signaling pathways by reducing the related bioactive proteins (216). Sonic hedgehog (SHH) pathway involves in regulating cell proliferation and differentiation. Excessive activation of SHH leads to carcinogenesis. The downstream transcription factor Gli1 is the key factor of the SHH pathway and it is regulated by membrane receptors Patched (Ptc) and Smoothened (Smo). Normally, Ptc inhibits Smo, and Gli1 binds to the protein kinase inhibitor SUFU and is trapped in the cytoplasm. When SHH binds to Ptc1, the inhibition of Smo is relieved and SUFU is disconnected from Gli1. Then Gli1 will enter the nucleus to initiate the expression of downstream genes (217). EGCG inhibited the expression of Smo, SHH, Gli1, and Gli2 (193) (Figure 5A).
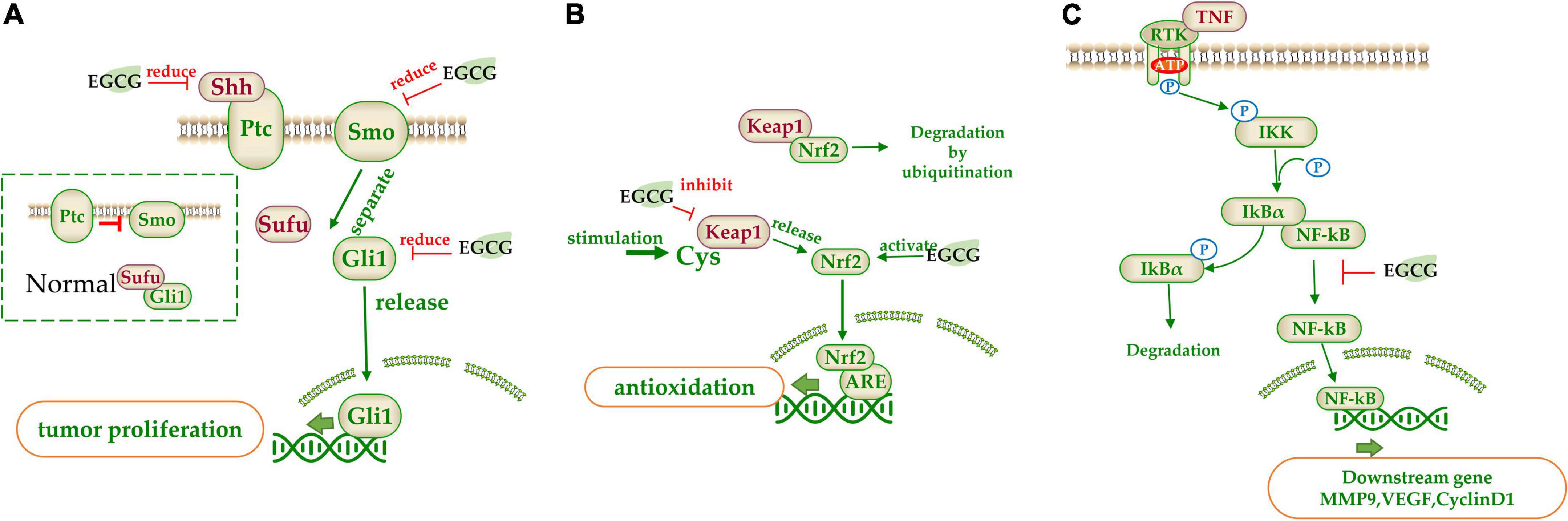
Figure 5. The target of catechins in the signaling pathway. (A) Sonic hedgehog (SHH) pathway signal pathway; (B) Nrf2 signal pathway; (C) NF-κB signal pathway. The green arrow represents the signal transmission, red arrow represents inhibition.
(−)-Epigallocatechin gallate regulates some signaling pathways by interacting with specific inhibitors in the pathway. Nrf2 pathway involves in a variety of diseases and Kelch-like ECH-associated protein 1 (Keap1) is its inhibitor which acts as a bridge between Nrf2 and E3 ubiquitin ligases. When Nrf2 is inactive, it will be degraded by ubiquitination. When Keap1’s key cysteine is modified upon stimulation, it will lose its inhibitive effect and release the Nrf2. The released Nrf2 is then transferred to the nucleus to bind with antioxidant responsive element (ARE), which activates downstream transcription factors. EGCG could inhibit Keap1 and activate Nrf2, resulting in the expression of the downstream antioxidant related genes (218) (Figure 5B). Furthermore, EGCG could induce the re-expression of secreted frizzled-related protein 1 (SFRP1), an inhibitor of the Wnt pathway, which prevents ligand-receptor interaction and is silenced in cancer cells (215).
(−)-Epigallocatechin gallate can regulate some signaling pathways by controlling downstream genes expression in the signal pathway. EGCG could act on the MMP associated with cell apoptosis (207, 219). These enzymes are secreted by tumor cells and can degrade various proteins in the ECM and are the main proteolytic enzymes in tumor invasion and metastasis. EGCG inhibited the activities of MMP2 and MMP9, and promoted the expression of tissue inhibitor of MMPs (TIMp1/2) to suppress the invasion and metastasis of tumor cells (107). Peptidyl-prolyl isomerase NIMA-interacting 1 (PIN1) is a specially phosphorylated prolyl isomerase necessary for mitotic regulation and a direct target of EGCG. EGCG inhibited the activity of PPIase (207) and reduced the expression of PIN, inducing apoptosis (220). NF-κB is normally inactivated when it binds to the inhibitor IkBα. However, when upstream signal factor TNF binds to membrane receptors, IkB kinase (IKK) is activated, which phosphorylates IkBα and dissociates NF-κB. Free NF-κB is released into the nucleus and binds with nuclear DNA to initiate the transcription of genes such as CyclinD1, C-MYC, MMP-9, and VEGF (221). EGCG could significantly downregulate NF-κB activity, inhibiting these gene transcription (222) (Figure 5C).
Inconsistent results and future expectations
Although there have been a lot of in vivo and in vitro studies revealing tea catechins had anticarcinogenic effects, inconsistent results were also observed and reported. A 7-year (1995–2001) population-based cohort study in Japan involving 41,440 male and female patients with ages ranging from 40 to 79 years gave no direct evidence showing green tea consumption being correlated to lower risk of lung cancer (223). No significant relationship was found between colon cancer and intake of EC or tea (30, 224). Population based case-control studies showed green tea drinking was no associated a reduced risk of pancreatic cancer (225). A screening study on prostate, lung, colorectal and ovarian (PLCO) cancers involving 57,398 men and women showed that heavy tea drinking was not correlated to risk of overall CRC (RR = 0.77, 95% CI = 0.55∼1.09, p = 0.17) or the risks of CRC cancer site (p = 0.14) or CRC stage (p = 0.60) (226). A cohort study in Japan showed no significant relationship of green tea drinking to the reduced risk of acute myeloid leukemia (227). A 14-year follow-up EPIC (European Prospective Investigation into Cancer and Nutrition) cohort study including 476,160 male and female residents from 10 European countries, among which there were 5,991 incident CRC cases (3,897 colon cancer cases and 2,094 rectum cancer cases), revealed by multivariable-adjusted Cox regression model, that a doubling intake of total dietary polyphenol was not correlated to CRC risk in men (HR = 0.97, 95% CI = 0.90∼1.05) or in women (HR = 1.06, 95% CI = 0.99∼1.14) (228).
What causes the inconsistency in these research findings? The metabolism and/or chemically changing of the in-taken catechins might be important factors influencing the anticarcinogenic effects of tea and catechins. There was a study showing that the in-taken catechins are promptly O-methylated by COMT (human catechol-O-methyltransferase) and so the association between tea intake and BC depends on COMT genotype. Among women who carried minimum one low activity COMT allele, the risk of BC in tea drinkers was significantly lower than those who did not drink tea (OR = 0.48, 95% CI = 0.29∼0.77), after balancing the related dietary, menstrual, reproductive, and demographic factors. Among women who carried homozygous high activity COMT allele, however, no significant difference in the BC risk was observed between non-tea drinkers and tea drinkers (OR = 1.02, 95% CI = 0.66∼1.60) (18, 20). The risk of lung cancer was decreased by 72% among daily tea drinkers who carried the OGG1 Cys (326) allele (95% CI = 0.09∼0.94). Among people carrying GSTM1 null homozygotes, on significant difference in lung cancer risk was observed between daily tea consumers and tea non-consumers. Green tea drinking showed no effect on the risk of lung cancer among GSTM1, AKR1C3, or OGG1 Ser (326) homozygote carriers. It is considered that the chemopreventive effects of green tea consumption may be limited to the population who are exceptionally susceptible to DNA damages induced by oxidative stress (71, 94, 156).
Interaction of catechins with partial drugs resulted in a reduction in drug bioavailability might lead decrease in the therapeutic efficacy of the drugs. Simultaneous administration of EGCG and anticancer drug Sunitinib would decrease Sunitinib concentration in plasma, thus reducing its therapeutic effect (224) because EGCG has the same binding sites on human serum albumin (HSA) as some drugs, such as DOX and TF (225, 226), leading to less bioavailability of the drugs.
To explore the potentials of tea catechins in anticarcinogenic drug development, the following topics should be focused on: (1) metabolism of in-taken catechins in the gastrointestinal tract and development of methods for protecting the in-taken catechins from degradation or transformation; (2) technology for improving the bioavailability of catechins, such as encapsulation and nanoparticle; (3) novel catechins formulae with synergic anticarcinogenic effects.
Conclusion
Epidemiological and in vivo studies showed that tea catechins have anticarcinogenic effects on many cancers including gynecological cancers, digestive tract cancers, incident glioma, liver and gallbladder cancers, lung cancer, etc. Catechins suppressed cancers through inhibiting proliferation and metastasis of cancer cells, antioxidation and scavenging free radicals, enhancing body immunity, and synergistically interacting with anticancer drugs, which involved many signaling pathways (Figure 6).
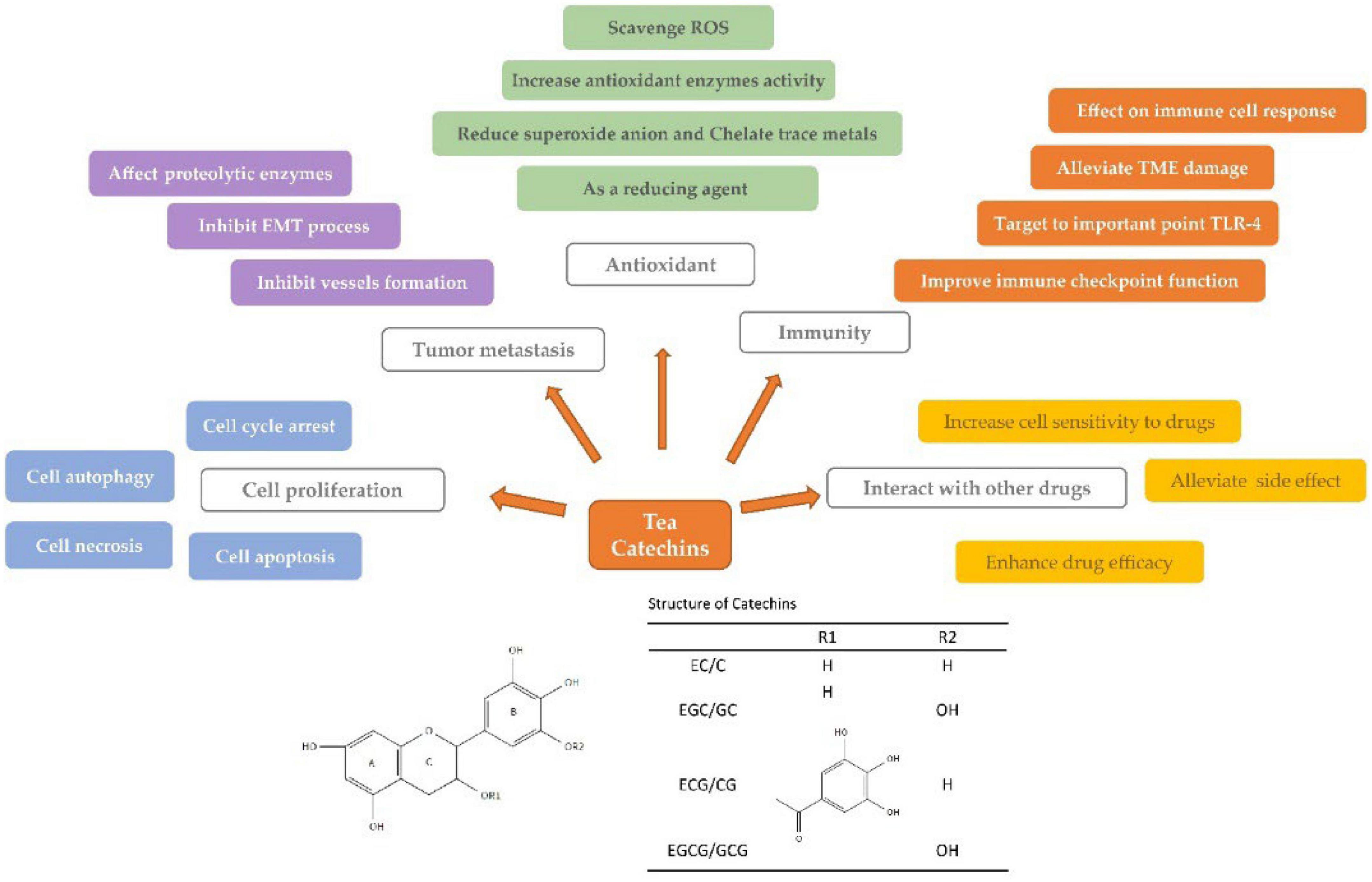
Figure 6. Anticarcinogenic mechanism of tea catechins. Tea catechins exert their anticarcinogenic effects by inhibiting cell proliferation (blue module), preventing tumor metastasis (purple module), reducing oxidative damage (green module), improving immune activity (orange module), and ameliorating the potency of anticancer drugs (yellow module). Different colored textboxes indicate the specific ways in which tea catechins exert.
Author contributions
Y-RL and X-QZ designed the project. Y-RL, X-QZ, and X-XL searched and collected the literature. X-XL wrote the section “Interaction with anticancer drugs” and designed the Figures. CL wrote the sections “Inhibition of cancer cell proliferation and growth” and “Antioxidant and free radical scavenging.” S-LD wrote the section “Inhibition of metastasis of cancer cells.” C-SO wrote the section “Regulation of immunity.” J-LL wrote the section “Regulation of signaling pathways.” J-HY wrote the section “Inconsistent results and future expectation.” Y-RL wrote the sections “Introduction,” “Gynecological cancers,” “Digestive tract cancers,” “Incident glioma,” and “Conclusion.” X-QZ wrote the sections “Liver and gallbladder cancers,” “Lung cancer,” and “Other cancers.”
Acknowledgments
We appreciate the Agriculture and Rural Affairs Department of Guangxi Zhuang Autonomous Region and China Agriculture Research System of MOF and MARA (project CARS-19) for their financial support for this study.
Conflict of interest
The authors declare that the research was conducted in the absence of any commercial or financial relationships that could be construed as a potential conflict of interest.
Publisher’s note
All claims expressed in this article are solely those of the authors and do not necessarily represent those of their affiliated organizations, or those of the publisher, the editors and the reviewers. Any product that may be evaluated in this article, or claim that may be made by its manufacturer, is not guaranteed or endorsed by the publisher.
References
1. Kocarnik J, Compton K, Dean F, Fu W, Gaw B, Harvey J, et al. Cancer incidence, mortality, years of life lost, years lived with disability, and disability-adjusted life years for 29 cancer groups from 2010 to 2019. JAMA Oncol. (2022) 8:420.
2. Bray F, Jemal A, Grey N, Ferlay J, Forman D. Global cancer transitions according to the human development index (2008–2030): a population-based study. Lancet Oncol. (2012) 13:790–801. doi: 10.1016/S1470-2045(12)70211-5
3. Foreman K, Marquez N, Dolgert A, Fukutaki K, Fullman N, Mcgaughey M, et al. Forecasting life expectancy, years of life lost, and all-cause and cause-specific mortality for 250 causes of death: reference and alternative scenarios for 2016-40 for 195 countries and territories. Lancet. (2018) 392:2052–90.
4. Wild C, Weiderpass E, Stewart B. World Cancer Report: Cancer Research for Cancer Prevention. Lyon: International Agency for Research on Cancer (2020).
5. Sung H, Ferlay J, Siegel R, Laversanne M, Soerjomataram I, Jemal A, et al. Global cancer statistics 2020: GLOBOCAN estimates of incidence and mortality worldwide for 36 cancers in 185 countries. CA Cancer J Clin. (2021) 71:209–49. doi: 10.3322/caac.21660
6. Xiang L, Wang A, Ye J, Zheng X, Polito C, Lu J, et al. Suppressive effects of tea catechins on breast cancer. Nutrients. (2016) 8:458. doi: 10.3390/nu8080458
7. Mandair D, Rossi R, Pericleous M, Whyand T, Caplin M. The impact of diet and nutrition in the prevention and progression of hepatocellular carcinoma. Expert Rev Gastroenterol Hepatol. (2014) 8:369–82. doi: 10.1586/17474124.2014.894879
8. Zheng X, Nie Y, Gao Y, Huang B, Ye J, Lu J, et al. Screening the cultivar and processing factors based on the flavonoid profiles of dry teas using principal component analysis. J Food Compos Anal. (2018) 67:29–37. doi: 10.1016/j.jfca.2017.12.016
9. Kim E, Liang Y, Jin J, Sun Q, Lu J, Du Y, et al. Impact of heating on chemical compositions of green tea liquor. Food Chem. (2007) 103:1263–7. doi: 10.1016/j.foodchem.2006.10.031
10. Liang H, Liang Y, Dong J, Lu J. Tea extraction methods in relation to control of epimerization of tea catechins. J Sci Food Agric. (2007) 87:1748–52. doi: 10.1002/jsfa.2913
11. Chen X, Lu W, Zheng Y, Gu K, Chen Z, Zheng W, et al. Exercise, tea consumption, and depression among breast cancer survivors. J Clin Oncol. (2010) 28:991–8. doi: 10.1200/JCO.2009.23.0565
12. Li M, Tse L, Chan W, Kwok C, Leung S, Wu C, et al. Evaluation of breast cancer risk associated with tea consumption by menopausal and estrogen receptor status among Chinese women in Hong Kong. Cancer Epidemiol. (2016) 40:73–8. doi: 10.1016/j.canep.2015.11.013
13. Chacko S, Thambi P, Kuttan R, Nishigaki I. Beneficial effects of green tea: a literature review. Chin Med. (2010) 5:13. doi: 10.1186/1749-8546-5-13
14. Suzuki Y, Tsubono Y, Nakaya N, Suzuki Y, Koizumi Y, Tsuji I. Green tea and the risk of breast cancer: pooled analysis of two prospective studies in Japan. Br J Cancer. (2004) 90:1361–3. doi: 10.1038/sj.bjc.6601652
15. Zhang M, Holman C, Huang J, Xie X. Green tea and the prevention of breast cancer: a case-control study in Southeast China. Carcinogenesis. (2006) 28:1074–8. doi: 10.1093/carcin/bgl252
16. Imai K, Suga K, Nakachi K. Cancer-preventive effects of drinking green tea among a Japanese population. Prev Med. (1997) 26:769–75. doi: 10.1006/pmed.1997.0242
17. Lei L, Yang Y, He H, Chen E, Du L, Dong J, et al. Flavan-3-ols consumption and cancer risk: a meta-analysis of epidemiologic studies. Oncotarget. (2016) 7:73573–92. doi: 10.18632/oncotarget.12017
18. Wu A, Yu M, Tseng C, Hankin J, Pike M. Green tea and risk of breast cancer in Asian Americans. Int J Cancer. (2003) 106:574–9. doi: 10.1002/ijc.11259
19. Nakachi K, Suemasu K, Suga K, Takeo T, Imai K, Higashi Y. Influence of drinking green tea on breast cancer malignancy among Japanese patients. Jpn J Cancer Res. (1998) 89:254–61. doi: 10.1111/j.1349-7006.1998.tb00556.x
20. Wu A, Tseng C, Van Den Berg D, Yu M. Tea intake, COMT genotype, and breast cancer in Asian-American women. Cancer Res. (2003) 63:7526–9.
21. Sun C, Yuan J, Koh W, Yu M. Green tea, black tea and breast cancer risk: a meta-analysis of epidemiological studies. Carcinogenesis. (2006) 27:1310–5. doi: 10.1093/carcin/bgi276
22. Wu A, Ursin G, Koh W, Wang R, Yuan J, Khoo K, et al. Green tea, soy, and mammographic density in Singapore Chinese women. Cancer Epidemiol Biomarkers Prev. (2008) 17:3358–65. doi: 10.1158/1055-9965.EPI-08-0132
23. Wang S, Li X, Yang Y, Xie J, Liu M, Zhang Y, et al. Does coffee, tea and caffeine consumption reduce the risk of incident breast cancer? A systematic review and network meta-analysis. Public Health Nutr. (2021) 24:6377–89. doi: 10.1017/S1368980021000720
24. Baker J, Boakye K, Mccann S, Beehler G, Rodabaugh K, Villella J, et al. Consumption of black tea or coffee and risk of ovarian cancer. Int J Gynecol Cancer. (2007) 17:50–4. doi: 10.1111/j.1525-1438.2006.00773.x
25. Gao M, Ma W, Chen X, Chang Z, Zhang X, Zhang M. Meta-analysis of green tea drinking and the prevalence of gynecological tumors in women. Asia Pac J Public Health. (2013) 25:43S–8S. doi: 10.1177/1010539513493313
26. Gao Y, Cao Z, Zhou F, Zhao Y, Tang L, Zhang H. Association between tea drinking and endometrial cancer risk: a meta-analysis. Food Sci Technol. (2022) 42:e90021. doi: 10.1590/fst.90021
27. Zheng W, Doyle T, Kushi L, Sellers T, Hong C, Folsom A. Tea consumption and cancer incidence in a prospective cohort study of postmenopausal women. Am J Epidemiol. (1996) 144:175–82. doi: 10.1093/oxfordjournals.aje.a008905
28. Ji B, Chow W, Hsing A, Mclaughlin J, Dai Q, Gao Y, et al. Green tea consumption and the risk of pancreatic and colorectal cancers. Int J Cancer. (1997) 70:255–8. doi: 10.1002/(SICI)1097-0215(19970127)70:3<255::AID-IJC1>3.0.CO;2-W
29. Mu L, Zhou X, Ding B, Wang R, Zhang Z, Chen C, et al. A case-control study on drinking green tea and decreasing risk of cancers in the alimentary canal among cigarette smokers and alcohol drinkers. Zhonghua Liu Xing Bing Xue Za Zhi. (2003) 24:192–5.
30. Yuan J, Gao Y, Yang C, Yu M. Urinary biomarkers of tea polyphenols and risk of colorectal cancer in the Shanghai cohort study. Int J Cancer. (2007) 120:1344–50. doi: 10.1002/ijc.22460
31. Suzuki E, Yorifuji T, Takao S, Komatsu H, Sugiyama M, Ohta T, et al. Green tea consumption and mortality among Japanese elderly people: the prospective Shizuoka elderly cohort. Ann Epidemiol. (2009) 19:732–9. doi: 10.1016/j.annepidem.2009.06.003
32. Inoue M, Sasazuki S, Wakai K, Suzuki T, Matsuo K, Shimazu T, et al. Green tea consumption and gastric cancer in Japanese: a pooled analysis of six cohort studies. Gut. (2009) 58:1323–32. doi: 10.1136/gut.2008.166710
33. Kang H, Rha S, Oh K, Nam C. Green tea consumption and stomach cancer risk: a meta-analysis. Epidemiol Health. (2010) 32:e2010001. doi: 10.4178/epih/e2010001
34. Nechuta S, Shu X, Li H, Yang G, Ji B, Xiang Y, et al. Prospective cohort study of tea consumption and risk of digestive system cancers: results from the Shanghai women’s health study. Am J Clin Nutr. (2012) 96:1056–63. doi: 10.3945/ajcn.111.031419
35. Takada M, Yamagishi K, Iso H, Tamakoshi A. Green tea consumption and risk of hematologic neoplasms: the Japan collaborative cohort study for evaluation of cancer risk (JACC Study). Cancer Cause Control. (2019) 30:1223–30. doi: 10.1007/s10552-019-01220-z
36. Chen H, Sun Z, Li C, Chou Y, Chang C, Lu F, et al. Cumulative tea consumption is inversely associated with colorectal adenomas in adults: a cross-sectional study in a Taiwanese population. Cancer Epidemiol. (2021) 73:101945. doi: 10.1016/j.canep.2021.101945
37. Creed J, Smith-Warner S, Gerke T, Egan K. A prospective study of coffee and tea consumption and the risk of glioma in the UK biobank. Eur J Cancer. (2020) 129:123–31. doi: 10.1016/j.ejca.2020.01.012
38. Bever A, Cassidy A, Rimm E, Stampfer M, Cote D. A prospective study of dietary flavonoid intake and risk of glioma in US men and women. Am J Clin Nutr. (2021) 114:1314–27. doi: 10.1093/ajcn/nqab178
39. Pranata R, Feraldho A, Lim M, Henrina J, Vania R, Golden N, et al. Coffee and tea consumption and the risk of glioma: a systematic review and dose-response meta-analysis. Brit J Nutr. (2022) 127:78–86. doi: 10.1017/S0007114521000830
40. Zhang X, Andreotti G, Gao Y, Deng J, Liu E, Rashid A, et al. Tea drinking and the risk of biliary tract cancers and biliary stones: a population-based case–control study in Shanghai, China. Int J Cancer. (2006) 118:3089–94. doi: 10.1002/ijc.21748
41. Ui A, Kuriyama S, Kakizaki M, Sone T, Nakaya N, Ohmori-Matsuda K, et al. Green tea consumption and the risk of liver cancer in Japan: the Ohsaki cohort study. Cancer Cause Control. (2009) 20:1939–45. doi: 10.1007/s10552-009-9388-x
42. Fon Sing M, Yang W, Gao S, Gao J, Xiang Y. Epidemiological studies of the association between tea drinking and primary liver cancer. Eur J Cancer Prev. (2011) 20:157–65. doi: 10.1097/CEJ.0b013e3283447497
43. Li Y, Chang S, Goldstein B, Scheider W, Cai L, You N, et al. Green tea consumption, inflammation and the risk of primary hepatocellular carcinoma in a Chinese population. Cancer Epidemiol. (2011) 35:362–8. doi: 10.1016/j.canep.2011.01.005
44. Mendilaharsu M, De Stefani E, Deneo-Pellegrini H, Carzoglio J, Ronco A. Consumption of tea and coffee and the risk of lung cancer in cigarette-smoking men: a case-control study in Uruguay. Lung cancer. (1998) 19:101–7. doi: 10.1016/S0169-5002(97)00075-5
45. Zhong L, Goldberg M, Gao Y, Hanley J, Parent M, Jin F. A population-based case-control study of lung cancer and green tea consumption among women living in Shanghai, China. Epidemiology. (2001) 12:695–700. doi: 10.1097/00001648-200111000-00019
46. Kubík A, Zatloukal P, Tomásek L, Pauk N, Petruzelka L, Plesko I. Lung cancer risk among nonsmoking women in relation to diet and physical activity. Neoplasma. (2004) 51:136.
47. Kubík A, Zatloukal P, Tomásek L, Pauk N, Havel L, Krepela E, et al. Dietary habits and lung cancer risk among non-smoking women. Eur J Cancer Prev. (2004) 13:471–80. doi: 10.1097/00008469-200412000-00002
48. Hakim I, Harris R. Joint effects of citrus peel use and black tea intake on the risk of squamous cell carcinoma of the skin. BMC Dermatol. (2001) 1:3. doi: 10.1186/1471-5945-1-3
49. Jian L, Xie L, Lee A, Binns C. Protective effect of green tea against prostate cancer: a case-control study in southeast China. Int J Cancer. (2004) 108:130–5. doi: 10.1002/ijc.11550
50. Bettuzzi S, Brausi M, Rizzi F, Castagnetti G, Peracchia G, Corti A. Chemoprevention of human prostate cancer by oral administration of green tea catechins in volunteers with high-grade prostate intraepithelial neoplasia: a preliminary report from a one-year proof-of-principle study. Cancer Res. (2006) 66:1234–40. doi: 10.1158/0008-5472.CAN-05-1145
51. Frankenfeld C, Cerhan J, Cozen W, Davis S, Schenk M, Morton L, et al. Dietary flavonoid intake and non-hodgkin lymphoma risk. Am J Clin Nutr. (2008) 87:1439–45. doi: 10.1093/ajcn/87.5.1439
52. Zhang M, Zhao X, Zhang X, Holman C. Possible protective effect of green tea intake on risk of adult leukaemia. Br J Cancer. (2008) 98:168–70. doi: 10.1038/sj.bjc.6604140
53. Kuo Y, Yu C, Liu C, Wang S, Pan P, Wu M, et al. A population-based, case-control study of green tea consumption and leukemia risk in Southwestern Taiwan. Cancer Causes Control. (2009) 20:57–65. doi: 10.1007/s10552-008-9217-7
54. Schaefer S, Kaiser A, Behrendt I, Eichner G, Fasshauer M. Association of alcohol types, coffee and tea intake with mortality: prospective cohort study of UK biobank participants. Br J Nutr. (2022) Online ahead of print. doi: 10.1017/S000711452200040X
55. Cheng S, Ho C, Feng J, Bai J, Guo S, Li X. On the antitumor activity of fresh green tea leaf. Agric Biol Chem. (1988) 52:1879–80. doi: 10.1080/00021369.1988.10868935
56. Ahn W, Huh S, Bae S, Lee I, Lee J, Namkoong S, et al. A major constituent of green tea, EGCG, inhibits the growth of a human cervical cancer cell line, CaSki cells, through apoptosis, G(1) arrest, and regulation of gene expression. DNA Cell Biol. (2003) 22:217–24. doi: 10.1089/104454903321655846
57. Sun H, Yin M, Hao D, Shen Y. Anti-cancer activity of catechin against a549 lung carcinoma cells by induction of cyclin kinase inhibitor p21 and suppression of cyclin E1 and P-AKT. Appl Sci. (2020) 10:2065. doi: 10.3390/app10062065
58. Babich H, Krupka M, Nissim H, Zuckerbraun H. Differential in vitro cytotoxicity of (–)-epicatechin gallate (ECG) to cancer and normal cells from the human oral cavity. Toxicol In Vitro. (2005) 19:231–42. doi: 10.1016/j.tiv.2004.09.001
59. Singh B, Shankar S, Srivastava R. Green tea catechin, epigallocatechin-3-gallate (EGCG): mechanisms, perspectives and clinical applications. Biochem Pharmacol. (2011) 82:1807–21. doi: 10.1016/j.bcp.2011.07.093
60. Yang C, Wang C, Hung J, Huang H, Chia Y, Wang P, et al. Pyrogallol induces G2-M arrest in human lung cancer cells and inhibits tumor growth in an animal model. Lung Cancer. (2009) 66:162–8. doi: 10.1016/j.lungcan.2009.01.016
61. Deguchi H, Fujii T, Nakagawa S, Koga T, Shirouzu K. Analysis of cell growth inhibitory effects of catechin through MAPK in human breast cancer cell line T47D. Int J Oncol. (2002) 21:1301–5. doi: 10.3892/ijo.21.6.1301
62. Ma Y, Li C, Gao F, Xu Y, Jiang Z, Liu J, et al. Epigallocatechin gallate inhibits the growth of human lung cancer by directly targeting the EGFR signaling pathway. Oncol Rep. (2014) 31:1343–9. doi: 10.3892/or.2013.2933
63. Mayr C, Wagner A, Neureiter D, Pichler M, Jakab M, Illig R, et al. The green tea catechin epigallocatechin gallate induces cell cycle arrest and shows potential synergism with cisplatin in biliary tract cancer cells. BMC Complement Altern Med. (2015) 15:194. doi: 10.1186/s12906-015-0721-5
64. Hibasami H, Komiya T, Achiwa Y, Ohnishi K, Kojima T, Nakanishi K, et al. Induction of apoptosis in human stomach cancer cells by green tea catechins. Oncol Rep. (1998) 5:527. doi: 10.3892/or.5.2.527
65. Hayakawa S, Saeki K, Sazuka M, Suzuki Y, Shoji Y, Ohta T, et al. Apoptosis induction by epigallocatechin gallate involves its binding to Fas. Biochem Biophys Res Commun. (2001) 285:1102–6. doi: 10.1006/bbrc.2001.5293
66. Brusselmans K, De Schrijver E, Heyns W, Verhoeven G, Swinnen J. Epigallocatechin-3-gallate is a potent natural inhibitor of fatty acid synthase in intact cells and selectively induces apoptosis in prostate cancer cells. Int J Cancer. (2003) 106:856–62. doi: 10.1002/ijc.11317
67. Yoon J, Lee J, Kim B, Ahn J, Yang K. Catechin-7-O-xyloside induces apoptosis via endoplasmic reticulum stress and mitochondrial dysfunction in human non-small cell lung carcinoma H1299 cells. Oncol Rep. (2014) 31:314–20. doi: 10.3892/or.2013.2840
68. Gan L, Zhong L, Shan Z, Xiao C, Xu T, Song H, et al. Epigallocatechin-3-gallate induces apoptosis in acute promyelocytic leukemia cells via a SHP-1-p38α MAPK-Bax cascade. Oncol Lett. (2017) 14:6314–20. doi: 10.3892/ol.2017.6980
69. Jiang S, Huang C, Zheng G, Yi W, Wu B, Tang J, et al. EGCG inhibits proliferation and induces apoptosis through downregulation of SIRT1 in nasopharyngeal carcinoma cells. Front Nutr. (2022) 9:851972. doi: 10.3389/fnut.2022.851972
70. Dong C, Wang Z, Shen P, Chen Y, Wang J, Wang H. Epigallocatechin-3-gallate suppresses the growth of human osteosarcoma by inhibiting the Wnt/β-catenin signalling pathway. Bioengineered. (2022) 13:8490–502. doi: 10.1080/21655979.2022.2051805
71. Farhan M, Rizvi A, Ahmad A, Aatif M, Alam M, Hadi S. Structure of some green tea catechins and the availability of intracellular copper influence their ability to cause selective oxidative DNA damage in malignant cells. Biomedicines. (2022) 10:664. doi: 10.3390/biomedicines10030664
72. Iwasaki R, Ito K, Ishida T, Hamanoue M, Adachi S, Watanabe T, et al. Catechin, green tea component, causes caspase-independent necrosis-like cell death in chronic myelogenous leukemia. Cancer Sci. (2009) 100:349–56. doi: 10.1111/j.1349-7006.2008.01046.x
73. Azmi N, Hui C, Fauzi N, Jasamai M, Kumolosasi E. Viability and apoptotic effects of green tea (Camellia sinensis) methanol extract on human leukemic cell lines. Acta Pol Pharm. (2018) 75:51–8.
74. Monga J, Aggarwal V, Suthar S, Monika Nongalleima K, Sharma M. Topical (+)-catechin emulsified gel prevents DMBA/TPA-induced squamous cell carcinoma of the skin by modulating antioxidants and inflammatory biomarkers in BALB/c mice. Food Funct. (2014) 5:3197–207. doi: 10.1039/C4FO00531G
75. Sánchez-Tena S, Alcarraz-Vizán G, Marín S, Torres J, Cascante M. Epicatechin gallate impairs colon cancer cell metabolic productivity. J Agr Food Chem. (2013) 61:4310–7. doi: 10.1021/jf3052785
76. Hsieh C, Lu C, Kuo Y, Chen W, Chao C. Studies on the non-invasive anticancer remedy of the triple combination of epigallocatechin gallate, pulsed electric field, and ultrasound. PLoS One. (2018) 13:e201920. doi: 10.1371/journal.pone.0201920
77. Grube S, Ewald C, Kögler C, Lawson Mclean A, Kalff R, Walter J. Achievable central nervous system concentrations of the green tea catechin egcg induce stress in glioblastoma cells in vitro. Nutr Cancer. (2018) 70:1145–58. doi: 10.1080/01635581.2018.1495239
78. Zhang S, Cao M, Fang F. The role of epigallocatechin-3-gallate in autophagy and endoplasmic reticulum stress (ERS)-induced apoptosis of human diseases. Med Sci Monitor. (2020) 26:e924558. doi: 10.12659/MSM.924558
79. Zhao L, Liu S, Xu J, Li W, Duan G, Wang H, et al. A new molecular mechanism underlying the EGCG-mediated autophagic modulation of AFP in HepG2 cells. Cell Death Dis. (2017) 8:e3160. doi: 10.1038/cddis.2017.563
80. Yuan C, Horng C, Lee C, Chiang N, Tsai F, Lu C, et al. Epigallocatechin gallate sensitizes cisplatin-resistant oral cancer CAR cell apoptosis and autophagy through stimulating AKT/STAT3 pathway and suppressing multidrug resistance 1 signaling. Environ Toxicol. (2017) 32:845–55. doi: 10.1002/tox.22284
81. Enkhbat T, Nishi M, Yoshikawa K, Jun H, Tokunaga T, Takasu C, et al. Epigallocatechin-3-gallate enhances radiation sensitivity in colorectal cancer cells through Nrf2 activation and autophagy. Anticancer Res. (2018) 38:6247–52. doi: 10.21873/anticanres.12980
82. Shayeghan M, Ansari A, Forouzesh F, Javidi M. Reactive oxygen species, the trident of Neptune in the hands of hecate; role in different diseases, signaling pathways, and detection methods. Arch Biochem Biophys. (2022) 728:109357. doi: 10.1016/j.abb.2022.109357
83. Zhou Y, Zheng J, Li Y, Xu D, Li S, Chen Y, et al. Natural polyphenols for prevention and treatment of cancer. Nutrients. (2016) 8:515. doi: 10.3390/nu8080515
84. Ravishankar D, Rajora A, Greco F, Osborn H. Flavonoids as prospective compounds for anti-cancer therapy. Int J Biochem Cell Biol. (2013) 45:2821–31. doi: 10.1016/j.biocel.2013.10.004
86. Geetha T, Garg A, Chopra K, Pal Kaur I. Delineation of antimutagenic activity of catechin, epicatechin and green tea extract. Mutat Res. (2004) 556:65–74. doi: 10.1016/j.mrfmmm.2004.07.003
87. Sang S, Cheng X, Stark R, Rosen R, Yang C, Ho C. Chemical studies on antioxidant mechanism of tea catechins: analysis of radical reaction products of catechin and epicatechin with 2,2-diphenyl-1-picrylhydrazyl. Bioorgan Med Chem. (2002) 10:2233–7. doi: 10.1016/S0968-0896(02)00089-5
88. Zhu N, Huang T, Yu Y, Lavoie E, Yang C, Ho C. Identification of oxidation products of (–)-epigallocatechin gallate and (–)-epigallocatechin with H2O2. J Agr Food Chem. (2000) 48:979–81. doi: 10.1021/jf991188c
89. Zhu N, Wang M, Wei G, Lin J, Yang C, Ho C. Identification of reaction products of (–)-epigallocatechin, (–)-epigallocatechin gallate and pyrogallol with 2,2-diphenyl-1-picrylhydrazyl radical. Food Chem. (2001) 73:345–9. doi: 10.1016/S0308-8146(00)00308-3
90. Meneses-Gutiérrez C, Hernández-Damián J, Pedraza-Chaverri J, Guerrero-Legarreta I, Téllez D, Jaramillo-Flores M. Antioxidant capacity and cytotoxic effects of catechins and resveratrol oligomers produced by enzymatic oxidation against T24 human urinary bladder cancer cells. Antioxidants (Basel). (2019) 8:214. doi: 10.3390/antiox8070214
91. Chow J, Liu J, Che Y, Hsieh M, Kao P, Cheng J, et al. The effects of catechin on superoxide dismutase activity and its gene expression in pheochromocytoma cells. Zhonghua Yi Xue Za Zhi (Taipei). (2002) 65:138–43.
92. Ishii T, Mori T, Tanaka T, Mizuno D, Yamaji R, Kumazawa S, et al. Covalent modification of proteins by green tea polyphenol (–)-epigallocatechin-3-gallate through autoxidation. Free Radical Biol Med. (2008) 45:1384–94. doi: 10.1016/j.freeradbiomed.2008.07.023
93. O’Sullivan J, Sheridan J, Mulcahy H, Tenniswood M, Morrissey C. The effect of green tea on oxidative damage and tumour formation in lobund-wistar rats. Eur J Cancer Prev. (2008) 17:489–501. doi: 10.1097/CEJ.0b013e3282f0c04e
94. Kager N, Ferk F, Kundi M, Wagner K, Misík M, Knasmüller S. Prevention of oxidative DNA damage in inner organs and lymphocytes of rats by green tea extract. Eur J Nutr. (2010) 49:227–34. doi: 10.1007/s00394-009-0068-0
95. Forester S, Lambert J. Antioxidant effects of green tea. Mol Nutr Food Res. (2011) 55:844. doi: 10.1002/mnfr.201000641
96. Choudhary S, Sood S, Donnell R, Wang H. Intervention of human breast cell carcinogenesis chronically induced by 2-amino-1-methyl-6-phenylimidazo[4,5-b]pyridine. Carcinogenesis. (2012) 33:876–85. doi: 10.1093/carcin/bgs097
97. Son Y, Cheong Y, Kim N, Chung H, Kang D, Pae H. Mitogen-activated protein kinases and reactive oxygen species: how can ROS activate MAPK pathways? J Signal Transduct. (2011) 2011:792639. doi: 10.1155/2011/792639
98. Chambers A, Groom A, Macdonald I. Dissemination and growth of cancer cells in metastatic sites. Nat Rev Cancer. (2002) 2:563–72. doi: 10.1038/nrc865
99. Yuan J, Li Y, Yang X. Inhibition of epigallocatechin gallate on orthotopic colon cancer by upregulating the Nrf2-UGT1A signal pathway in nude mice. Pharmacology. (2007) 80:269–78. doi: 10.1159/000106447
100. Zhang J, Lei Z, Huang Z, Zhang X, Zhou Y, Luo Z, et al. Epigallocatechin-3-gallate (EGCG) suppresses melanoma cell growth and metastasis by targeting TRAF6 activity. Oncotarget. (2016) 7:79557–71. doi: 10.18632/oncotarget.12836
101. Chen S, Yao X, Peng B, Xu Y, Wang G, Huang J, et al. Epigallocatechin-3-gallate inhibits migration and invasion of human renal carcinoma cells by downregulating matrix metalloproteinase-2 and matrix metalloproteinase-9. Exp Ther Med. (2016) 11:1243–8. doi: 10.3892/etm.2016.3050
102. Luo K, Chen W, Lung W, Wei X, Cheng B, Cai Z, et al. EGCG inhibited bladder cancer SW780 cell proliferation and migration both in vitro and in vivo via down-regulation of NF-κB and MMP-9. J Nutr Biochem. (2017) 41:56–64. doi: 10.1016/j.jnutbio.2016.12.004
103. Ho Y, Yang S, Peng C, Chou M, Chang Y. Epigallocatechin-3-gallate inhibits the invasion of human oral cancer cells and decreases the productions of matrix metalloproteinases and urokinase-plasminogen activator. J Oral Pathol Med. (2007) 36:588–93. doi: 10.1111/j.1600-0714.2007.00588.x
104. Ho H, Huang C, Lu Y, Yeh C, Ho Y, Yang S, et al. Epigallocatechin-3-gallate inhibits migration of human nasopharyngeal carcinoma cells by repressing MMP-2 expression. J Cell Physiol. (2019) 234:20915–24. doi: 10.1002/jcp.28696
105. Zhang Y, Owusu L, Duan W, Jiang T, Zang S, Ahmed A, et al. Anti-metastatic and differential effects on protein expression of epigallocatechin-3-gallate in HCCLM6 hepatocellular carcinoma cells. Int J Mol Med. (2013) 32:959–64. doi: 10.3892/ijmm.2013.1446
106. Maeda-Yamamoto M, Suzuki N, Sawai Y, Miyase T, Sano M, Hashimoto-Ohta A, et al. Association of suppression of extracellular signal-regulated kinase phosphorylation by epigallocatechin gallate with the reduction of matrix metalloproteinase activities in human fibrosarcoma HT1080 cells. J Agr Food Chem. (2003) 51:1858–63. doi: 10.1021/jf021039l
107. Deng Y, Lin J. EGCG inhibits the invasion of highly invasive CL1-5 lung cancer cells through suppressing MMP-2 expression via JNK signaling and induces G2/M arrest. J Agr Food Chem. (2011) 59:13318–27. doi: 10.1021/jf204149c
108. Deb G, Thakur V, Limaye A, Gupta S. Epigenetic induction of tissue inhibitor of matrix metalloproteinase-3 by green tea polyphenols in breast cancer cells. Mol Carcinogen. (2015) 54:485–99. doi: 10.1002/mc.22121
109. Hong G, Wu H, Ma S, Su Z. Catechins from oolong tea improve uterine defects by inhibiting STAT3 signaling in polycystic ovary syndrome mice. Chin Med. (2020) 15:125. doi: 10.1186/s13020-020-00405-y
110. Slivova V, Zaloga G, Demichele S, Mukerji P, Huang Y, Siddiqui R, et al. Green tea polyphenols modulate secretion of urokinase plasminogen activator (uPA) and inhibit invasive behavior of breast cancer cells. Nutr Cancer. (2005) 52:66–73. doi: 10.1207/s15327914nc5201_9
111. Shin S, Kim M, Jung W, Chong Y. (–)-Epigallocatechin gallate derivatives reduce the expression of both urokinase plasminogen activator and plasminogen activator inhibitor-1 to inhibit migration, adhesion, and invasion of MDA-MB-231 cells. Phytother Res. (2018) 32:2086–96. doi: 10.1002/ptr.6154
112. Siddiqui I, Malik A, Adhami V, Asim M, Hafeez B, Sarfaraz S, et al. Green tea polyphenol EGCG sensitizes human prostate carcinoma LNCaP cells to TRAIL-mediated apoptosis and synergistically inhibits biomarkers associated with angiogenesis and metastasis. Oncogene. (2008) 27:2055–63. doi: 10.1038/sj.onc.1210840
113. Wei R, Penso N, Hackman R, Wang Y, Mackenzie G. Epigallocatechin-3-gallate (EGCG) suppresses pancreatic cancer cell growth, invasion, and migration partly through the inhibition of Akt pathway and epithelial-mesenchymal transition: enhanced efficacy when combined with gemcitabine. Nutrients. (2019) 11:1856. doi: 10.3390/nu11081856
114. Takahashi A, Watanabe T, Mondal A, Suzuki K, Kurusu-Kanno M, Li Z, et al. Mechanism-based inhibition of cancer metastasis with (–)-epigallocatechin gallate. Biochem Biophys Res Commun. (2014) 443:1–6. doi: 10.1016/j.bbrc.2013.10.094
115. Li T, Zhao N, Lu J, Zhu Q, Liu X, Hao F, et al. Epigallocatechin gallate (EGCG) suppresses epithelial-mesenchymal transition (EMT) and invasion in anaplastic thyroid carcinoma cells through blocking of TGF-beta 1/Smad signaling pathways. Bioengineered. (2019) 10:282–91. doi: 10.1080/21655979.2019.1632669
116. Fujiki H, Sueoka E, Rawangkan A, Suganuma M. Human cancer stem cells are a target for cancer prevention using (–)-epigallocatechin gallate. J Cancer Res Clin. (2017) 143:2401–12. doi: 10.1007/s00432-017-2515-2
117. Sicard A, Dao T, Suarez N, Annabi B. Diet-derived gallated catechins prevent TGF-β-mediated epithelial-mesenchymal transition, cell migration and vasculogenic mimicry in chemosensitive ES-2 ovarian cancer cells. Nutr Cancer. (2021) 73:169–80. doi: 10.1080/01635581.2020.1733624
118. Suganuma M, Takahashi A, Watanabe T, Iida K, Matsuzaki T, Yoshikawa H, et al. Biophysical approach to mechanisms of cancer prevention and treatment with green tea catechins. Molecules. (2016) 21:1566. doi: 10.3390/molecules21111566
119. Stacker S, Caesar C, Baldwin M, Thornton G, Williams R, Prevo R, et al. VEGF-D promotes the metastatic spread of tumor cells via the lymphatics. Nat Med. (2001) 7:186–91. doi: 10.1038/84635
120. Mineva N, Paulson K, Naber S, Yee A, Sonenshein G. Epigallocatechin-3-gallate inhibits stem-like inflammatory breast cancer cells. PLoS One. (2013) 8:e73464. doi: 10.1371/journal.pone.0073464
121. Maruyama T, Murata S, Nakayama K, Sano N, Ogawa K, Nowatari T, et al. (–)-Epigallocatechin-3-gallate suppresses liver metastasis of human colorectal cancer. Oncol Rep. (2014) 31:625–33. doi: 10.3892/or.2013.2925
122. Shankar S, Marsh L, Srivastava R. EGCG inhibits growth of human pancreatic tumors orthotopically implanted in Balb C nude mice through modulation of FKHRL1/FOXO3a and neuropilin. Mol Cell Biochem. (2013) 372:83–94. doi: 10.1007/s11010-012-1448-y
123. Wu D, Liu Z, Li J, Zhang Q, Zhong P, Teng T, et al. Epigallocatechin-3-gallate inhibits the growth and increases the apoptosis of human thyroid carcinoma cells through suppression of EGFR/RAS/RAF/MEK/ERK signaling pathway. Cancer Cell Int. (2019) 19:43. doi: 10.1186/s12935-019-0762-9
124. Rawangkan A, Wongsirisin P, Namiki K, Iida K, Kobayashi Y, Shimizu Y, et al. Green tea catechin is an alternative immune checkpoint inhibitor that inhibits PD-L1 expression and lung tumor growth. Molecules. (2018) 23:2071. doi: 10.3390/molecules23082071
125. Ravindran Menon D, Li Y, Yamauchi T, Osborne D, Vaddi P, Wempe M, et al. EGCG inhibits tumor growth in melanoma by targeting JAK-STAT signaling and its downstream PD-L1/PD-L2-PD1 axis in tumors and enhancing cytotoxic T-cell responses. Pharmaceuticals. (2021) 14:1081. doi: 10.3390/ph14111081
126. Rawangkan A, Iida K, Sakai R, Fujiki H, Suganuma M. Green tea catechin, EGCG, enhances antitumor immunity by down-regulation of PD-L1 expression in non-small human lung cancer cell lines. Cancer Res. (2017) 77:2665. doi: 10.1158/1538-7445.AM2017-2665
127. Mccarty M, Iloki-Assanga S, Lewis Lujany L. Nutraceutical targeting of TLR4 signaling has potential for prevention of cancer cachexia. Med Hypotheses. (2019) 132:10926. doi: 10.1016/j.mehy.2019.109326
128. Kumazoe M, Yamashita M, Nakamura Y, Takamatsu K, Bae J, Yamashita S, et al. Green tea polyphenol EGCG upregulates tollip expression by suppressing Elf-1 expression. J Immunol. (2017) 199:3261–9. doi: 10.4049/jimmunol.1601822
129. Li T, Li F, Liu X, Liu J, Li D. Synergistic anti-inflammatory effects of quercetin and catechin via inhibiting activation of TLR4-MyD88-mediated NF-κB and MAPK signaling pathways. Phytother Res. (2019) 33:756–67. doi: 10.1002/ptr.6268
130. Song Q, Zhang G, Wang B, Cao G, Li D, Wang Y, et al. Reinforcing the combinational immuno-oncotherapy of switching “cold” tumor to “hot” by responsive penetrating nanogels. Acs Appl Mater Inter. (2021) 13:36824–38. doi: 10.1021/acsami.1c08201
131. Rogovskii V, Popov S, Sturov N, Shimanovskii N. The possibility of preventive and therapeutic use of green tea catechins in prostate cancer. Anticancer Agents Med Chem. (2019) 19:1223–31. doi: 10.2174/1871520619666190404153058
132. Gonzalez Suarez N, Fernandez-Marrero Y, Torabidastgerdooei S, Annabi B. EGCG prevents the onset of an inflammatory and cancer-associated adipocyte-like phenotype in adipose-derived mesenchymal stem/stromal cells in response to the triple-negative breast cancer secretome. Nutrients. (2022) 14:1099. doi: 10.3390/nu14051099
133. Pan P, Huang Y, Oshima K, Yearsley M, Zhang J, Arnold M, et al. The immunomodulatory potential of natural compounds in tumor-bearing mice and humans. Crit Rev Food Sci. (2019) 59:992–1007. doi: 10.1080/10408398.2018.1537237
134. Zheng Y, Viswanathan B, Kesarwani P, Mehrotra S. Dietary agents in cancer prevention: an immunological perspective. Photochem Photobiol. (2012) 88:1083–98. doi: 10.1111/j.1751-1097.2012.01128.x
135. Santilli G, Anderson J, Thrasher A, Sala A. Catechins and antitumor immunity: not MDSC’s cup of tea. Oncoimmunology. (2013) 2:e24443. doi: 10.4161/onci.24443
136. Xu P, Yan F, Zhao Y, Chen X, Sun S, Wang Y, et al. Green tea polyphenol EGCG attenuates MDSCs-mediated immunosuppression through canonical and non-canonical pathways in a 4t1 murine breast cancer model. Nutrients. (2020) 12:1042. doi: 10.3390/nu12041042
137. Mukherjee S, Hussaini R, White R, Atwi D, Fried A, Sampat S, et al. Tricurin, a synergistic formulation of curcumin, resveratrol, and epicatechin gallate, repolarizes tumor-associated macrophages and triggers an immune response to cause suppression of HPV+ tumors. Cancer Immunol Immun. (2018) 67:761–74. doi: 10.1007/s00262-018-2130-3
138. Patel S, Akalkotkar A, Bivona J, Lee J, Park Y, Yu M, et al. Vitamin A or E and a catechin synergize as vaccine adjuvant to enhance immune responses in mice by induction of early interleukin-15 but not interleukin-1 beta responses. Immunology. (2016) 148:352–62. doi: 10.1111/imm.12614
139. Yang Y, Han X, Chen Y, Wu J, Li M, Yang H, et al. EGCG induces pro-inflammatory response in macrophages to prevent bacterial infection through the 67LR/p38/JNK signaling pathway. J Agr Food Chem. (2021) 69:5638–51. doi: 10.1021/acs.jafc.1c01353
140. Chen L, Teng H, Jia Z, Battino M, Miron A, Yu Z, et al. Intracellular signaling pathways of inflammation modulated by dietary flavonoids: the most recent evidence. Crit Rev Food Sci. (2018) 58:2908–24. doi: 10.1080/10408398.2017.1345853
141. Sunil M, Sunitha V, Santhakumaran P, Mohan M, Jose M, Radhakrishnan E, et al. Protective effect of (+)-catechin against lipopolysaccharide-induced inflammatory response in RAW 264.7 cells through downregulation of NF-kappa B and p38 MAPK. Inflammopharmacology. (2021) 29:1139–55. doi: 10.1007/s10787-021-00827-6
142. Pasala P, Reddy L, Silvia N, Reddy Y, Sampath A, Dorababu N, et al. Molecular docking and in vivo immunomodulatory activity of Albizia procera bark on doxorubicin induced immunosuppressive rats. J King Saud Univ Sci. (2022) 34:101828. doi: 10.1016/j.jksus.2022.101828
143. Huang S, Kao Y, Shih S, Tsai M, Lin C, Chen L, et al. Epigallocatechin-3-gallate exhibits immunomodulatory effects in human primary T cells. Biochem Biophys Res Commun. (2021) 550:70–6. doi: 10.1016/j.bbrc.2021.02.132
144. Cheng A, Tan X, Sun J, Gu C, Liu C, Guo X. Catechin attenuates TNF-α induced inflammatory response via AMPK-SIRT1 pathway in 3T3-L1 adipocytes. PLoS One. (2019) 14:e217090. doi: 10.1371/journal.pone.0217090
145. Lagha A, Grenier D. Tea polyphenols protect gingival keratinocytes against TNF-α-induced tight junction barrier dysfunction and attenuate the inflammatory response of monocytes/macrophages. Cytokine. (2019) 115:64–75. doi: 10.1016/j.cyto.2018.12.009
146. Ganeshpurkar A, Saluja A. Immunomodulatory effect of rutin, catechin, and hesperidin on macrophage function. Indian J Biochem Bio. (2020) 57:58–63.
147. Wu D, Lewis E, Pae M, Meydani S. Nutritional modulation of immune function: analysis of evidence, mechanisms, and clinical relevance. Front Immunol. (2019) 9:3160. doi: 10.3389/fimmu.2018.03160
148. Almaguer G, Ortiz-Vilchis P, Cordero P, Martinez-Vega R, Perez-Duran J, Meaney E, et al. Anticancer potential of (–)-epicatechin in a triple-negative mammary gland model. J Pharm Pharmacol. (2021) 73:1675–82. doi: 10.1093/jpp/rgab133
149. Ma X, Li M, Lu G, Wang R, Wei Y, Guo Y, et al. Anti-inflammation of epicatechin mediated by TMEM35A and TMPO in bovine mammary epithelial cell line cells and mouse mammary gland. J Dairy Sci. (2021) 104:12925–38. doi: 10.3168/jds.2021-20571
150. Cordero-Herrera I, Chen X, Ramos S, Devaraj S. (–)-Epicatechin attenuates high-glucose-induced inflammation by epigenetic modulation in human monocytes. Eur J Nutr. (2017) 56:1369–73. doi: 10.1007/s00394-015-1136-2
151. Stadlbauer S, Steinborn C, Klemd A, Hattori F, Ohmori K, Suzuki K, et al. Impact of green tea catechin ECG and its synthesized fluorinated analogue on prostate cancer cells and stimulated immunocompetent cells. Planta Med. (2018) 84:813–9. doi: 10.1055/s-0044-102099
152. Chen A, Jiang P, Zeb F, Wu X, Xu C, Chen L, et al. EGCG regulates CTR1 expression through its pro-oxidative property in non-small-cell lung cancer cells. J Cell Physiol. (2020) 23:7970–81. doi: 10.1002/jcp.29451
153. Jiang P, Wu X, Wang X, Huang W, Feng Q. NEAT1 upregulates EGCG-induced CTR1 to enhance cisplatin sensitivity in lung cancer cells. Oncotarget. (2016) 7:43337–51. doi: 10.18632/oncotarget.9712
154. Jiang P, Chen A, Wu X, Zhou M, Ul Haq I, Mariyam Z, et al. NEAT1 acts as an inducer of cancer stem cell-like phenotypes in NSCLC by inhibiting EGCG-upregulated CTR1. J Cell Physiol. (2018) 233:4852–63. doi: 10.1002/jcp.26288
155. Jiang P, Xu C, Chen L, Chen A, Wu X, Zhou M, et al. EGCG inhibits CSC-like properties through targeting miR-485/CD44 axis in A549-cisplatin resistant cells. Mol Carcinogen. (2018) 57:1835–44. doi: 10.1002/mc.22901
156. Heyza J, Arora S, Zhang H, Conner K, Lei W, Floyd A, et al. Targeting the DNA repair endonuclease ERCC1-XPF with green tea polyphenol epigallocatechin-3-gallate (EGCG) and its prodrug to enhance cisplatin efficacy in human cancer cells. Nutrients. (2018) 10:1644. doi: 10.3390/nu10111644
157. Xue M, Liu X, Cheng B, Rui X, Wu M, Lv J. Epigallocatechin gallate enhances inhibition effect of DDP on the proliferation of gastric cancer BGC-823 cells by regulating p19Arf-p53-p21Cip1 signaling pathway. Asian Pac J Cancer Prev. (2021) 22:1263–70. doi: 10.31557/APJCP.2021.22.4.1263
158. Li Y, Wu S, Lu S, Chen F, Guo Y, Gan S, et al. (–)-Epigallocatechin-3-gallate inhibits nasopharyngeal cancer stem cell self-renewal and migration and reverses the epithelial-mesenchymal transition via NF-kappa B p65 inactivation. Tumor Biol. (2015) 36:2747–61. doi: 10.1007/s13277-014-2899-4
159. Fatima S, Al-Mohaimeed N, Al-Shaikh Y, Tyagi P, Banu N, Hasan S, et al. Combined treatment of epigallocatechin gallate and coenzyme Q10 attenuates cisplatin-induced nephrotoxicity via suppression of oxidative/nitrosative stress, inflammation and cellular damage. Food Chem Toxicol. (2016) 94:213–20. doi: 10.1016/j.fct.2016.05.023
160. Arafa M, Atteia H. Protective role of epigallocatechin gallate in a rat model of cisplatin-induced cerebral inflammation and oxidative damage: impact of modulating NF-κB and Nrf2. Neurotox Res. (2020) 37:380–96. doi: 10.1007/s12640-019-00095-x
161. Hu F, Wei F, Wang Y, Wu B, Fang Y, Xiong B. EGCG synergizes the therapeutic effect of cisplatin and oxaliplatin through autophagic pathway in human colorectal cancer cells. J Pharmacol Sci. (2015) 128:27–34. doi: 10.1016/j.jphs.2015.04.003
162. Navarro-Hortal M, Varela-López A, Romero-Márquez J, Rivas-García L, Speranza L, Battino M, et al. Role of flavonoids against adriamycin toxicity. Food Chem Toxicol. (2020) 146:111820. doi: 10.1016/j.fct.2020.111820
163. Fabbri R, Macciocca M, Vicenti R, Caprara G, Piccinni M, Paradisi R, et al. Epigallocatechin-3-gallate inhibits doxorubicin-induced inflammation on human ovarian tissue. Bioscience Rep. (2019) 39:BSR20181424. doi: 10.1042/BSR20181424
164. Wei R, Wirkus J, Yang Z, Machuca J, Esparza Y, Mackenzie G. EGCG sensitizes chemotherapeutic-induced cytotoxicity by targeting the ERK pathway in multiple cancer cell lines. Arch Biochem Biophys. (2020) 692:108546. doi: 10.1016/j.abb.2020.108546
165. Satonaka H, Ishida K, Takai M, Koide R, Shigemasa R, Ueyama J, et al. (–)-Epigallocatechin-3-gallate down-regulates doxorubicin-induced overexpression of p-glycoprotein through the coordinate inhibition of PI3K/Akt and MEK/ERK signaling pathways. Anticancer Res. (2017) 37:6071–7. doi: 10.21873/anticanres.12055
166. Li H, Krstin S, Wink M. Modulation of multidrug resistant in cancer cells by EGCG, tannic acid and curcumin. Phytomedicine. (2018) 50:213–22. doi: 10.1016/j.phymed.2018.09.169
167. Chen L, Ye H, Zhang G, Yao W, Chen X, Zhang F, et al. Autophagy inhibition contributes to the synergistic interaction between EGCG and doxorubicin to kill the hepatoma Hep3B cells. PLoS One. (2014) 9:e85771. doi: 10.1371/journal.pone.0085771
168. Wang W, Chen D, Zhu K. SOX2OT variant 7 contributes to the synergistic interaction between EGCG and Doxorubicin to kill osteosarcoma via autophagy and stemness inhibition. J Exp Clin Canc Res. (2018) 37:37. doi: 10.1186/s13046-018-0689-3
169. Minnelli C, Laudadio E, Mobbili G, Galeazzi R. Conformational insight on WT- and mutated-EGFR receptor activation and inhibition by epigallocatechin-3-gallate: over a rational basis for the design of selective non-small-cell lung anticancer agents. Int J Mol Sci. (2020) 21:1721. doi: 10.3390/ijms21051721
170. Zhang X, Zhang H, Tighiouart M, Lee J, Shin H, Khuri F, et al. Synergistic inhibition of head and neck tumor growth by green tea (–)-epigallocatechin-3-gallate and EGFR tyrosine kinase inhibitor. Int J Cancer. (2008) 123:1005–14. doi: 10.1002/ijc.23585
171. Amin A, Khuri F, Chen Z, Shin D. Synergistic growth inhibition of squamous cell carcinoma of the head and neck by erlotinib and epigallocatechin-3-gallate: the role of p53-dependent inhibition of nuclear factor-κB. Cancer Prev Res. (2009) 2:538–45. doi: 10.1158/1940-6207.CAPR-09-0063
172. Haque A, Rahman M, Chen Z, Saba N, Khuri F, Shin D, et al. Combination of erlotinib and EGCG induces apoptosis of head and neck cancers through posttranscriptional regulation of Bim and Bcl-2. Apoptosis. (2015) 20:986–95. doi: 10.1007/s10495-015-1126-0
173. Milligan S, Burke P, Coleman D, Bigelow R, Steffan J, Carroll J, et al. The green tea polyphenol EGCG potentiates the antiproliferative activity of c-Met and epidermal growth factor receptor inhibitors in non–small cell lung cancer cells. Clin Cancer Res. (2009) 15:4885–94. doi: 10.1158/1078-0432.CCR-09-0109
174. Shin D, Nannapaneni S, Patel M, Shi Q, Liu Y, Chen Z, et al. Phase Ib study of chemoprevention with green tea Polyphenon E and Erlotinib in patients with advanced premalignant lesions (APL) of the head and neck. Clin Cancer Res. (2020) 26:5860–8. doi: 10.1158/1078-0432.CCR-20-2276
175. Wang P, Henning S, Heber D, Vadgama J. Sensitization to docetaxel in prostate cancer cells by green tea and quercetin. J Nutri Biochem. (2015) 26:408–15. doi: 10.1016/j.jnutbio.2014.11.017
176. Jesus Nunez-Iglesias M, Novio S, García C, Pérez-Muñuzuri M, Martínez M, Santiago J, et al. Co-adjuvant therapy efficacy of catechin and procyanidin B2 with docetaxel on hormone-related cancers in vitro. Int J Mol Sci. (2021) 22:7178. doi: 10.3390/ijms22137178
177. Wu H, Xin Y, Xiao Y, Zhao J. Low-dose docetaxel combined with (–)-epigallocatechin-3-gallate inhibits angiogenesis and tumor growth in nude mice with gastric cancer xenografts. Cancer Biother Radio. (2012) 27:204–9. doi: 10.1089/cbr.2011.1103
178. Jackson J, Letchford K. The effective solubilization of hydrophobic drugs using epigallocatechin gallate or tannic acid-based formulations. J Pharm Sci. (2016) 105:3143–52. doi: 10.1016/j.xphs.2016.06.027
179. Wu W, Dong J, Gou H, Geng R, Yang X, Chen D, et al. EGCG synergizes the therapeutic effect of irinotecan through enhanced DNA damage in human colorectal cancer cells. J Cell Mol Med. (2021) 25:7913–21. doi: 10.1111/jcmm.16718
180. Lin L, Wang M, Tsai T. Food–drug interaction of (–)-epigallocatechin-3-gallate on the pharmacokinetics of irinotecan and the metabolite SN-38. Chem-Biol Interact. (2008) 174:177–82. doi: 10.1016/j.cbi.2008.05.033
181. Borah G, Bharali M. Green tea catechins in combination with irinotecan attenuates tumorigenesis and treatment-associated toxicity in an inflammation-associated colon cancer mice model. J Egypt Natl Canc Inst. (2021) 33:17. doi: 10.1186/s43046-021-00074-4
182. Vaillancourt K, Ben Lagha A, Grenier D. A green tea extract and epigallocatechin-3-gallate attenuate the deleterious effects of irinotecan in an oral epithelial cell model. Arch Oral Biol. (2021) 126:105035. doi: 10.1016/j.archoralbio.2021.105135
183. Huang W, Ding L, Huang Q, Hu H, Liu S, Yang X, et al. Carbonyl reductase 1 as a novel target of (–)-epigallocatechin gallate against hepatocellular carcinoma. Hepatology. (2010) 52:703–14. doi: 10.1002/hep.23723
184. Wang E, Barecki-Roach M, Johnson W. Elevation of P-glycoprotein function by a catechin in green tea. Biochem Biophys Res Commun. (2002) 297:412–8. doi: 10.1016/S0006-291X(02)02219-2
185. Toth E, Erdodi F, Kiss A. Activation of myosin phosphatase by epigallocatechin-gallate sensitizes THP-1 leukemic cells to daunorubicin. Anticancer Agents Med Chem. (2021) 21:1092–8. doi: 10.2174/1871520620666200717142315
186. Volta V, Ranzato E, Martinotti S, Gallo S, Russo M, Mutti L, et al. Preclinical demonstration of synergistic active nutrients/drug (AND) combination as a potential treatment for malignant pleural mesothelioma. PLoS One. (2013) 8:e58051. doi: 10.1371/journal.pone.0058051
187. Tang S, Fu J, Shankar S, Srivastava R. EGCG enhances the therapeutic potential of gemcitabine and CP690550 by inhibiting STAT3 signaling pathway in human pancreatic cancer. PLoS One. (2012) 7:e31067. doi: 10.1371/journal.pone.0031067
188. Martinotti S, Ranzato E, Parodi M, Vitale M, Burlando B. Combination of ascorbate/epigallocatechin-3-gallate/gemcitabine synergistically induces cell cycle deregulation and apoptosis in mesothelioma cells. Toxicol Appl Pharm. (2014) 274:35–41. doi: 10.1016/j.taap.2013.10.025
189. Teschke R, Xuan T. How can green tea polyphenols affect drug metabolism and should we be concerned? Expert Opin Drug Metab Toxicol. (2019) 15:989–91. doi: 10.1080/17425255.2019.1697228
190. Pons-Fuster Lopez E, Gomez Garcia F, Lopez Jornet P. Combination of 5-Florouracil and polyphenol EGCG exerts suppressive effects on oral cancer cells exposed to radiation. Arch Oral Biol. (2019) 101:8–12. doi: 10.1016/j.archoralbio.2019.02.018
191. Tang H, Zeng L, Wang J, Zhang X, Ruan Q, Wang J, et al. Reversal of 5-fluorouracil resistance by EGCG is mediate by inactivation of TFAP2A/VEGF signaling pathway and down-regulation of MDR-1 and P-gp expression in gastric cancer. Oncotarget. (2017) 8:82842–53. doi: 10.18632/oncotarget.20666
192. Pyrko P, Schönthal A, Hofman F, Chen T, Lee A. The unfolded protein response regulator GRP78/BiP as a novel target for increasing chemosensitivity in malignant gliomas. Cancer Res. (2007) 67:9809–16. doi: 10.1158/0008-5472.CAN-07-0625
193. La X, Zhang L, Li Z, Li H, Yang Y. (–)-epigallocatechin gallate (EGCG) enhances the sensitivity of colorectal cancer cells to 5-FU by inhibiting GRP78/NF-κB/miR-155-5p/MDR1 pathway. J Agr Food Chem. (2019) 67:2510–8. doi: 10.1021/acs.jafc.8b06665
194. Hassanein N, Hassan E, Hegab A, Elahl H. Chemopreventive effect of sulindac in combination with epigallocatechin gallate or kaempferol against 1,2-dimethyl hydrazine-induced preneoplastic lesions in rats: a comparative study. J Biochem Mol Toxic. (2018) 32:e22198. doi: 10.1002/jbt.22198
195. Suganuma M, Saha A, Fujiki H. New cancer treatment strategy using combination of green tea catechins and anticancer drugs. Cancer Sci. (2011) 102:317–23. doi: 10.1111/j.1349-7006.2010.01805.x
196. Ohishi T, Kishimoto Y, Miura N, Shiota G, Kohri T, Hara Y, et al. Synergistic effects of (–)-epigallocatechin gallate with sulindac against colon carcinogenesis of rats treated with azoxymethane. Cancer Lett. (2002) 177:49–56. doi: 10.1016/S0304-3835(01)00767-4
197. Chang C, Chang P, Tu M, Lu C, Kuo S, Amagaya S, et al. Epigallocatechin gallate sensitizes CAL-27 human oral squamous cell carcinoma cells to the anti-metastatic effects of gefitinib (Iressa) via synergistic suppression of epidermal growth factor receptor and matrix metalloproteinase-2. Oncol Rep. (2012) 28:1799–807. doi: 10.3892/or.2012.1991
198. Liu J, Zhong T, Yi P, Fan C, Zhang Z, Liang G, et al. A new epigallocatechin gallate derivative isolated from Anhua dark tea sensitizes the chemosensitivity of gefitinib via the suppression of PI3K/mTOR and epithelial-mesenchymal transition. Fitoterapia. (2020) 143:104590. doi: 10.1016/j.fitote.2020.104590
199. Xie C, You C, Zhang N, Sheng H, Zheng X. Epigallocatechin gallate preferentially inhibits o(6)-methylguanine DNA-methyltransferase expression in glioblastoma cells rather than in nontumor glial cells. Nutr Cancer. (2018) 70:1339–47. doi: 10.1080/01635581.2018.1539189
200. Zhang Y, Wang S, Ma J, Li H, Ye J, Xie S, et al. EGCG inhibits properties of glioma stem-like cells and synergizes with temozolomide through downregulation of P-glycoprotein inhibition. J Neurooncol. (2015) 121:41–52. doi: 10.1007/s11060-014-1604-1
201. Chen T, Wang W, Golden E, Thomas S, Sivakumar W, Hofman F, et al. Green tea epigallocatechin gallate enhances therapeutic efficacy of temozolomide in orthotopic mouse glioblastoma models. Cancer Lett. (2011) 302:100–8. doi: 10.1016/j.canlet.2010.11.008
202. Tsukamoto S, Kumazoe M, Huang Y, Lesnick C, Kay N, Shanafelt T, et al. SphK1 inhibitor potentiates the anti-cancer effect of EGCG on leukaemia cells. Br J Haematol. (2017) 178:153–5. doi: 10.1111/bjh.14119
203. Takahashi N, Kobayashi M, Ogura J, Yamaguchi H, Satoh T, Watanabe K, et al. Immunoprotective effect of epigallocatechin-3-gallate on oral anticancer drug-induced alpha-defensin reduction in Caco-2 cells. Biol Pharm Bull. (2014) 37:490–2. doi: 10.1248/bpb.b13-00700
204. Liu J, Chen S, Lin C, Tsai S, Liang Y. Inhibition of melanoma growth and metastasis by combination with (–)-epigallocatechin-3-gallate and dacarbazine in mice. J Cell Biochem. (2001) 83:631–42. doi: 10.1002/jcb.1261
205. Fujimura Y, Sumida M, Sugihara K, Tsukamoto S, Yamada K, Tachibana H. Green tea polyphenol EGCG sensing Motif on the 67-kDa laminin receptor. PLoS One. (2012) 7:e37942. doi: 10.1371/journal.pone.0037942
206. Kumazoe M, Hiroi S, Tanimoto Y, Miyakawa J, Yamanouchi M, Suemasu Y, et al. Cancer cell selective probe by mimicking EGCG. Biochem Biophys Res Commun. (2020) 525:974–81. doi: 10.1016/j.bbrc.2020.03.021
207. Negri A, Naponelli V, Rizzi F, Bettuzzi S. Molecular targets of epigallocatechin—gallate (EGCG): a special focus on signal transduction and cancer. Nutrients. (2018) 10:1936. doi: 10.3390/nu10121936
208. Tsukamoto S, Huang Y, Umeda D, Yamada S, Yamashita S, Kumazoe M, et al. 67-kDa laminin receptor-dependent protein phosphatase 2A (PP2A) activation elicits melanoma-specific antitumor activity overcoming drug resistance. J Biol Chem. (2014) 289:47. doi: 10.1074/jbc.M114.604983
209. Rashidi B, Malekzadeh M, Goodarzi M, Masoudifar A, Mirzaei H. Green tea and its anti-angiogenesis effects. Biomed Pharmacother. (2017) 89:949–56. doi: 10.1016/j.biopha.2017.01.161
210. Xiao X, Jiang K, Xu Y, Peng H, Wang Z, Liu S, et al. (–)-Epigallocatechin-3-gallate induces cell apoptosis in chronic myeloid leukaemia by regulating Bcr/Abl-mediated p38-MAPK/JNK and JAK2/STAT3/AKT signalling pathways. Clin Exp Pharmacol Physiol. (2019) 46:126–36. doi: 10.1111/1440-1681.13037
211. Xu F, Na L, Li Y, Chen L. Roles of the PI3K/AKT/mTOR signalling pathways in neurodegenerative diseases and tumours. Cell Biosci. (2020) 10:54. doi: 10.1186/s13578-020-00416-0
212. Van Aller G, Carson J, Tang W, Peng H, Zhao L, Copeland R, et al. Epigallocatechin gallate (EGCG), a major component of green tea, is a dual phosphoinositide-3-kinase/mTOR inhibitor. Biochem Biophys Res Commun. (2011) 406:194–9. doi: 10.1016/j.bbrc.2011.02.010
213. Yuan J, Dong X, Yap J, Hu J. The MAPK and AMPK signaling: interplay and implication in targeted cancer therapy. J Hematol Oncol. (2020) 13:113. doi: 10.1186/s13045-020-00949-4
214. Wang Z, Li Z, Ji H. Direct targeting of beta-catenin in the Wnt signaling pathway: current progress and perspectives. Med Res Rev. (2021) 41:2109–29. doi: 10.1002/med.21787
215. Goedeke J, Maier S, Eichenmueller M, Mueller-Hoecker J, von Schweinitz D, Kappler R. Epigallocatechin-3-gallate inhibits hepatoblastoma growth by reactivating the Wnt inhibitor SFRP1. Nutr Cancer. (2013) 65:1200–7. doi: 10.1080/01635581.2013.828085
216. Li A, Gu K, Wang Q, Chen X, Fu X, Wang Y, et al. Epigallocatechin-3-gallate affects the proliferation, apoptosis, migration and invasion of tongue squamous cell carcinoma through the hippo-TAZ signaling pathway. Int J Mol Med. (2018) 42:2615–27.
217. Rimkus T, Carpenter R, Qasem S, Chan M, Lo H. Targeting the sonic hedgehog signaling pathway: review of smoothened and GLI inhibitors. Cancers. (2016) 8:22. doi: 10.3390/cancers8020022
218. Talebi M, Talebi M, Farkhondeh T, Mishra G, 0lgün S, Samarghandian S. New insights into the role of the Nrf2 signaling pathway in green tea catechin applications. Phytother Res. (2021) 35:3078–112. doi: 10.1002/ptr.7033
219. Yang C, Wang H, Chen J, Zhang J. Effects of tea catechins on cancer signaling pathways. Enzymes. (2014) 36:195–221.
220. Della Via F, Shiraishi R, Santos I, Ferro K, Salazar-Terreros M, Franchi Junior G, et al. (–)-Epigallocatechin-3-gallate induces apoptosis and differentiation in leukaemia by targeting reactive oxygen species and PIN1. Sci Rep. (2021) 11:9103. doi: 10.1038/s41598-021-88478-z
221. Zinatizadeh M, Schock B, Chalbatani G, Zarandi P, Jalali S, Miri S. The nuclear factor kappa B (NF-kB) signaling in cancer development and immune diseases. Genes Dis. (2021) 8:287–97. doi: 10.1016/j.gendis.2020.06.005
222. Shirakami Y, Shimizu M. Possible mechanisms of green tea and its constituents against cancer. Molecules. (2018) 23:2284. doi: 10.3390/molecules23092284
223. Li Q, Kakizaki M, Kuriyama S, Sone T, Yan H, Nakaya N, et al. Green tea consumption and lung cancer risk: the Ohsaki study. Brit J Cancer. (2008) 99:1179–84. doi: 10.1038/sj.bjc.6604645
224. Il’Yasova D, Martin C, Sandler R. Tea intake and risk of colon cancer in African-Americans and whites: North Carolina colon cancer study. Cancer Causes Control. (2003) 14:767–72.
225. Luo J, Inoue M, Iwasaki M, Sasazuki S, Otani T, Ye W, et al. Green tea and coffee intake and risk of pancreatic cancer in a large-scale, population-based cohort study in Japan (JPHC study). Eur J Cancer Prev. (2007) 16:542–8. doi: 10.1097/CEJ.0b013e32809b4d30
226. Dominianni C, Huang W, Berndt S, Hayes R, Ahn J. Prospective study of the relationship between coffee and tea with colorectal cancer risk: the PLCO cancer screening trial. Br J Cancer. (2013) 109:1352–9. doi: 10.1038/bjc.2013.434
227. Ugai T, Matsuo K, Sawada N, Iwasaki M, Yamaji T, Shimazu T, et al. Coffee and green tea consumption and subsequent risk of acute myeloid leukemia and myelodysplastic syndromes in Japan. Int J Cancer. (2018) 142:1130–8. doi: 10.1002/ijc.31135
228. Zamora-Ros R, Cayssials V, Jenab M, Rothwell J, Fedirko V, Aleksandrova K, et al. Dietary intake of total polyphenol and polyphenol classes and the risk of colorectal cancer in the European prospective investigation into cancer and nutrition (EPIC) cohort. Eur J Epidemiol. (2018) 33:1063–75. doi: 10.1007/s10654-018-0408-6
Glossary
5-FU, 5-fluorouracil; 67LR, 67 kD laminin receptor; ABC, ATP-binding cassette transporter; ACF, aberrant crypt foci; ACHN, human renal cell carcinoma; AFP, α-fetal protein; AKR1C3, aldo-keto reductase family 1 member c3; Akt, protein kinase B; AMPK, adenosine 5′-monophosphate-activated protein kinase; AP-1, activator protein 1; ARE, antioxidant responsive element; Arg-1, arginase 1; ATP, adenosine triphosphate; ATP7A, ATPase copper transporting 7 alpha; Bax, Bcl2-associated x protein; BC, breast cancer; Bcl-2, B-cell lymphoma-2; Bim, Bcl-2 interacting mediator of cell death; BTC, biliary tract cancer; C, (+)-catechin; CAA, cancer-associated adipocyte; CAR, oral cancer cell-line; CBR1, carbonyl reductase 1; CCL, C-C motif ligand; CCR2, C-C motif chemokine receptor 2; CD4, cluster of differentiation 4; CDC2, cell division control protein 2; CDK, cyclin dependent kinase; CFS, cancer-free survival; CG, (+)-catechin-3-gallate; cGMP, cyclic guanosine monophosphate; CI, cumulative incidence; CIP1, cyclin dependent kinase interacting protein 1; CK1, casein kinase 1; CLL, chronic lymphocytic leukemia; COMT, human catechol-O-methyltransferase; COX-2, cyclooxygenase 2; CRC, colorectal cancer; CSCs, cancer stem cells; CTL, cytotoxic T-cells; CTR1, copper transport protein 1; DAPK2, death associated protein kinase 2; DCs, dendritic cells; DLL3, delta-like ligand 3; DMBA, 7,12-dimethylbenz(a)anthracene; DNR, daunorubicin; DOC, docetaxel; DOX, doxorubicin; DPPH, 2,2-diphenyl-1-picrylhydrazyl; dTMP, deoxythymidylate; dUMP, deoxyuridine monophosphate; EC, (−)-epicatechin; ECG, (−)-epicatechin gallate; ECM, extracellular matrix; EGC, (−)-epigallocatechin; EGCG, (−)-epigallocatechin gallate; EGCGD, epigallocatechin gallate derivative; EGF, epidermal growth factor; EGFR, epidermal growth factor receptor; Elf-1, ETS transcription factor; EMT, epithelial-mesenchymal transition; EPIC, European Prospective Investigation into Cancer and Nutrition; ER, estrogen receptor; ErbB2, Erb-b2 receptor tyrosine kinase 2; ERCC1/XPF, excision repair cross-complementation group 1/xeroderma pigmentosum group F; ERK, extracellular regulated protein kinases; FAK, focal adhesion kinase; FZD, Frizzled family of proteins; GADD153, growth arrest and DNA damage-inducible 153; GC, (+)-gallocatechin; GCG, (+)-gallocatechin-3-gallate; G-CSF, granulocyte colony-stimulating factor; GLI1, glioma-associated oncogene homolog 1; GRP78, 78-kd glucose-regulated protein; GSK3, glycogen synthase kinase 3; GSTM1, glutathione S-transferase mu 1; GTPs, green tea polyphenols; HCC, hepatic cell carcinoma; HepG(2), human hepatocellular carcinomas; HIF-1α, hypoxia inducible factor-1α; HO-1, heme oxygenase 1; HPV-16, human papillomavirus type 16; HR, hazard ratio; HSA, human serum albumin; IFN-γ, interferon γ; IkBα, inhibitor of NF-κB; IKK, Ikb kinase; IL-1R, interleukin-1 receptor; iNOS, inducible nitric oxide synthase; IRN, irinotecan; IRS, insulin receptor substrate; JNK, C-jun n-terminal kinase; Keap1, kelch like ech-associated protein 1; KIP1, kinesin-like protein 1; LC3, light chain 3; LDM, low-dose metronomic; LNCaP, human prostate cancer cell-line; LncRNA, long non-coding RNA; MAP3KI4, NF-κB induced kinase; MAPK, mitogen-activated protein kinase; MCP1, chemokine monocyte chemotactic protein 1; MDA, malondialdehyde; MDA-MB-231, breast cancer cell-line; MDM2, murine double minute 2; MDR1, multidrug resistance 1; MDSCs, myeloid-derived suppressor cells; MGMT, O-6-methylguanine DNA-methyltransferase; MMPs, matrix metalloproteinases; MnSOD, manganese superoxide dismutase; mTOR, mechanistic target of rapamycin; MyD88, myeloid differentiation primary response protein 88; MYPT1, myosin phosphatase target subunit; NAFLD, non-alcoholic fatty liver disease; NEAT1, nuclear enriched abundant transcript1; NF-κB, nuclear factor κB; NHL, non-Hodgkin lymphoma; NK, natural killer cells; NO, nitric oxide; NOX1, phagocyte-like NADPH oxidase 1; NPC, nasopharyngeal carcinoma; Nrf2, nuclear factor-erythroid related factor 2; OC, oral cancer; OGG1, 8-oxoguanine DNA glycosylase; OPG, osteoprotegerin; OR, odds ratio; P21, cyclin inhibition protein 2l; PAI-1, plasminogen activator inhibitor-1; p-AKT, phosphorylated protein kinase B; P-Akt, phospho-protein kinase B; PARP, poly ADP ribose polymerase; PC-12, pheochromocytoma cells; PCOS, polycystic ovary syndrome; PD-1, programmed death factor 1; PD-L1, programmed death factor ligand 1; PEF, pulsed electric field; PGE2, prostaglandin e2; P-gp, P-glycoprotein; PgR, progesterone receptor; PI3K, phosphatidylinositol 3-kinase; PIKK, phosphatidylinositol 3-kinase-related protein kinase; PIN1, peptidyl-prolyl isomerase NIMA-interacting 1; PKA, protein kinase A; PLCO, prostate, lung, colorectal and ovarian cancers; PMD, mammographic density; PP2A, protein phosphatase 2A; PPIase, peptidyl-prolyl cis-trans isomerase; Ptc, membrane receptors patched; RANK, receptor activator of nuclear factor κB; RARα1, retinoic acid receptor alpha 1; RKO, colorectal cancer cell-line; ROS, reactive oxygen species; RR, relative risk; SAPK, stress-activated protein kinase; SCC, squamous cell carcinoma; SEAHS, Southeastern Arizona Health Study; SFRP1, secreted frizzled-related protein 1; SHH, sonic hedgehog pathway; SHP1, Src homology containing protein tyrosine phosphatase 1; SKY, tyrosine protein kinase; Smad, drosophila mothers against decapentaplegic protein; Smo, membrane receptors smoothened; SN-38, 7-ethyl-10-hydroxycamptothecin; SOX2OT, sex-determining region y-box 2 overlapping transcript; SPHK1, sphingosine kinase 1; STAT3, signal transducers and activators of transcription; SUFU, suppressor of fused; SUM-149, breast cancer cell-line; SW780, bladder cancer cell-line; TGF-β1, transforming growth factor-β; THP-1, human monocytic leukemia cell-line; TIMPs, tissue inhibitor of matrix metalloproteinases; TLR-4, toll-like receptor 4; TME, tumor microenvironment; TMZ, temozolomide; TNBC, triple-negative breast cancer; TNF-α, tumor necrosis factor α; Tollip, toll interaction protein; TPA, 12-O-tetradecanoylphorbol-13-acetate; TRAIL, factor-related apoptosis-inducing ligand; TWIST 1, twist family bHLH transcription factor 1; uPA, urokinase plasminogen activator; VEGF, vascular endothelial growth factor; VEGFR2, vascular endothelial growth factor receptor 2; WAF1, wild-type p53 activated fragment 1; Wnt, wingless-type mice mammary tumor virus integration site family.
Keywords: Camellia sinensis, tea catechins, anticancer, antioxidant, free radicals, synergistic interaction, metastasis, signaling pathway
Citation: Li X-X, Liu C, Dong S-L, Ou C-S, Lu J-L, Ye J-H, Liang Y-R and Zheng X-Q (2022) Anticarcinogenic potentials of tea catechins. Front. Nutr. 9:1060783. doi: 10.3389/fnut.2022.1060783
Received: 03 October 2022; Accepted: 21 November 2022;
Published: 05 December 2022.
Edited by:
Qunfeng Zhang, Chinese Academy of Sciences (CAS), ChinaReviewed by:
Mei-Ya Liu, Tea Research Institute (CAAS), ChinaZhenlei Zhao, Zhejiang Hospital, China
Xinghui Li, Nanjing Agricultural University, China
Copyright © 2022 Li, Liu, Dong, Ou, Lu, Ye, Liang and Zheng. This is an open-access article distributed under the terms of the Creative Commons Attribution License (CC BY). The use, distribution or reproduction in other forums is permitted, provided the original author(s) and the copyright owner(s) are credited and that the original publication in this journal is cited, in accordance with accepted academic practice. No use, distribution or reproduction is permitted which does not comply with these terms.
*Correspondence: Yue-Rong Liang, eXJsaWFuZ0B6anUuZWR1LmNu; Xin-Qiang Zheng, eHF6aGVuZ0B6anUuZWR1LmNu
†These authors have contributed equally to this work