- 1Applied Optics and Photonics Group, School of Engineering and Physical Sciences, Heriot-Watt University, Edinburgh, UK
- 2Department of Physics, Centre for Photonics and Photonic Materials, University of Bath, Bath, UK
The focus of this review is recent work to develop microstructured hollow core fibers for two applications where the flexible delivery of a single mode beam is desired. Also, a brief review of other fiber based solutions is included. High power, short-pulsed lasers are widely used for micro-machining, providing high precision and high quality. However, the lack of truly flexible beam delivery systems limits their application to the processing of relatively small planar components. To address this, hollow-core optical fibers for the 1 μm and green wavelength ranges have been developed. The hollow core overcomes the power delivery limitations of conventional silica fibers arising from non-linear effects and material damage in the solid core. The fibers have been characterized in terms of power handling capability, damage threshold, bend loss and dispersion, and practically demonstrated delivery of high peak power pulses from the nanosecond to the femtosecond regime. Such fibers are ideal candidates for industrial laser machining applications. In laser surgical applications, meanwhile, an Er:YAG laser (2.94 μm) is frequently the laser of choice because the water contained in tissue strongly absorbs this wavelength. If this laser beam is precisely delivered damage to surrounding tissue can be minimized. A common delivery method of surgical lasers, for use in the operating theater, is articulated arms that are bulky, cumbersome and unsuitable for endoscopic procedures. To address this need for flexible mid-IR delivery silica based hollow core fibers have been developed. By minimizing the overlap of the light with glass it is possible to overcome the material absorption limits of silica and achieve low attenuation. Additionally, it is possible to deliver pulse energies suitable for the ablation of both hard and soft tissue even with very small bend radii. The flexibility and small physical size of systems based on these fibers will enable new minimally invasive surgical procedures.
Background
Flexible Delivery for Next Generation Industrial Micro-Machining Lasers
The last few decades have seen industrial lasers replace many conventional tools in diverse areas of manufacturing, enabling increased productivity, quality, and functionality. The introduction of a wide range of laser processes has revolutionized automotive, aerospace and electronics production. The demands from these new applications for finer feature sizes in a wide range of materials has led to continued development of higher performance laser systems. In particular, high beam quality systems are important for precision micro-machining, providing small and intense focused spots (i.e., small tool size) in order to generate small-scale, high quality features [1, 2]. To address this demand high average power laser systems are now commercially available which generate short (nanosecond), and more recently, ultrashort (picosecond) pulses at the high repetition rates and high peak powers ideal for precision micro-machining. In addition the high peak powers allow for precision processing of a much wider variety of materials from glasses to metals at practical processing speeds. The ability of conventional silica fiber optics to deliver the high beam quality, high peak power pulses required for such micro-machining processes has been severely restricted due to limitations imposed by optical damage.
Therefore practical manufacturing using these laser systems either have stacked linear translation stages to manipulate the sample under the laser beam, or employ galvo scanheads (if faster translation speeds are required) to scan the beam over the sample. In general this limits the practical application to planar processes. A flexible delivery system would be ideal for extending the application to processing of more complex non-planar, freeform components as it would enable integration into novel robotic platforms. Additionally, a flexible fiber delivery system would simplify integration of laser systems into any manufacturing process by reducing the requirement for bulk or flying optics, eliminating alignment issues and providing a route for robust multiplexing (where one expensive laser system may be shared between many processing workstations).
Single mode pulse delivery at 1064 nm has been demonstrated in a conventional solid core fiber [1] but with very low peak powers of the order of 250 W. Also, the pulse durations in this work were much longer (0.125 ms) than is ideal for high precision micro-machining. Indeed, conventional single-mode silica fibers have been shown to damage at pulse energies of only ~5 μJ with 532 nm, 8 ns pulses [3], whereas pulse energies of ~1 mJ are required for laser machining at realistic process rates. Alternatives for solid core fiber delivery of Nd:YAG laser light for machining purposes has concentrated on large core or multimode delivery [4–6] to overcome the high intensities that arise in a small single mode. Even though very high energy pulses (10–30 J) can be delivered through a silica fiber (because of larger mode area) without exceeding the laser induced damage threshold (LIDT) the beam quality suffers (M2 ~ 20) and is not suitable for high precision machining [4]. Again, in this instance, the pulse duration is in the millisecond regime, much longer than the desired ns or ps regime required for precision micro-machining. Finally, significant effort has been made to develop large mode area single mode fibers [7]. These employ a range of techniques to preserve the single mode in a large core [7]. Whilst they have been prevalent in new fiber laser system architecture, where the fiber is not manipulated, they exhibit a high degree of bend sensitivity and hence are unsuitable for industrial and medical beam delivery.
Flexible Delivery for Medical (Er:YAG) Lasers
The Er:YAG laser (2.94 μm) is commonly used in medical procedures due to the very high absorption coefficient of water (12000 cm−1 at this wavelength [8]), the main constituent of biological tissue. Consequently this enables precise tissue removal with a small heat penetration depth and high ablation rates. Additionally the high precision and minimal heat-affected zone reduces cell death in surrounding tissue. Laser based surgical procedures have additional benefits in that there is no applied pressure which can reduce pain experienced by the patient (particularly for dental drilling [9]) and the cut dimension is dictated by the focused spot size which generally can be significantly smaller than traditional surgical tools. However, as is shown in Figure 1, bulk silica is strongly absorbing at this wavelength (>50dB/m) [10] which prohibits the use of solid core silica fibers for delivery of light in these laser systems.
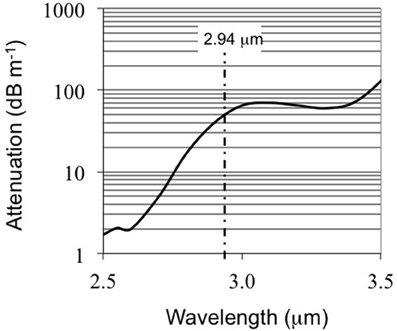
Figure 1. Bulk attenuation for dry silica (Suprasil F300) used for the fabrication of the HC-PCF, from [10].
The most commonly used system for the delivery of Er:YAG laser light in the operating theater is an articulated arm [9]. There exist a number of drawbacks with these systems, such as misalignment issues which in the worst case results in significant beam wander as the arm is manipulated. These systems are also difficult to maintain requiring a dedicated technical expert. More significant is the restriction they impose on the surgeon, as these systems are bulky and cumbersome (in comparison with other surgical tools), and they are unsuitable for endoscopic delivery and use in minimally invasive procedures. It is clear therefore that a lightweight and highly flexible fiber delivery system would alleviate these problems and radically increase the usefulness of Er:YAG lasers in surgery.
The development of flexible and robust fibers for this particular mid-infrared wavelength is particularly challenging from a materials aspect and consequently fibers that have been manufactured to date for such use have significant drawbacks. A variety of solid core fibers have been investigated for Er:YAG delivery such as those based on Chalcogenide glasses [11], GeO2 glasses [12, 13] or Sapphire [13]. Relatively high power delivery has been demonstrated with these systems at fluences useful for surgery but, in order to reduce the energy density in the solid core to a level below the LIDT of the bulk material, larger cores must be used. These large core fibers are multimoded and consequently the energy distribution and density at the fiber output is bend sensitive.
In endoscopy applications, such as endourology, the typical bend diameters required are of the order of 15 cm [14]. This imposes a mechanical limit on the maximum core diameter of the fiber. For example, such bend radii are only possible with solid core sapphire fibers with core diameters below 600 μm which then imposes a damage threshold on the fiber [15] ultimately limiting the maximum deliverable energy. Fluoride glass fibers with an attenuation of ~0.2 dB/m provide a promising alternative, the state-of-the-art delivery systems with these fibers can achieve bend diameters of 20 cm [16] although the output beam profile does change with bending due to the multimode character. However, in order to develop radical new minimally invasive procedures, where access is severely restricted, it would be necessary have bend diameters below 1 cm so the current solid core fiber solutions are not viable.
Hollow Core Fiber Delivery Solutions
It is clear that solid core fibers have not yet provided appropriate solutions for flexible delivery of high peak power industrial lasers or medical Er:YAG systems, and indeed are unlikely to do so in the foreseeable future. Consequently, effort has been focused on circumventing the issues associated with having high intensity radiation in a solid core by implementing hollow core fiber designs.
Since their first practical demonstration in 1999 [17] hollow core photonic crystal fibers (HC-PCF) and specifically those known as hollow core photonic bandgap fibers (HC-PBGF) have been proposed as candidates to provide a solution for flexible delivery of high peak power laser light. These fibers (fabricated from a stack of capillaries with a central “defect” i.e., missing capillaries [17]) can outperform conventional solid core single mode silica fibers. This is because the light is guided in air and so they overcome the limitations due to the low damage threshold and non-linear effects dominant in conventional single-mode solid core silica fibers [18, 19] and can also minimize attenuation imposed by material absorption [20].
The guidance mechanism for the HC-PBGF relies on the confinement of light within a low-index “defect” which is surrounded by a photonic crystal cladding [21]. For a particular wavelength or frequency, bandgaps exist for values of the propagation constant, β, in which one would normally expect propagating modes. These are surrounded at both higher and lower values of β by propagating modes. It is possible for bandgaps to exist where β < k (k being the wavevector of a vacuum,) which allows trapping of light in the “defect” or air core. However, bandgaps only occur for a limited range of k > β which means that HC-PBGFs only guide over a limited wavelength range [21].
The first demonstration of high-energy nanosecond pulses (65 ns) at high repetition rate (15 kHz) delivered from a 1064 nm Nd:YAG micro-machining laser through a HC-PBGF showed an order of magnitude increase in deliverable pulse energy compared to a conventional single mode fiber [22]. This fiber was a so-called 7-cell defect HC-PGBF where seven capillaries are removed from the central region to form an ~8 μm hollow core (Figure 2A). This design was further improved by using a larger core design (a 19 cell defect to form an ~13 μm hollow core as shown in Figure 2B) and practical micro-machining was demonstrated for the first time with fiber-delivered light using 60 ns pulses of the order of 1 mJ at repetition rates of up to 100 kHz [23]. The delivered beam is approximately Gaussian although the spatial beam profile does show some additional structure which arises due to the hexagonal form of the cladding structure [24]. Nevertheless, the high beam quality of the laser light is preserved at the workpiece, even whilst the fiber is tightly bent, ideal for micro-machining. Although these fibers were limited by a relatively small core size and a less than ideal overlap of the light with the silica cladding this work nevertheless clearly demonstrated the advantages of such technology for industrial beam delivery.
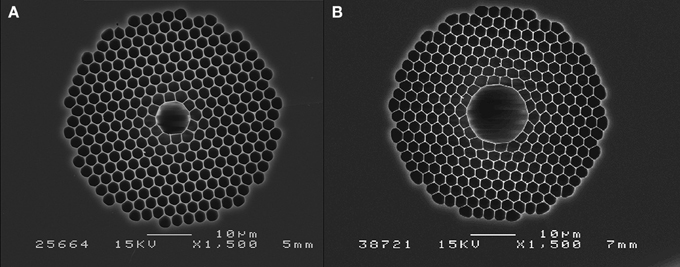
Figure 2. HC-PBGF designed for guidance at 1064 nm with (A) an 8 μm core formed with a seven cell defect and [22] (B) a 13 μm core formed with a 19 cell defect [23].
The ability of the HC-PBGF design to overcome the material absorption limitations imposed by the silica has also been demonstrated with the first report in 2005 of single mode guidance in a silica HC-PBGF having a minimum attenuation of 2.6 dB/m (at 3.14 μm) [25]. This has been improved on recently with a 19-cell silica HC-PCF having a minimum attenuation of 0.13 dB/m in the 3.1–3.7 μm range [26]. A similar HC-PCF design to those shown in Figure 2 was demonstrated for delivery of an Er:YAG laser light for surgical applications [20]. The fiber attenuation in this instance was 1.2 dB/m (compared to 50 dB/m for silica at this wavelength) allowing pulse energies of up to 14 mJ (225 μs) to be delivered. Due to the small core size this translates to a fluence of 66 J/cm2 twice the threshold required for ablation of dental enamel, the human tissue that is most difficult to ablate. The fiber was able to deliver such pulses whilst being bent to radii of approximately 2.5 mm and is hence a perfect candidate for minimally invasive surgical procedures. The pulse energy delivered was ultimately limited by poor coupling between the laser and the fiber, the laser having a very low beam quality with a donut beam shape. Calculations based on damage tests performed on the silica cladding predict that, given a higher beam quality laser source and better coupling, pulses in the order of 500 mJ could be delivered [20].
In 2002 a different type of hollow core microstructure fiber was proposed, the Kagome-lattice HC-PCF [30] with the guidance described as an Inhibited Coupling mechanism [31]. However, the most notable advances with this type of fiber have been made more recently with the development of a hypocycloid-type Kagome-lattice fiber where the core wall was altered to a “negative curvature” geometry (from a circular or polygonal structure) to reduce coupling into the cladding [32]. These fibers have a broader transmission window and have been demonstrated to deliver ns pulses with energies in the range of 10 mJ [33]. Also, delivery of 10.5 ps pulses with an energy of approximately 97 μJ and an average power of 5 W, corresponding to a peak power of 8 mW through 10 cm long Kagome fiber [34] has been shown.
Another area of interest and importance in industry for the flexible delivery of high peak power laser pulses is in spark ignition [35]. Laser flame initiation has been shown to have potential advantages for ignition in applications such as internal reciprocating engines, rockets and in gas engines used for power generation [35]. A flexible, fiber delivered system, where a single high power laser system is delivered to the ignition points in such applications offers significant advantages over open path optics such a reliability, robustness and relative simplicity which would enable a lower cost system to be developed. Although a number of fibers are being investigated for this application it is hollow core microstructured fibers, in particular the Kagome-Lattice HC-PCF, that have shown particularly promising results. Two designs of Kagome-lattice HC-PCF (a single cell defect and a 7-cell defect) were demonstrated to deliver 9 ns pulses of up to 10 mJ before breakdown [33]. The high beam quality of the delivered pulses allowed tight focussing causing air breakdown and hence spark ignition of butane was demonstrated for the first time in a HC-PCF [33].
For the delivery of medical lasers there also exists a range of hollow waveguide (HWG) fibers that confine the optical radiation by different mechanisms, e.g., total internal reflection; Bragg reflection; and internal reflection at a dielectric coated metallic interface in the case of leaky tube waveguides, which guide the light in the infrared wavelength range up to 20 μm [36–38]. However, most types of HWG support many guided modes which, as with the solid core multimode fibers described earlier, leads to high bend losses, inter-modal dispersion and interference which causes changes to the output beam profile as the fiber is bent [39]. For example, the attenuation of a HWG with a 750 μm core has been reported as 1.9 dB/m (90° bend) and 2.9 dB/m (180° bend) when the bend diameter was 30 cm [40].
More recent developments of HWG's have concentrated on the fabrication of smaller core diameters to increase their flexibility. Also, alternative techniques for the fabrication of longer fibers have been investigated to overcome the length limitation (2–3 m) associated with the usual fabrication process (where a reflective layer is deposited inside a glass capillary via vapor deposition [41]). Matsuura et al. [41] demonstrated fabrication of a Pyrex-glass hollow fiber with an inner diameter of 280 μm using the glass-drawing technique in which the Ag and dielectric layer are applied on the outside of the fiber, which enables longer fibers (>20 m). However, at around 3 μm the losses for a 15 cm length piece of fabricated fiber were in the order of 5 to 10 dB, no energy handling capability was presented and the fibers were mechanically fragile. Matsuura et al. [42] also reported a HWG with an inner diameter of 250 μm and a transmission of 77% for 10 cm long fiber piece, however the output beam profile changed with the input power and bending. Although restricted to the delivery of light in the 10 μm wavelength region (e.g., CO2 laser delivery at 10.6 μm) the most promising of the HWG fibers is the so-called Omniguide fiber [43, 44]. These are now being implemented into commercial medical devices. However, with core diameters of a few hundred microns and overall fiber diameters in the order of 1 mm they still have limitations with regards to bend diameter and flexibility. Additionally, due to material limitations, practical Omniguide fibers for Er:YAG laser delivery at 2.94 μm have not yet been realized [45].
Hollow Core Negative Curvature Fibers (NCF) for Industrial and Medical Applications
This section provides a detailed review of the recent progress in the development of novel silica Negative Curvature Fibers (NCF) for industrial and medical beam delivery applications. The silica hypocycloid-type Kagome-lattice and silica HC-PBGF have demonstrated favorable properties for high peak power delivery of laser light and transmission beyond the normal absorption limits of the bulk material as discussed above. However, one feature of both these fiber designs is that the complexity of the cladding is high. The importance of the negative curvature geometry to enhance the guidance was identified with the hypocycloid-type Kagome-lattice type fiber however, and a new fiber design was proposed [46, 47] that enhances this curvature with a much simpler structure, the Negative Curvature Fiber. In 2011 Pryamikov reported a hollow silica fiber with a negative curvature core wall formed by a single ring of capillaries (compared to the complex stacked layers found in Kagome fibers or HC-PBGF) and demonstrated transmission bands extending beyond 3.5 μm [46]. A similar NCF design was then fabricated using chalcogenide glass to demonstrate a negative-curvature fiber which extended the transmission region to 10.6 μm for CO2 laser transmission [48]. The following sections review progress with the NCF design (Figure 3A) first reported by Yu et al in 2012 [47].
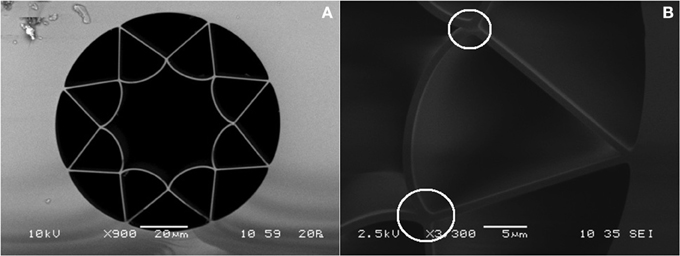
Figure 3. (A) SEM image of the NCF with a 43 μm core designed for the 1 μm regime. (B) SEM picture of the capillary forming the negative curvature of the fiber core wall and the cladding nodes (white circles) [50].
Fiber Design
The NCF was fabricated using the stack and draw technique [47] where eight identical circular silica capillaries were used to fabricate a preform with a jacketing tube, which is subsequently drawn down to produce the final fiber dimensions. The critical fiber parameters such as the core wall thickness, core diameter and radius of the negative curvature of the core wall are controlled by adjusting the draw parameters [47].
The NCF guidance mechanism is described as an Anti-Resonant Reflecting Optical Waveguiding (ARROW) phenomenon where the core wall structure acts as a Fabry-Perot resonant cavity [49]. The wavelengths that are not in resonance with the core wall are reflected back into the core and propagate with low loss as a result of destructive interference in the Fabry-Perot resonator. The frequencies resonant with the wall cannot be confined and therefore leak away to the cladding region where they are highly attenuated. Consequently the thickness of the core wall (or capillaries) determines the wavelength range for guidance. One notable feature of this NCF geometry is the contact points (or cladding nodes) between adjacent capillaries that can act as independent, but highly lossy, waveguides (Figure 3B). However, the negative curvature of the core wall acts to physically distance the guided core mode from these potential lossy modes and therefore coupling to them is significantly reduced [50].
Fiber Attenuation and Bending Loss
Fibers based on the above design were fabricated and successfully demonstrated for transmission at 2.94 μm [51, 52], 1064 and 1030 nm [50] and 532 and 515 nm [53].
To measure the attenuation of the fiber at 2.94 μm a cut back measurement was carried out, using a tunable laser as the optical source [51]. The total attenuation in these NCF fibers is a combination of confinement and absorptive losses and losses induced by bending. Consequently a practical total fiber loss was measured using a cutback technique with a coiled fiber (0.25 m radius of curvature) and found to be 0.183 ± 0.05 dB/m which is more than acceptable for medical laser delivery where it is likely that no more than around 1 or 2 m of fiber would be required. The additional loss induced by decreasing the bend diameter of the fiber was measured using a 180° bend with radii from 25 cm down to 2.5 cm for 1.23 m long fiber. It was shown that there were no significant additional loses (see Figure 4) if the bend radius is >15 cm which is sufficient for many applications [51]. This additional loss is likely to arise from core mode(s) coupling into cladding modes that are strongly absorbed. It should be highlighted that further optimization of the NCF design has resulted in fiber for the green spectral regime that has much reduced bend loss (discussed below), and it is expected that a similar performance could be obtained at 2.94 μm with a similarly optimized fiber hence producing a fiber with no bend sensitivity at bend radii in the order of a few cm which would be ideal for endoscopic application [14]. Additionally, it should also be stressed that despite this additional bend loss even at very small bend diameters (<10 cm) the NCF was capable of delivering pulses with a fluence far in excess of that required for hard tissue ablation (see below).
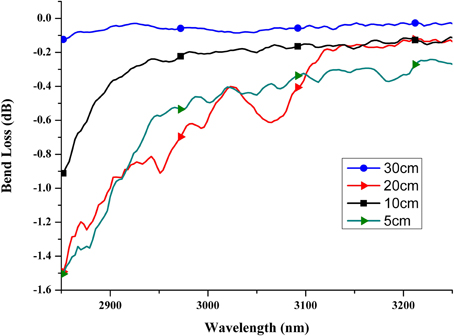
Figure 4. Additional losses due to a single 180° bend in a 1.23 m long NCF piece [51].
The attenuation of the NCF fabricated for guidance at 1030 nm and 1064 nm showed significant bend sensitivity [50], again due to the coupling into higher order modes. Using a Spectra-Physics Q-switched Nd:YVO4 nanosecond laser (60 ns pulses at 1064 nm) and a Trumpf TruMicro picosecond laser (6 ps pulses at 1030 nm) the fiber attenuation was measured independently of bend loss for a 1 m long, straight fiber, and was found to be 0.23 and 0.16 dB/m for 1030 and 1064 nm, respectively. The attenuation spectrum of a coiled fiber was also measured over the spectral range from 1000 to 1400 nm. The low-loss region covers more than 300 nm (1010–1330 nm) of the IR spectral bandwidth. This spectrum is shown in Figure 5. Nevertheless, despite the high bend sensitivity with this particular fiber the power delivery was excellent (see below) and suitable for practical micro-machining applications. Optimized fibers similar to those demonstrated in the green spectral regime are expected to have very low bend sensitivity and hence better performance for real beam delivery applications.
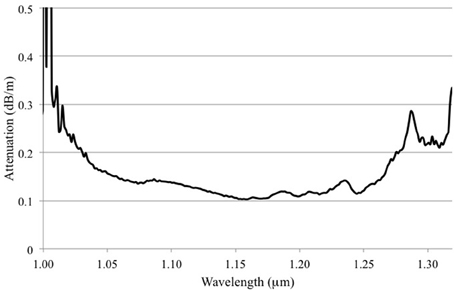
Figure 5. Attenuation spectrum of the NCF for the 1 μm wavelength regime [50].
The fibers with the lowest bend sensitivity fabricated to date for the green spectral region have an attenuation of 0.23 dB/m at 515 nm and 0.21 dB/m at 532 nm. These measurements were made with the frequency-doubled output of the nanosecond laser (~55 ns at 532 nm) and the picosecond laser (6 ps at 515 nm) [53]. By optimizing the fiber fabrication process it was possible to have a highly confined single mode with minimized coupling to higher ordered modes (which otherwise introduces significant bend sensitivity). Consequently, the single mode guided in this fiber is stable and independent of bending or perturbations of the fiber [53].
Damage Limitations and Pulse Delivery
The fiber damage thresholds and ultimate power delivery capabilities of each of the NCFs discussed above was investigated.
At 2.94 μm a microsecond pulsed Er:YAG laser was used (225 μs) as this is the most relevant and commonly used type for current medical procedures [51]. The maximum output energy delivered through the NCF was 195 ± 1 mJ for a 33 cm length of fiber and 54 ± 4 mJ for a 9.88 m length with the fiber bent to a diameter of 50 cm over a length of 80 cm. The output beam was single mode-like with an approximately Gaussian profile and was stable when the fiber was bent (Figure 6). Based on a core diameter of 94 μm these energies equate to delivered energy densities of 2300 J/cm2 for the 33 cm length and 764 J/cm2 for the 9.88 m clearly exceeding the requirement for ablation of human dental enamel (35 J/cm2). Additionally, neither the input or output facets of the NCF were damaged during the experiments when using the Er:YAG laser at maximum power. Consequently, the ultimate energy deliverable could not be ascertained but was instead limited by the available Er:YAG system.
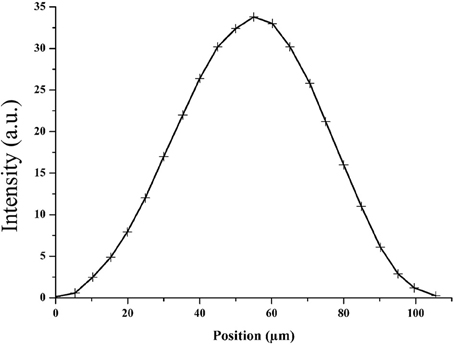
Figure 6. Fiber output beam profile (2.94 μm) for the NCF bent with a diameter of ~50 cm over a length of 80 cm [51].
For the ns regime at 1064 nm the Spectra Physics Q-switched laser (M2 < 1.3) was used [50]. This provided 60 ns pulses with a pulse energy >1 mJ and an average power of 18.2 W at a repetition rate of 15 kHz. Pulse delivery tests were conducted with both a 0.7 m straight fiber and a 10.5 m fiber coiled to a diameter of 60 cm. Both fibers proved capable of handling the maximum pulse energy from the laser without damage, with the 0.7 m straight fiber delivering pulses of 1.1 mJ at an average power of 16.3 W and a peak power of 18.3 kW [50]. Due to the fiber loss the delivered pulse for the 10.5 m coiled fiber falls to 0.8 mJ but is still highly useful for micro-machining applications. The damage threshold could not be reached with the 60 ns system however using a shorter pulsed laser system (9 ns at 1064 nm) the fiber failed at 3.2 mJ. Assuming the commonly accepted scaling of damage threshold with pulse duration of τ0.5 [50] this translates to an 8 mJ pulse at 60 ns which is 16 times greater than reported with the HC-PCF design [22].
Picosecond delivery was tested using the Trumpf picosecond laser (M2 > 1.3). Testing was carried out using 6 ps pulses, 400 kHz repetition rate, pulse energy of up to 116 μJ at 1030 nm and average and peak powers of 46.3 W and 19.3 mW, respectively [50]. 92 μJ pulses (average power of 36.7 W and peak power of 15.3 mW) were delivered through a 1 m straight length of fiber. These delivered powers are higher than those currently reported for the hypocycloid Kagome fiber [34]. Delivery was also carried out using an 8 m length NCF coiled to a diameter of 23 cm. In this instance, due to additional bend loss, the transmitted energy was 49 μJ (average power of 19.6 W and peak power of 5.6 mW).
Unfortunately, the fiber output exhibited few-moded behavior for both ns delivery at 1064 nm and ps delivery at 1030 nm (Figure 7). It was possible to achieve a delivered pulse with relatively high beam quality of M2 = 1.5 by coupling the majority of the power into the fundamental mode. However, due to the multimode behavior of the fiber a more typical delivered beam had an M2 of around 3 and inter-modal interference led to a fluctuating spatial beam profile as the fiber was manipulated.
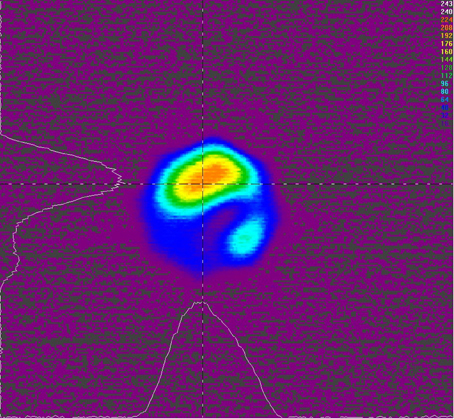
Figure 7. False color image of delivered beam profile for the NCF at 1030 nm (6 ps pulse) [50].
An intensity autocorrelation measurement was made, demonstrating that there is no measurable dispersion of a 6 ps pulse delivered through the 1 m straight fiber in comparison with the original laser pulse [50]. However, the pulse did exhibit broadening (from 6 to 8.7 ps) after propagation through the longer (8 m) coiled fiber [50]. There was no noticeable distortion of the pulse shape and no degradation of the optical spectra of NCF delivered pulse compared with the laser output [50].
The NCF fabricated for the green spectral range was assessed using the frequency doubled output of the laser systems described above i.e., 532 nm for ns delivery and 515 nm for ps [53]. The ns laser provided 55 ns pulses at a 15 kHz rep. rate with pulse energy up to 0.65 mJ (average power 9.8 W and a peak power of 12 kW). No damage occurred, even when the full output power of the laser was used. Pulses of 0.38 mJ (peak power 6.9 kW) were delivered through a 9.5 m length. Pulse from the Trumpf TruMicro (6 ps pulses, 400 kHz, maximum pulse energy 62 μJ (average power 25 W and peak power of 10.3 MW) were coupled into 9.5 m of NCF with a coupling efficiency of 86% which allowed delivery of pulses with energies of 18 μJ and peak power of 3 mW (7.3 W average). Damage to the fiber input end-facet occurred at pulse energies of 50 μJ corresponding to a peak power of 8.3 mW and peak power density of 10.6 TWcm−2. Perhaps most importantly, the fiber fabricated for this wavelength regime provided a highly stable output profile in comparison to the NCF discussed above for guidance at 1 μm. This shows that the delivered beam is truly single mode, making it ideal for practical applications (Figure 8). It is expected that a similarly improved fiber for delivery of the 1 μm wavelength is possible.
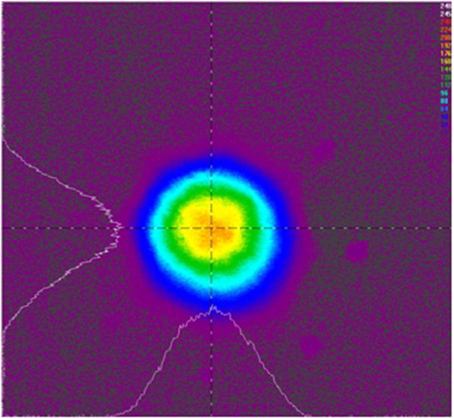
Figure 8. False color image of spatial beam profile delivered by NCF at 515 nm (6 ps pulse) [53].
Practical Demonstration of NCF for Industrial and Medical Applications
Micro-Machining Applications
It is clear that the NCFs show great promise for practical industrial application in terms of power handling capability. In order to demonstrate the suitability of the NCF for micro-machining applications a number of trials have been performed; namely through cutting of aluminum sheet and marking of titanium with the ns laser and milling of fused silica with the ps laser [50, 53]. In these experiments the fiber was used to deliver the beam from the laser to the input of a galvanometer scan head that directs and scans the beam onto the workpiece. The fibers were coiled to simulate a realistic situation. A more detailed description of the experimental arrangement can be found [50, 53].
At 1064 nm, NCF-delivered 0.8 mJ, 60 ns pulses were used at a repetition rate of 15 kHz laser to through-cut 0.3 mm thick aluminum sheet. The delivered pulse energy was sufficient to perform precise cutting of relatively small shapes, less than 1 mm by 1 mm with a cutting speed of 1 mm/s, see Figure 9. Despite the multi-mode nature of the fiber and bending-induced profile changes, high quality shapes were generated.
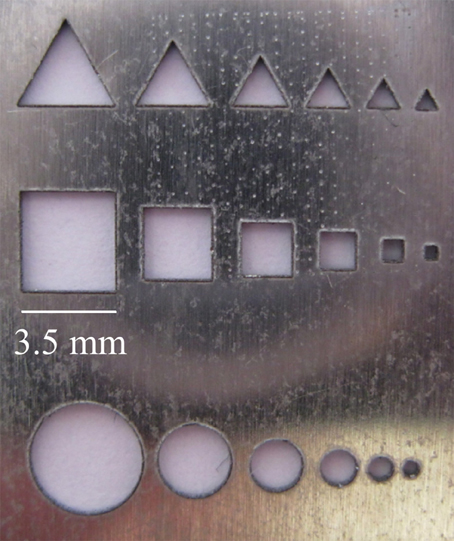
Figure 9. Example of through-cutting of 0.3 mm thick aluminum sheet using 60 ns pulses (1064 nm) delivered via the NCF [50].
Using the same laser parameters, marking of titanium was also demonstrated (Figure 10), but with a significantly higher surface scanning speed of 100 mm/s. Sharp definition of the desired image was achieved on the sample with no observable damage to the surrounding material, typical for a well optimized micro-marking process. This demonstrates that the NCF did not degrade the beam to a level that affects the laser machining process.
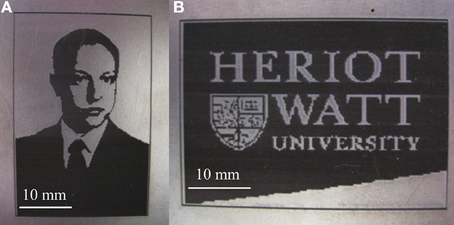
Figure 10. Examples of marking on titanium (A,B) using 60 ns pulses (1064 nm) delivered via the NCF [50].
Picosecond pulsed lasers have been shown to be ideally suited for the machining of materials that are traditionally difficult to process such as ceramics and glass [54, 55]. The Trumpf ps laser (6 ps, 1030 nm) was therefore used to machine silica glass (Figure 11) using a similar experimental arrangement to the ns processing described above, incorporating a coiled length of NCF [50]. The pulse energy was 52 μJ at a repetition rate of 400 kHz with a scanning speed of 100 mm/s. The key feature in these experiments was that no cracking within the glass was observed after processing (Figure 11). This was the first demonstration of crack-free laser machining of glass with fiber delivered pulses in the 1 μm wavelength region. Due to the low damage threshold and non-linear effects this is not possible with large mode area solid core fibers or indeed conventional hollow-core fibers. Although longer ns pulses can be delivered using large mode area solid core fibers the thermal effects would result in cracking of the silica. This further highlights the usefulness of NCFs for practical micro-machining applications as they are capable of delivering the pulses generated by state-of-art micro-machining lasers, without degrading either the beam profile or pulse length.
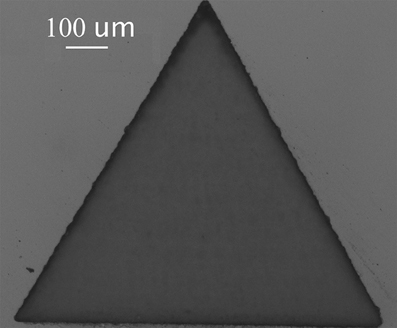
Figure 11. Micro-milled pattern in fused silica using 6 ps pulses (1030 nm) delivered pulses via the NCF [50].
Tissue Ablation
For practical application in surgery the hollow core NCF would require encapsulation (as would all hollow core fibers used in such an application). This is to avoid ingress of debris and liquids (e.g., blood or tissue fragments) into the core. The approach adopted was to use a solid sapphire endtip mounted onto the fiber output end [51] (Figure 12).
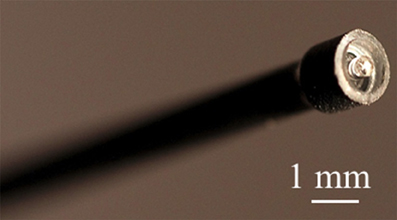
Figure 12. Sapphire endtip mounted onto the NCF designed for 2.94 μm guidance [51].
The consequence of having a sapphire window is that the energy delivered must be restricted in order to avoid damage to the endtip. Nevertheless, the energy delivered at 2.94 μm via a 2 m long NCF was 30 mJ (225 μs pulse) which translates to an energy density of >500 J/cm2 directly at the output facet of the endtip. This energy density was far in excess of that required for ablation of soft and hard biological tissue (Table 1) and importantly is well within the maximum energy demonstrated with the NCF at this wavelength.
Laser processing of porcine bone (see Figure 13) and muscle was demonstrated using NCF delivered laser pulses [51]. To experimentally simulate the envisaged practical application, the end-tipped fiber was tested in contact mode, with increasing standoff distances and in aqueous conditions (see Figure 13). One final practical consideration is that for sterilization purposes the NCF might be required to undergo an autoclaving process. A sealed NCF was treated in a water atmosphere at 121°C and 15 psi for 15 min. After repeated autoclaving the treatment no degradation of the NCF was observed. These experiments provide a convincing demonstration that NCFs are a viable solution for the flexible delivery of Er:YAG lasers for medical applications and it is now expected that a number of minimally invasive procedures will be developed based on this technology.
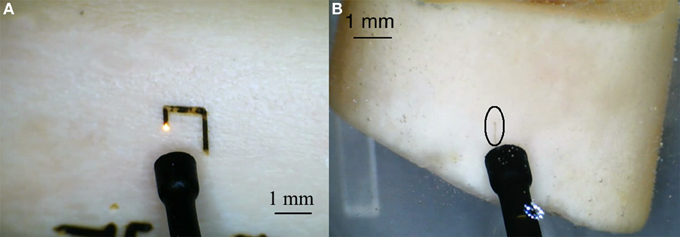
Figure 13. Processing of bone with NCF delivered Er:YAG laser pulse4s (2.94 μm 225 μs) (A) in air and (B) under water (the black circle highlights the cut region) [51].
Conclusions
There has been significant effort over the last decade to address the challenges of integrating next generation laser systems into manufacturing processes and to expand the use of lasers in medicine. The fundamental requirement of having a truly flexible fiber delivery system has, until now, been a significant restriction to the development of these technologies. However, as described in this review, the emergence of novel hollow core fiber designs, which both extend the power handing capability and the operating wavelength regime of fibers by reducing the interaction of the light with the glass material, have significantly advanced this field.
Of these hollow core fibers it is clear that NCF provide very promising solutions. They have been practically demonstrated to flexibly deliver both ps and ns pulses from commercial micro-machining lasers whilst preserving the beam quality and the pulse parameters necessary for practical applications. Low loss guidance at 1063, 1030, and 532 nm for pulse lengths of 60 ns and 6 ps at repetition rates optimized for micro-machining has been successfully shown and examples of processed materials show no reduction in quality due to the delivery fiber.
The NCF has also been proven to be a realistic delivery system for the Er:YAG (2.94 μm) laser. The NCF design allows an all silica fiber to operate well above the transmission cut-off for conventional silica fibers (around 2 μm) with low guidance demonstrated up to around 4.5 μm. Using such a fiber to deliver 225 μs pulses at 2.94 μm practical ablation of both soft and hard tissue has been demonstrated. Relatively low bend sensitivity was reported and pulses suitable for medical cutting of tissue is possible at fiber bend diameters that are consistent with integration into endoscopic systems. A practical solution for end-capping NCF at these mid-infrared wavelengths has also been proposed utilizing a sapphire window. This clearly demonstrates that the NCF has significant potential for exploitation in new minimally invasive medical procedures.
It is expected that the next few years will see an emergence of NCF in practical systems in both industrial and medical environments and it is predicted that this will lead to an enhancement of use of lasers systems in these fields.
Conflict of Interest Statement
The authors declare that the research was conducted in the absence of any commercial or financial relationships that could be construed as a potential conflict of interest.
Acknowledgments
This work is funded by the UK Engineering and Physical Sciences Research Council under grants EP/I01246X/1 and EP/I011315/1. Dr. W. Belardi is now at the Optoelectronics Research Centre, University of Southampton, Southampton, UK and Dr. A. Urich is now at Karl Storz Gmbh & Co. KG.
References
1. Hand DP, Jones JDC. Single-mode delivery of Nd:YAG light for precision machining applications. Appl Opt. (1998) 37:1602–6. doi: 10.1364/AO.37.001602
PubMed Abstract | Full Text | CrossRef Full Text | Google Scholar
2. Meijer J. Laser beam machining (LBM), state of the art and new opportunities. J. Mater Process Technol. (2004) 149:2–17. doi: 10.1016/j.jmatprotec.2004.02.003
3. Stephens TJ. Fibre-Optic Delivery of High Peak Power Laser Pulses for Flow Measu.rement. PhD Thesis, Heriot-Watt University, UK (2003).
4. Kuhn A, Blewett IJ, Hand DP, French, P, Richmond M, Jones JDC. Optical fibre beam delivery of high-energy laser pulses: beam quality preservation and fibre end-preparation. Opt Lasers Eng. (2000) 34:273–88. doi: 10.1016/S0143-8166(00)00082-8
5. Kuhn A, French P, Hand DP, Blewett IJ, Richmond M, Jones JDC. Preparation of fiber optics for the delivery of high-energy high-beam-quality Nd:YAG laser pulses. Appl Opt. (2000) 39:6136–43. doi: 10.1364/AO.39.006136
PubMed Abstract | Full Text | CrossRef Full Text | Google Scholar
6. Su D, Boechat AAB, Jones JDC. Optimum beam launching conditions for graded index optical fibres: theory and practice. IEE Proc-J. (1993) 140:221–6. doi: 10.1049/ip-j.1993.0035
7. Limpert J, Stutzki F, Jansen F, Otto H-J, Eidam T, Jauregui1, C, et al. Yb-doped large-pitch fibres: effective single-mode operation based on higher-order mode delocalisation. Light Sci Appl. (2012). 1:e8. doi: 10.1038/lsa.2012.8
8. Robertson CW, Williams D. Lambert absorption coefficients of water in the infrared. J Opt Soc Am. (1971) 61:1316–20. doi: 10.1364/JOSA.61.001316
PubMed Abstract | Full Text | CrossRef Full Text | Google Scholar
9. Stubinger S, von Rechenberg, VB, Zeilhofer HF, Sader R, Landes C. Er : YAG laser osteotomy for removal of impacted teeth: clinical comparison of two techniques. Lasers Surg. Med. (2007) 39:583–8. doi: 10.1002/lsm.20528
PubMed Abstract | Full Text | CrossRef Full Text | Google Scholar
10. Humbach O, Fabian H, Grzesik U, Haken U, Heitmann W. Analysis of OH absorption bands in synthetic silica. J Non-Cryst Solids (1996) 203:19–26. doi: 10.1016/0022-3093(96)00329-8
11. Sanghera JS, Shaw JS, Aggarwal ID. Applications of chalcogenide glass optical fibers. C R Chim. (2002) 5:873–83. doi: 10.1016/S1631-0748(02)01450-9
12. Scott NJ, Barton NJ, Casperson AL, Tchapyjnikov A, Levin K, Tran D, et al. Mid-IR germanium oxide fibers for contact erbium laser tissue ablation in endoscopic surgery. IEEE J Sel Top Quantum Electron. (2007) 13:1709–14. doi: 10.1109/JSTQE.2007.910557
PubMed Abstract | Full Text | CrossRef Full Text | Google Scholar
13. Fried NM, Yang YB, Chaney CA, Fried D. Transmission of Q-switched erbium: YSGG (lambda=2.79 mu m) and erbium: YAG (lambda=2.94 mu m) laser radiation through germanium oxide and sapphire optical fibres at high pulse energies. Lasers Med Sci. (2004) 19:155–60. doi: 10.1007/s10103-004-0316-8
PubMed Abstract | Full Text | CrossRef Full Text | Google Scholar
14. Huang Z, Fu F, Zhong Z, Zhang L, Xu R, Zhao, X. Flexible ureteroscopy and laser lithotripsy for bilateral multiple intrarenal stones: is this a valuable choice? Urology (2012) 80:800–4. doi: 10.1016/j.urology.2012.05.013
PubMed Abstract | Full Text | CrossRef Full Text | Google Scholar
15. Raif J, Vardi M, Nahlieli O, Gannot I. An Er : YAG laser endoscopic fiber delivery system for lithotripsy of salivary stones. Lasers Surg Med. (2006) 38:580–7. doi: 10.1002/lsm.20344
PubMed Abstract | Full Text | CrossRef Full Text | Google Scholar
16. Kotsifaki DG, Serafetinides AA. Mid-infrared radiation transmission through fluoride glass multimode optical fibers. Opt Laser Technol. (2011) 43:1448–52. doi: 10.1016/j.optlastec.2011.04.017
17. Cregan RF, Mangan BJ, Knight JC, Birks TA, Russell PS, Roberts PJ, et al. Single-mode photonic bandgap guidance of light in air. Science (1999) 285:1537–9. doi: 10.1126/science.285.5433.1537
PubMed Abstract | Full Text | CrossRef Full Text | Google Scholar
19. Issa NA, Argyros A, van Eijkelenborg A, Zagari J. Identifying hollow waveguide guidance in air-cored microstructured optical fibres. Opt Express (2003) 11:996–1001. doi: 10.1364/OE.11.000996
PubMed Abstract | Full Text | CrossRef Full Text | Google Scholar
20. Urich A, Maier RRJ, Mangan BJ, Renshaw S, Knight JC, Hand, DP, et al. Delivery of high energy Er:YAG pulsed laser light at 2.94 μm through a silica hollow core photonic crystal fiber. Opt Express (2012) 20:6677–84. doi: 10.1364/OE.20.006677
PubMed Abstract | Full Text | CrossRef Full Text | Google Scholar
21. Bouwmans G, Luan F, Knight JC, Russell P St J, Farr L, Mangan B, et al. Properties of a hollow-core photonic bandgap fiber at 850 nm wavelength. Opt Express (2003) 11:1613–20. doi: 10.1364/OE.11.001613
PubMed Abstract | Full Text | CrossRef Full Text | Google Scholar
22. Shephard JD, Jones JDC, Hand DP, Bouwmans G, Knight JC, Russell P St J, et al. High energy nanosecond laser pulses delivered single-mode through hollow-core PBG fibers. Opt Express (2004) 12:717–23. doi: 10.1364/OPEX.12.000717
PubMed Abstract | Full Text | CrossRef Full Text | Google Scholar
23. Shephard JD, Couny F, Russell P St J, Jones JDC, Knight JC, Hand DP. Improved hollow-core photonic crystal fiber design for delivery of nanosecond pulses in laser micromachining applications. Appl Opt. (2005) 44:4582–8. doi: 10.1364/AO.44.004582
PubMed Abstract | Full Text | CrossRef Full Text | Google Scholar
24. Shephard JD, Roberts PJR, Jones JDC, Knight JK, Hand DP. Measuring the beam quality of hollow core photonic crystal fibres. J Lightwave Technol. (2006) 24:3761–9. doi: 10.1109/JLT.2006.881845
25. Shephard JD, MacPherson WN, Maier RRJ, Jones JDC, Hand DP, Mohebbi M, et al. Single-mode mid-IR guidance in a hollow-core photonic crystal fiber. Opt Express (2005) 13:7139–44. doi: 10.1364/OPEX.13.007139
PubMed Abstract | Full Text | CrossRef Full Text | Google Scholar
26. Wheeler NV, Heidt AM, Baddela NK, Numkam Fokoua E, Hayes JR, Sandoghchi SR et al. Low-loss and low-bend-sensitivity mid-infrared guidance in a hollow-core–photonic-bandgap fiber. Opt Lett. (2104) 39:295–8. doi: 10.1364/OL.39.000295
PubMed Abstract | Full Text | CrossRef Full Text | Google Scholar
27. Pierce MC, Dickinson MR, Devlin H. Selective photothermal ablation of tissue with a fibre delivered Er : YAG laser, In: Proceedings of Laser-Tissue Interaction X: Photochemical, Photothermal, and Photomechanical. San Jose, CA (1999).
28. Hohenleutner U, Hohenleutner S, Baumler W, Landthaler M. Fast and effective skin ablation with an Er:YAG laser: determination of ablation rates and thermal damage zones. Lasers Surg Med. (1997) 20:242–7. doi: 10.1002/(SICI)1096-9101(1997)20:3<242::AID-LSM2>3.0.CO;2-Q
PubMed Abstract | Full Text | CrossRef Full Text | Google Scholar
29. Nishimoto Y, Otsukii M, Yamauti M, Eguchi T, Sato Y, Foxton RM, et al. Effect of pulse duration of Er : YAG laser on dentin ablation. Dent Mater J. (2008) 27:433–9. doi: 10.4012/dmj.27.433
PubMed Abstract | Full Text | CrossRef Full Text | Google Scholar
30. Benabid F, Knight JC, Antonopoulos G, Russell PSJ. Stimulated Raman scattering in hydrogen-filled hollow-core photonic crystal fiber. Science (2002) 298:399. doi: 10.1126/science.1076408
PubMed Abstract | Full Text | CrossRef Full Text | Google Scholar
31. Couny F, Benabid F, Roberts PJ, Light PS, Raymer MG. Generation and photonic guidance of multi-octave optical-frequency combs. Science (2007) 318:1118. doi: 10.1126/science.1149091
PubMed Abstract | Full Text | CrossRef Full Text | Google Scholar
32. Wang YY, Wheeler NV, Couny F, Roberts PJ, Benabid F. Low loss broadband transmission in hypocycloid-core Kagome hollow-core photonic crystal fiber. Opt Lett. (2001) 36:669–71. doi: 10.1364/OL.36.000669
PubMed Abstract | Full Text | CrossRef Full Text | Google Scholar
33. Beaudou B, Gerome F, Wang YY, Alharbri M, Bradley TD, Humbert G, et al. Milijoule laser pulse delivery for spark ignition through kagome hollow-core fiber. Opt Lett. (2012) 37:1430–2. doi: 10.1364/OL.37.001430
PubMed Abstract | Full Text | CrossRef Full Text | Google Scholar
34. Emaury F, DutinCF, Saraceno CJ, Trant M, Heckl OH, Wang YY, et al. Beam delivery and pulse compression to sub-50 fs of a modelocked thin-disk laser in a gas-filled Kagome-type HC-PCF fiber. Opt Express (2013) 21:4986–4. doi: 10.1364/OE.21.004986
35. Yalin AP. High power fiber delivery for laser ignition applications. Opt Express (2013) 21:A1102–12. doi: 10.1364/OE.21.0A1102
PubMed Abstract | Full Text | CrossRef Full Text | Google Scholar
36. Hongo A, Miyagi M, Kato Y, Suzumura M, Kubota S, Wang Y, et al. Fabrication of dielectric-coated silver hollow glass waveguides for the infrared by liquid-flow coating method, In: Proceedings of SPIE 2677 Biomedical Fiber Optics. San Jose, CA (1996). p. 55–63. doi: 10.1117/12.237566
37. Harrington JA. A review of IR transmitting, hollow waveguides. Fiber Integrated Opt. (2000) 19:211–27. doi: 10.1080/01468030050058794
38. Bowden BF, Harrington JA. Fabrication and characterization of chalcogenide glass for hollow Bragg fibers. Appl Opt. (2009) 48:3050–4. doi: 10.1364/AO.48.003050
PubMed Abstract | Full Text | CrossRef Full Text | Google Scholar
39. Parry JP, Stephens TJ, Shephard JD, Jones JDC, Hand DP. Analysis of optical damage mechanisms in hollow-core waveguides delivering nanosecond pulses from a Q-switched Nd : YAG laser. Appl Opt. (2006) 45:9160–7. doi: 10.1364/AO.45.009160
PubMed Abstract | Full Text | CrossRef Full Text | Google Scholar
40. Kotsifaki DG, Serafetinides AA. Pulsed infrared radiation transmission through hollow silica waveguides. Opt Laser Technol. (2009) 41:365–73. doi: 10.1016/j.optlastec.2008.09.006
41. Matsuura Y, Kasahara R, Katagiri T, Miyagi M. Hollow infrared fibers fabricated by glass-drawing technique. Opt Express (2002) 10:488–92. doi: 10.1364/OE.10.000488
PubMed Abstract | Full Text | CrossRef Full Text | Google Scholar
42. Matsuura Y, Miyagi M. Er:YAG, CO, and CO2 laser delivery by ZnS-coated Ag hollow waveguides. Appl Opt. (1993) 32:6598–601. doi: 10.1364/AO.32.006598
PubMed Abstract | Full Text | CrossRef Full Text | Google Scholar
43. Temelkuran B, Hart SD, Benoit G, Joannopoulos JD, Fink Y. Wavelength-scalable hollow optical fibres with large photonic bandgaps for CO2 laser transmission. Nature (2002) 420:650–3. doi: 10.1038/nature01275
PubMed Abstract | Full Text | CrossRef Full Text | Google Scholar
44. Choudhri O, Karamchandani J, Gooderham P, Steinberg GK. Flexible omnidirectional carbon dioxide laser as an effective tool for resection of brainstem, supratentorial, and intramedullary cavernous malformations. Neurosurgery (2014) 10:34–44. doi: 10.1227/NEU.0000000000000212
PubMed Abstract | Full Text | CrossRef Full Text | Google Scholar
45. Xu G, Zhang W, Huang Y, Peng J. Loss characteristics of single-HE11-mode bragg fiber. J Lightwave Technol. (2007) 25:359–66. doi: 10.1109/JLT.2006.886673
46. Pryamikov AD, Biriukov AS, Kosolapov AF, Plotnichenko VG, Semjonov SL, Dianov EM. Demonstration of a waveguide regime for a silica hollow-core microstructured optical fiber with a negative curvature of the core boundary in the spectral region > 3.5 μm. Opt Express (2011) 19:1441–8. doi: 10.1364/OE.19.001441
PubMed Abstract | Full Text | CrossRef Full Text | Google Scholar
47. Yu F, Wadsworth WJ, Knight JC. Low loss silica hollow core fibers for 3–4 μm spectral region. Opt Express (2012) 20:11153–8. doi: 10.1364/OE.20.011153
PubMed Abstract | Full Text | CrossRef Full Text | Google Scholar
48. Kosolapov AF, Pryamikov AD, Biriukov AS, Shiryaev VS, Astapovich MS, Snopatin GE, et al. Demonstration of CO2-laser power delivery through chalcogenide-glass fiber with negative-curvature hollow core. Opt Express (2011) 19:25723–8. doi: 10.1364/OE.19.025723
PubMed Abstract | Full Text | CrossRef Full Text | Google Scholar
49. Litchinister NM, Abeeluck AK, Headley C, Eggleton BJ. Antiresonant reflecting photonic crystal optical waveguides. Opt Express (2002) 27:1592–4. doi: 10.1364/OL.27.001592
50. Jaworski P, Yu F, Maier RRJ, Wadsworth WJ, Knight JC, Shephard JD, et al. Picosecond and nanosecond pulse delivery through a hollow-core Negative Curvature Fiber for micro-machining applications. Optics Express (2013) 21:22742–53. doi: 10.1364/OE.21.022742
PubMed Abstract | Full Text | CrossRef Full Text | Google Scholar
51. Urich A, Maier RRJ, Yu F, Knight JC, Hand DP, Shephard JD. Flexible delivery of Er:YAG radiation at 2.94 μm with negative curvature silica glass fibers: a new solution for minimally invasive surgical procedures. Biomed Opt Express (2013) 4:193–205. doi: 10.1364/BOE.4.000193
PubMed Abstract | Full Text | CrossRef Full Text | Google Scholar
52. Urich A, Maier RRJ, Yu F, Knight JC, Hand DP, Shephard JD. Silica hollow core microstructured fibres for mid-infrared surgical applications. J Non-Cryst Solids (2013) 377:236–9. doi: 10.1016/j.jnoncrysol.2013.01.055
53. Jaworski P, Yu F, Carter RM, Wadsworth WJ, Birks TA, Knight JC, et al. High peak power nanosecond and picosecond pulse delivery through a hollow-core Negative Curvature Fiber in the green spectral region for micro-machining. In: Advanced Photonics, OSA Technical Digest (online) (Optical Society of America). Paper SoM3B.5. Barcelona (2014). doi: 10.1364/SOF.2014.SoM3B.5
54. Parry JP, Shephard JD, Hand DP, Moorhouse C, Jones N, Weston N. Laser micromachining of zirconia (Y-TZP) ceramics in the picosecond regime and the impact on material strength. Int J Appl Ceramic Technol. (2011) 8:163–71. doi: 10.1111/j.1744-7402.2009.02420.x
Keywords: microstructured fibers, hollow core fibers, beam delivery, industrial lasers, medical lasers
Citation: Shephard JD, Urich A, Carter RM, Jaworski P, Maier RRJ, Belardi W, Yu F, Wadsworth WJ, Knight JC and Hand DP (2015) Silica hollow core microstructured fibers for beam delivery in industrial and medical applications. Front. Phys. 3:24. doi: 10.3389/fphy.2015.00024
Received: 20 January 2015; Accepted: 27 March 2015;
Published: 10 April 2015.
Edited by:
Andrey D. Pryamikov, Fiber Optics Research Center of Russian Academy of Sciences, RussiaReviewed by:
Antonio Riveiro Rodriguez, University of Vigo, SpainTatiana S. Perova, The University of Dublin, Ireland
Copyright © 2015 Shephard, Urich, Carter, Jaworski, Maier, Belardi, Yu, Wadsworth, Knight and Hand. This is an open-access article distributed under the terms of the Creative Commons Attribution License (CC BY). The use, distribution or reproduction in other forums is permitted, provided the original author(s) or licensor are credited and that the original publication in this journal is cited, in accordance with accepted academic practice. No use, distribution or reproduction is permitted which does not comply with these terms.
*Correspondence: Jonathan D. Shephard, Applied Optics and Photonics Group, School of Engineering and Physical Sciences, Heriot-Watt University, Edinburgh EH14 4AS, UKai5kLnNoZXBoYXJkQGh3LmFjLnVr