Passively Q-Switched Mode-Locked Tm,Ho:CaYAlO4 Laser Based on Double-Walled Carbon Nanotube Saturable Absorber
- 1Department of Physics, Shaanxi University of Science and Technology, Xi'an, China
- 2Institute of Laser Technology, Tianshui Normal University, Tianshui, China
- 3Wireless and Optoelectronics Research and Innovation Centre, Faculty of Computing, Engineering and Science, University of South Wales, Pontypridd, United Kingdom
- 4Institute of Physics and Optoelectronics Technology, Baoji University of Arts and Sciences, Baoji, China
A passively Q-switched mode-locked (QML) operation in Tm,Ho:CaYAlO4 bulk laser was demonstrated experimentally by employing double-walled carbon nanotubes (DWCNTs) as a saturable absorber. The laser is pumped by a self-made wavelength tunable Ti:sapphire laser, and the pump threshold of Tm,Ho:CaYAlO4 laser was measured at 677 mW using transmittance of 1.5% output coupler. A stable QML operation state was achieved when the absorption pumping power reached 1,958 mW. When the pumping power reached 2.6 W, the maximum output power was 64 mw with a central wavelength of 2,085 nm, the corresponding repetition frequency of mode-locked pulse was 98.04 MHz, and the modulation depth in Q-switching envelopes is close to 100%.
Introduction
Recently, thanks to the rapid development of laser technology, a variety of lasers, including medium infrared ultrafast lasers [1, 2], ultrafast fiber lasers [3, 4] are playing an important role in more and more fields [5, 6]. Among them, ultrafast solid-state lasers emitting around eye-safe 2 μm exhibits the potential applications in LIDAR, biomedicine, time-resolved spectroscopy, atmospheric remote sensing, nonlinear frequency conversion, optical communications, etc. [7–9]. Crystal, ceramic, and glass materials doped with thulium (Tm3+), holmium (Ho3+) ions [10], or Tm3+, Ho3+ co-doped are currently the most promising candidates for 2-μm mid-infrared laser sources. The passive mode-locking technique with saturable absorber (SA) [11–13] is the most widely convenient and low-cost method to obtain ultrafast lasers at 2-μm wavelength. Up to now, SAs such as semiconductor saturable absorber mirrors (SESAMs) [14–16], carbon nanotubes [17–19], graphene [20–22], and transition metal dichalcogenides (TMDs) [23, 24] have been adopted for passive Q-switching or mode-locking operations. However, SESAMs have the disadvantage of having narrow bandwidth, complex fabrication, and high cost to limit the application and development of mid-infrared ultrafast lasers. Therefore, it is very important to develop 2-μm wavelength ultrafast lasers based on new materials.
In recent years, a batch of new 2D nanomaterials with unique properties has received widespread attention. Among them, carbon nanotubes are favored as new SA in the field of ultrashort pulse laser due to their excellent electrical, optical, and mechanical properties. According to the number of graphite layers, carbon nanotubes are divided into single-walled carbon nanotubes (SWCNT) and multiwalled carbon nanotubes [25]. It has been reported that SWCNT-SAs can passively mode lock in 0.8–2-μm laser [26–28]. As the simplest multiwalled carbon nanotubes, double-walled carbon nanotubes (DWCNTs) are made of two layers of graphite, which are coiled according to a certain helix angle. The diameters of the inner and outer walls are 0.8–1.1 and 1.6–1.8 nm, respectively, and the spacing between the inner and outer layers is 0.34–0.39 nm [29]. Under the same irradiation condition, DWCNT conductivity is better due to its higher chemical stability and smaller energy gap than SWCNT [30]. Meanwhile, DWCNT has the characteristics of relatively low cost, relatively short relaxation time, and the advantages for mass production. Therefore, DWCNT-SAs has ultra-short recovery time, wider absorption band, and higher damage threshold in a 1–2-μm band [31]. Now, the reports on mode-locked DWCNT-SAs are mainly focused on the solid-state and fiber lasers at 1-μm band [32, 33], while the reports on mode-locked DWCNT-SAs at 2-μm band are few, and the output power is lower than 200 mW [34–36]. For example, Wang et al. [37] achieved a mode-locked operation of 0.98 ps in Tm3+-doped silica fiber, and Qu et al. [38] achieved a mode-locked operation in Tm:YAP laser. Over the years, our group has been devoted to the research of ultrafast laser technology in the mid-infrared band. In 2018, our group realized the Q-switched and mode-locked simultaneous operation with low threshold based on the DWCNTs in the Tm,Ho:LLF laser, and the maximum output power was 234 mW [39].
CaYAlO4(CYA) crystal belongs to the perovskite structure, which is an excellent laser medium matrix material fabricated by the Czochralski method [40]. Tm,Ho:CaYAlO4 crystals have higher absorption efficiency and wider tuning width, and their main absorption peaks are 691, 797, 1,212, and 1,694 nm [41]. Currently, there are few researches on related mode locking of this crystal. In 2018, only Zhao et al. realized continuous mode-locking operation in Tm,Ho:CaYAlO4 laser based on SESAM [10].
In this paper, a stable simultaneously Q-switched mode-locking (QML) operation was experimentally demonstrated for the first time in Tm,Ho:CaYAlO4 crystal by using DWCNTs-SA. The pump source was a self-made wavelength-tunable Ti:sapphire solid laser. With 1.5% output coupler, the maximum output power of QML is 64 mw at the central wavelength of 2,085 nm, the repetition frequency of mode-locked pulse in Q-switched envelope is 98.04 MHz, and the modulation depth was close to 100%.
Experimental System
The experimental setup of the passive mode-locking Tm,Ho:CaYAlO4 laser is shown in Figure 1 [9]. A typical X-type five-mirror cavity structure is adopted to obtain better pattern matching effect, which is composed of a typical X-type four-mirror folded cavity and focused concave mirror. The laser is pumped by a self-made Ti:sapphire solid-state laser with an output wavelength of 798 nm. The laser crystal of Tm,Ho:CaYAlO4 with 6% Tm3+ and 0.5% Ho3+-doped was cut at the angle of Brewster. Its size is 3 × 3 × 4 mm, and the strongest absorption peak is 798 nm. In order to reduce the thermal lensing effect of crystal and mitigate the thermal load, it is necessary to cool the laser crystal to ensure the stable operation of the laser. Here, the laser crystal is wrapped in indium foil and mounted in a copper heat sink, which is cooled by circulating water at a constant temperature of 12°C [42]. The standard X-folded cavity consisted of M1, M2, M3, M4, and an output coupler (M5). In Figure 1, M1 and M2 are 2-μm pump mirrors produced by Layertec company with curvature radii of 100 and 75 mm, respectively, whose transmittance are higher than 95% in the wavelength range from 770 to 1,050 nm, and reflectivity is higher than 99.9% at 2-μm wave bands. M3 is a plane-concave reflector with the curvature radius of concave surface of 100 mm, and M4 is a planar reflector. The reflectivity of both flat concave mirror M3 and flat reflector M4 is >99.9% for oscillating light at 2-μm wave bands. M5 is an output coupler with partial transmission for oscillating light. In the experiment, the output mirror with a transmittance of 1.5% was selected to obtain a high intracavity power density. DWCNTs were inserted before the M4 plane mirror as SA. The collimated pump light is incident into the Tm,Ho:CaYAlO4 crystal by a focusing lens (f = 150 mm) with higher than 95% transmittance for 798 nm. The laser beam diameter is 54 μm on the surface of SA, which is calculated using the laser cavity mode ABCD propagation matrix theory.
Experimental Results and Discussion
The absorption and output characteristics of the laser are shown in Figure 2. First, the absorption efficiency of the laser crystal of Tm,Ho:CaYAlO4 is shown in Figure 2A [43]. It can be seen that the absorption efficiency of laser crystal to the pump light at 798 nm is 89.7% due to a large amount of pump light that is absorbed by the crystal when there is no laser running in the cavity. When continuous wave (CW) operation in the cavity is realized, the absorption efficiency of the laser crystal increases to about 91.6% due to the large number of upper-level particles returning to the lower level under stimulated radiation. After inserting the DWCNT-SA into the cavity, the operating state of QML is achieved, and the absorption efficiency of the laser crystal did not change significantly and still remained around 91.6%.
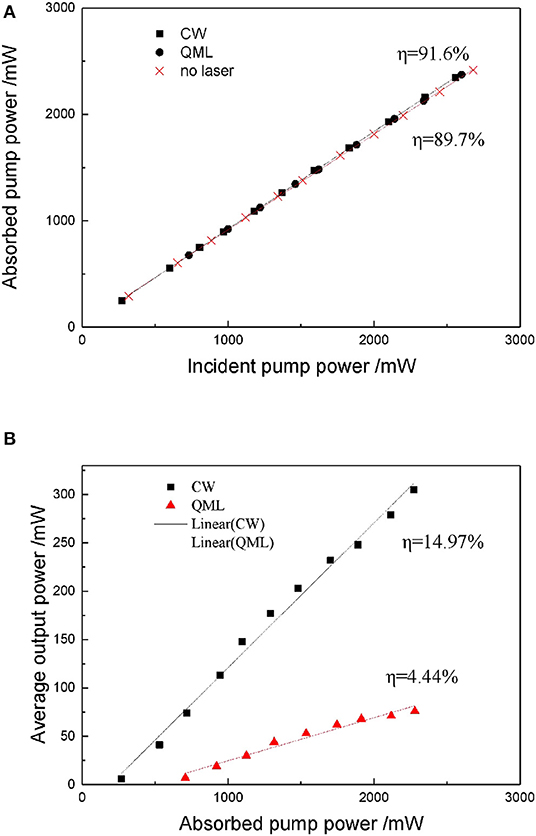
Figure 2. Experimental data diagram. (A) The dependence of absorbed power on incident pump power. (B) The dependence of average output power on absorbed pump power under CW and QML.
Next, the average output power as a function of the absorbed pump power under CW and QML is plotted in Figure 2B. In the experiment, 1.5% output coupling mirror is used. When the laser is in CW operation, the laser threshold power is 249 mW, the maximum output power is 301 mW, and the corresponding slope efficiency is 14.97%. When DWCNT-SA was inserted before M4 in the cavity, the laser threshold power increased to 677 mW. When the absorbed pump power reached 1,958 mW, laser entered a stable QML operation, and the maximum output power under the same conditions was 64 mW, which has a corresponding slope efficiency of 4.44%. Here, the main reason why we choose 1.5% output coupler is that it can provide high intracavity power to start QML.
Figure 3 shows the typical spectra of the mode-locked pulse measured with a spectrometer (AvaSpecNIR256-2.5TEC) [9]. As can be seen from Figure 3, the central wavelength of a mode-locked laser pulse is 2,085 nm, and the full width at half-maximum bandwidth is about 13 nm. A 2-μm fast photodiode (ET-5000) was used to connect a 200-MHz digital oscilloscope (RIGOL, DS4024) to detect QML pulse sequences. Figure 4 shows the QML pulse sequences, which is obtained by scanning times of (a) 1 ms, (b) 100 μs, and (c) 10 ns. When the output power reaches the maximum, the pulse width of the Q-switched envelope is 12 μs, the repetition frequency is 83.33 kHz, the frequency of the mode-locked pulse is 98.04 MHz, and the modulation depth of the mode-locked pulse is close to 100%, which is consistent with the theoretical repetition frequency corresponding to the 1.5-m cavity length.
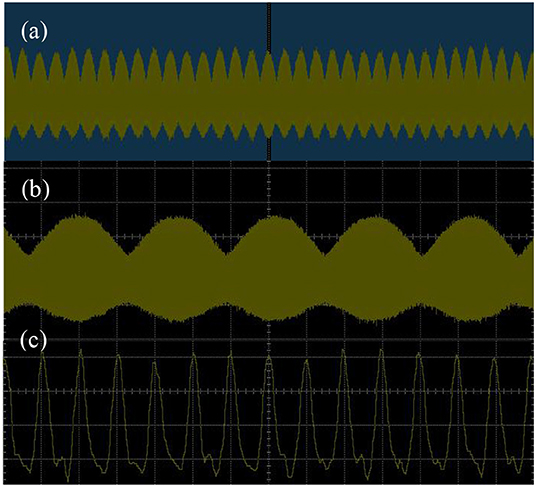
Figure 4. Mode-locked pulse sequence recorded in (A) 1 ms, (B) 100 μs, and (C) 10 ns per division (div) timescales.
QML is in the transition state from Q-switch to CW mode locking [44–46], Since an autocorrelator is only suitable for measuring the pulse width of a CW mode-locked pulse, a QML pulse cannot obtain autocorrelation envelope because of the envelope modulation of kilohertz, so we can only estimate the pulse duration roughly in theory.
Hence, formula (1) is used to calculate the width of the mode-locked pulse [47].
Here, tm is the rising edge time of the measured mode-locked pulse, tr is the rising edge time of the actual mode-locked pulse, tp is the rising edge time of the photodetector, and t0 is the rising edge time of the oscilloscope. In the experiment, the rising edge time of the mode-locked pulse is about 2.1 ns, and the rising edge time of the photodetector is about 35 ps. t0 can be estimated as 2,000 ps using formula (2) as follows.
Among them, Wb is the bandwidth of the oscilloscope, which is 200 MHz in the experiment. Therefore, it can be calculated that the rise time of a mode-locked pulse is about 639.35 ps. Since the actual mode-locked pulse width is about 1.25 times the rising edge time, it is calculated as 799.2 ps.
Conclusion
A passively Q-switched mode locking operation is realized experimentally by using DWCNT-SA in Tm,Ho:CaYAlO4 all-solid laser for the first time. After DWCNT-SA was added into the resonator cavity, the pump threshold of Tm,Ho:CaYAlO4 solid-state laser was measured as 677 mW using the transmittance of 1.5% output coupler. When the absorption pumping power reached 1,958 mW, Tm,Ho:CaYAlO4 solid state laser entered a stable QML operation state. When the pump power was 2.6 W, the maximum output power was 64 mw at the central wavelength of 2,085 nm, the mode-locked pulse repetition frequency is 98.04 MHz, and the modulation depth is close to 100%. The experimental results show that DWCNT-SA can be used as a quick starting element for passively QML solid state laser of 2-μm band, which has an important development and application value.
Data Availability Statement
All datasets generated for this study are included in the article/supplementary material.
Author Contributions
YZ was the author of the experimental scheme and the general director of the project. WL was the specific guidance for postgraduates during the experiment. DQ, who is a doctoral student, and RS and CC, who are graduate students of the research group, have implemented the experimental scheme.
Funding
This work was supported by the National Key Research and Development Program (2017YFB045200), the National Science Foundation of China (Nos. 11774257, 61564008), the International Science and Technology Cooperation and Exchanges Project of Shaanxi (No. 2018KW-016), the Key Sciences and Technology Project of Baoji City (No. 2015CXNL-1-3), and the Open Research Fund of State Key Laboratory of Transient Optics and Photonics (No. SKLST201802).
Conflict of Interest
The authors declare that the research was conducted in the absence of any commercial or financial relationships that could be construed as a potential conflict of interest.
References
1. Hui ZQ, Xu WX, Li XH, Guo PL, Zhang Y, Liu JS Cu2S nanosheets for ultrashort pulse generation in the near-infrared region Nanoscale. (2019) 11:6045–51. doi: 10.1039/C9NR00080A
2. Ma J, Qin ZP, Xie GQ, Qian LJ, Tang DY Review of mid-infrared mode-locked laser sources in the 2.0 μm−3.5 μm spectral region, Appl Phys Rev. (2019) 6:021317. doi: 10.1063/1.5037274
3. Liu X, Han D, Sun Z, Zeng C, Lu H, Mao D, et al. Versatile multi-wavelength ultrafast fiber laser mode-locked by carbon nanotubes. Sci Rep. (2013) 3:2718. doi: 10.1038/srep02718
4. Li XH, Wu K, Sun ZP, Meng B, Wang YG, Wang YS, et al. Single-wall carbon nanotubes and graphene oxide-based saturable absorbers for low phase noise mode locked fiber lasers, Sci Rep. (2016) 6:25266. doi: 10.1038/srep25266
5. Duan XM, Chen C, Ding Y, Yao BQ, Wang YZ. Widely tunable middle infrared optical parametric oscillator pumped by the q-switched Ho: GdVO4 laser. Chin Phys Lett. (2018) 35:054205. doi: 10.1088/0256-307X/35/5/054205
6. Zhao Y, Guo P, Li X, Jin Z Ultrafast photonics application of graphdiyne in optical communication region. Carbon. (2019) 149:336–41. doi: 10.1016/j.carbon.2019.04.075
7. Wang J, Sramek C, Paulus YM, Lavinsky D, Schuele G, Anderson D, et al. Retinal safety of near-infrared lasers in cataract surgery. J Biomed Opt. (2012) 17:95001. doi: 10.1117/1.JBO.17.9.095001
8. Van Leeuwen TG, Jansen ED, Motamedi M, Welch AJ, Borst C. Excimer laser ablation of soft tissue: a study of the content of rapidly expanding and collapsing bubbles. IEEE J Quant Electron. (2002) 30:1339–45. doi: 10.1109/3.303700
9. Keller U, Weingarten KJ, Kartner FX, Kopf D, Braun B, Jung ID, et al. Semiconductor saturable absorber mirrors (SESAM's) for femtosecond to nanosecond pulse generation in solid-state lasers. IEEE J. Sel. Top. Quantum Electron. (1996) 2:435–53. doi: 10.1109/2944.571743
10. Zhao Y, Wang Y, Zhang X, Mateos X, Pan ZB, Loikoet P, et al. 87 fs mode-locked Tm, Ho: CaYAlO 4 laser at ~ 2043 nm. Opt Lett. (2018) 43: 915–8. doi: 10.1364/OL.43.000915
11. Ling WJ, Xia T, Dong Z, You LF, Zhang MX, Zuo YY, et al. Passively mode-locked Tm, Ho:LLF laser at 1895 nm. J Opt. (2019) 48:209–13. doi: 10.1007/s12596-019-00528-y
12. Kong LC, Xie GQ, Yuan P, Qian LJ, Wang SX, Yu HH, et al. Passive Q-switching and Q-switched mode-locking operations of 2 μm Tm: CLNGG laser with MoS2 saturable absorber mirror. Photon Res. (2015) 3:A47–A50. doi: 10.1364/PRJ.3.000A47
13. Liu JS, Li XH, Guo YX, Qyyum A, Shi ZJ, Feng TC, et al. SnSe2 nanosheets for sub-picosecond harmonic mode locked pulse generation. Small. (2019) 15:1902811. doi: 10.1002/smll.201902811
14. Li JF, Luo HY, He YL, Liu Y, Zhang L, Zhou KM, et al. Semiconductor saturable absorber mirror passively Q-switched 2.97 μm fluoride fiber laser. Laser Phys Lett. (2014) 11:065102. doi: 10.1088/1612-2011/11/6/065102
15. Yamashita S, Inoue Y, Maruyama S, Murakami Y, Yaguchi H, Jablonski M, et al. Saturable absorbers incorporating carbon nanotubes directly synthesized onto substrates and fibers and their application to mode-locked fiber lasers. Opt Lett. (2004) 29:1581–583. doi: 10.1364/OL.29.001581
16. Liu J, Li YQ, Zheng LH, Su LB, Xu J, Wang YG, Passive Q-switched mode locking of a diode-pumped Tm: SSO laser near 2 μm Laser. Phys Lett. (2013) 10:105812. doi: 10.1088/1612-2011/10/10/105812
17. Chen XT, Zhao SZ, Zhao J, Yang KJ, Li GQ, Li DC, et al. Sub-100 ns passively Q-switched Nd: LuAG laser with multi-walled carbon nanotube. Opt Laser Technol. (2014) 64:7–10. doi: 10.1016/j.optlastec.2014.04.019
18. Ling WJ, Xia T, Dong Z, Zuo YY, Li K, Liu Q, et al. Passively Q-switched mode-locked low threshold Tm,Ho:LLF laser with an single walled carbon nanotubes saturable absorber. Acta Phys Sin. (2018) 67:92–7. doi: 10.7498/aps.67.20171748
19. Feng C, Liu DH, Liu J. Graphene oxide saturable absorber on golden reflective film for Tm:YAP Q-switched mode-locking laser at 2 μm J. Mod. Opt. (2012) 59:1825–8. doi: 10.1080/09500340.2012.747633
20. Ma J, Xie GQ, Lv P, Gao WL, Yuan P, Qian LJ, et al. Wavelength versatile graphene-gold film saturable absorber mirror for ultra broadband mode-locking of bulk lasers. Sci Rep. (2014) 4:5016. doi: 10.1038/srep05016
21. Li XH, Yu XH, Sun ZP, Yan ZY, Sun B, Cheng YB, et al. High-power graphene mode-locked Tm/Ho co-doped fiber laser with evanescent field interaction. Sci Rep. (2015) 5:16624. doi: 10.1038/srep16624
22. Ma YF, Sun HY, Ran BF, Zhang SC, Zhang H, Tittel FK, et al. Passively Q-switched Tm:YAlO3 laser based on WS2/MoS2 two-dimensional nanosheets at 2 μm. Opt Laser Technol. (2020) 126:106084–9. doi: 10.1016/j.optlastec.2020.106084
23. Ling WJ, Xia T, Dong Z, Liu Q, Wang YG. Passively Q-switched mode-locked Tm,Ho:LLF laser with a WS2 saturable absorber. Acta Phys Sin. (2017) 11:156–61. doi: 10.1364/ACPC.2017.Su2A.165
24. Feng JJ, Li XH, Shi ZJ, Zhen C, Li XW, Leng DY, et al. 2D ductile transition metal chalcogenides (TMCs): a novel high performance Ag2S nanosheets for ultrafast photonics. Adv Opt Mater. (2019) 8:1901762. doi: 10.1002/adom.201901762
25. Lin XC, Zhang L, Tsang YH, Wang YG, Yu HJ, Yan SL, et al. Multi-walled carbon nanotube as a saturable absorber for a passively mode-locked Nd:YVO laser. Laser Phys Lett. (2013) 10:055805. doi: 10.1088/1612-2011/10/5/055805
26. Rotermund F, Cho WB, Choi SY, Baek IH, Yim JH, Lee S, et al. Mode-locking of solid-state lasers by single-walled carbon-nanotube based saturable absorbers. Quant Electron. (2012) 42:663–70. doi: 10.1070/QE2012v042n08ABEH014775
27. Schmidt A, Choi SY, Yeom D I, Rotermund F, Mateos X, Segura M, et al. Femtosecond pulses near 2 μm from a Tm:KLuW laser mode-locked by a single-walled carbon nanotube saturable absorber. Appl Phys Express. (2012) 5:2704. doi: 10.1143/APEX.5.092704
28. Schmidt A, Koopmann P, Huber G, Fuhrberg P, Choi SY, Yeom D, et al. 175 fs Tm:Lu2O3 laser at 2.07μm mode-locked using single-walled carbon nanotubes. Opt Express. (2012) 20:5313. doi: 10.1364/OE.20.005313
29. Hasan T, Sun Z, Tan P, Popa D, Flahaut E, Kelleher EJR, et al. Double-wall carbon nanotubes for wide-band, ultrafast pulse generation. ACS Nano. (2014) 8:4836–47. doi: 10.1021/nn500767b
30. Maeng I, Kang C, Oh S J, Son JH, An K H, Lee YH. Terahertz electrical and optical characteristics of double-walled carbon nanotubes and their comparison with single-walled carbon nanotubes. Appl Phys Lett. (2007) 90:56. doi: 10.1063/1.2435338
31. Yang Q, Wang YG, Liu DH, Liu J, Zheng LH, Su LB, et al. Dual-wavelength mode-locked Yb:LuYSiO5 laser with a double-walled carbon nanotube saturable absorber. Laser Phys Lett. (2012) 9:135–40. doi: 10.1002/lapl.201110111
32. Zhang L, Wang YG, Yu HJ, Sun L, Hou W, Lin XC, et al. Passive mode-locked Nd:YVO4, laser using a multi-walled carbon nanotube saturable absorber. Laser Phys. (2011) 21:1382–6. doi: 10.1134/S1054660X11150333
33. Cheng KN, Lin YH, Lin GR. Single-and double-walled carbon nanotube based saturable absorbers for passive mode-locking of an erbium-doped fiber laser. Laser Phys. (2013) 23:045105. doi: 10.1088/1054-660X/23/4/045105
34. Liu J, Wang Y, Qu Z, Fan X. 2 μm passive Q-switched mode-locked Tm3+: YAP laser with single-walled carbon nanotube absorber. Opt Laser Technol. (2012) 44:960–2. doi: 10.1016/j.optlastec.2011.11.001
35. Martinez A, Fuse K, Xu B, Yamashita S. Optical deposition of graphene and carbon nanotubes in a fiber ferrule for passive mode-locked lasing. Opt Express. (2010) 18:23054–61. doi: 10.1364/OE.18.023054
36. Cho WB, Schmidt A, Yim JH, Choi SY, Lee S, Rotermund F, et al. Passive mode-locking of a Tm-doped bulk laser near 2 μm using a carbon nanotube saturable absorber. Opt Express. (2009) 17:11007–12. doi: 10.1364/OE.17.011007
37. Wang F, Jiang Z, Hasan T, Sun Z, Popa D, Torrisi F, et al. Double-Wall Carbon Nanotube Q-Switched and Mode-Locked Two-Micron Fiber Lasers. In: Conference On Lasers and Electro-Optics. San Jose, CA: IEEE (2012). doi: 10.1364/CLEO_SI.2012.CF1N.4
38. Qu ZS, Wang YG, Liu J, Zheng LH, Su LB, Xu J. Passively mode-locked 2μm Tm:YAP laser with a double-wall carbon nanotube absorber. Chin Phys B. (2012) 21:064211. doi: 10.1088/1674-1056/21/6/064211
39. Ling W, Xia T, Dong Z, Zhang M, Zuo Y, Li K, et al. Low threshold 1895 nm mode-locked laser based on double wall carbon nanotubes. Acta Opt Sin. (2018) 38:0614001. doi: 10.3788/AOS201838.0614001
40. Kong LC, Qin ZP, Xie GQ, Xu XD, Xu J, Yuan P, et al. Dual-wavelength synchronous operation of a mode-locked 2-μm Tm:CaYAlO 4 laser. Opt Lett. (2015) 40:356–8. doi: 10.1364/OL.40.000356
41. Di JQ, Zhou DH, Xu XD, Xia CT, Gao W, Zheng H, et al. Spectroscopic properties of Tm, Ho: CaYAlO4 single crystal. Cryst Res Technol. (2014) 49:446–51. doi: 10.1002/crat.201300347
42. Wang X, Wang Y, Mao D, Li L, Chen ZD. Passively Q-switched Nd: YVO4 laser based on Fe3O4 nanoparticles saturable absorber. Optic Mater Express. (2017) 7:2913–21. doi: 10.1364/OME.7.002913
43. Ling WJ, Xia T, Sun R, Chen C, Xu Q, Zhang YN. Low threshold, high efficiency passively mode-locked picosecond Tm, Ho: LiLuF4 laser. Front Phys. (2020) 7:216. doi: 10.3389/fphy.2019.00216
44. Liu X., Popa D., Akhmediev N. Revealing the transition dynamics from Q switching to mode locking in a soliton laser. Phys Rev Lett. (2019) 123:093901. doi: 10.1103/PhysRevLett.123.093901
45. Liu X., Pang M.. Revealing the buildup dynamics of harmonic mode-locking states in ultrafast lasers. Laser Photon Rev. (2019) 13:1800333. doi: 10.1002/lpor.201800333
46. Liu X., Cui Y.. Revealing the behavior of soliton buildup in a mode-locked laser. Adv Photon. (2019) 1:016003. doi: 10.1117/1.AP.1.1.016003
Keywords: Tm,Ho:CaYAlO4 laser, passively Q-switched mode-locked, double-walled carbon nanotube, saturable absorber, resonant cavity
OCIS codes: 140.3580 (Lasers, solid-state), 140.3070 (Infrared and far-infrared lasers), 140.3380 (Laser materials), 140.4050 (Mode-locked lasers), 140.3410 (Laser resonators)
Citation: Zhang Y, Ling W, Qiao D, Sun R and Chen C (2020) Passively Q-Switched Mode-Locked Tm,Ho:CaYAlO4 Laser Based on Double-Walled Carbon Nanotube Saturable Absorber. Front. Phys. 8:86. doi: 10.3389/fphy.2020.00086
Received: 20 January 2020; Accepted: 10 March 2020;
Published: 15 April 2020.
Edited by:
Xiaohui Li, Shaanxi Normal University, ChinaReviewed by:
Yufei Ma, Harbin Institute of Technology, ChinaZhan-Qiang Hui, Xi'an University of Posts and Telecommunications, China
Copyright © 2020 Zhang, Ling, Qiao, Sun and Chen. This is an open-access article distributed under the terms of the Creative Commons Attribution License (CC BY). The use, distribution or reproduction in other forums is permitted, provided the original author(s) and the copyright owner(s) are credited and that the original publication in this journal is cited, in accordance with accepted academic practice. No use, distribution or reproduction is permitted which does not comply with these terms.
*Correspondence: Weijun Ling, wjlingts@sina.com