- 1State Key Laboratory of Radiation Medicine and Protection, School of Radiation Medicine and Protection, Institute of Space Life Sciences, Medical College of Soochow University, Collaborative Innovation Center of Radiological Medicine of Jiangsu Higher Education Institutions, Suzhou, China
- 2Biophysics Lab, Physics Department, Faculty of Science, Al-Azhar University, Cairo, Egypt
- 3Department of Health Physics and Diagnostic Sciences, University of Nevada, Las Vegas, NV, United States
The radiation exposure of individuals has been on the rise due to an increased amount of radiation use, e.g., in medicine for diagnostic imaging and treatment procedures, industrial applications including military defense activities and nuclear power plants, and in academics for educational and scientific research. Space exploration missions and space tourism are additional areas of protracted low dose exposure situations with radiation types not present on the Earth. In contrast to high doses of ionizing radiation, cancer risk assessment of the more commonly encountered or protracted radiation exposure is still under debate and uncertainty making it fuzzy area. A major challenge lies in providing a scientific basis to estimate low dose radiation carcinogenesis risks. In this review we aim, through the collected epidemiological and experimental studies' data, to address the central questions in radiological protection; including quantification of the risks and uncertainties from low doses of ionizing radiation and what is a sound scientific consensus to advise on risk perception for low dose radiation exposure.
Introduction
Health effects of exposure to ionizing radiation were identified shortly after the discovery of X-rays in 1895. Epilation was first probed then skin burns documented soon after [1]. With the invention of high voltage X-ray tubes and their implementation in medical clinics, injuries to tissues, known as tissue reactions, are a sequela of penetration of large amount of radiation into the body.
The carcinogenic effects of ionizing radiation are late effects that occur with a probability that depends on radiation dose. Cancer risk of low dose radiation has become an essential component of radiation protection and has attracted public and social concerns about safety in relation to variety of issues, such as medical imaging tests for the early detection of defeats, the future of nuclear power, environmental radiation exposure from terrestrial radon, nuclear weapons test fallout, radiological terrorism and human space exploration. For example, most radiological examinations produce doses in the range of 3–30 mSv. Obviously, high doses of ionizing radiation (>100 mSv) increases cancer risk [2], while at lower doses the situation is much less clear. Epidemiological studies suggest that the lowest dose value of ionizing radiation at which good evidence of increased cancer risks in human exists is ≈10–50 mSv for an acute exposure [3] and ≈50–100 mSv for prolonged exposure [4].
In order to quantify the risk of low dose radiation, large epidemiological studies are needed to get a useful degree of precision. For example, if excess cancer death cases have been recorded in sample size of 500 persons in response to 1,000 mSv dose exposure, then sample size of 50,000 would be needed for documenting the carcinogenic effect of 100 mSv, and ≈5 million for 10 mSv dose. In other words, the sample size should increase as the inverse square of the dose in order to maintain the statistical precision and power [5]. For several decades, the Linear non-threshold (LNT) model has been the standard risk assessment used by the radiation protection community to determine the health outcomes associated with low doses by means of extrapolation from the risk assessed at high doses [6], ICRP publications 99 and 103 [7, 8], UNSCEAR 2012 and 2017 reports [9, 10], and the BEIR VII report [11]. The LNT relationship is a practical way to fit limited epidemiology data. However, LNT is also often cast in terms of biophysical hypotheses, such as: (a) Damage induction is directly proportional to dose, from 1 mGy to 100 Gy; (b) mis-repair of DNA double-strand break (DSB) is thought to have a probability of inducing invasive neoplastic cell transformation, irrespective of DSB baseline rate and dose delivered to the cell. Intrinsic defense tools against carcinogenesis, such as DNA repair and programmed cell death, make the LNT model obsolete. It is regularly argued that the LNT model is overprotective and low-level radiation exposure may have health benefits as a set of data showed that these countermeasures are higher at low doses than at high doses and for fractionated or protracted irradiation than for acute irradiation [12]. By contrast, some biological effects of radiation, such as persistent transmissible genomic instability and bystander phenomena [13] could increase cancer risk above extrapolation [14]. The current risk estimation, depicted in Figure 1, is to extrapolate radiation-induced cancer risks from higher doses, where the risk is assessed epidemiologically, to lower doses.
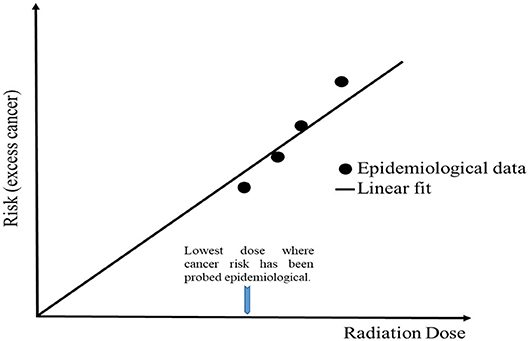
Figure 1. LNT dose response model for radiation-induced stochastic health effects estimation. Its straight line extrapolated to zero assuming radiation has the potential to cause lesions at any dose value.
Nuclear disaster causes additional negative effects on public perception concerning radiation risk, and results in overestimating health risks of radiation exposure even at extremely low levels of radiation (several mSv). Such public confusion in South Korea after the Fukushima accident resulted in temporary closures of schools, massive selling of radioprotective masks and refusal of Japanese farming products. All of these actions were adopted by public even in absence of strong evidence for radioactive contamination according to official announcements from the Korean government [15]. Radiation experts (biologists, epidemiologists, and physicists) should be able to reduce societal confusion about the health risk of low dose radiation exposure based on the experimental results and population-based observational data. Several low-dose exposure scenarios are identified.
Nuclear Emergency and War-Time Exposures
Japanese survivors of the atomic bombing in Hiroshima and Nagasaki are thought to be the most reliable source of information about long-term effects of radiation exposure on health because of the large size of the cohort of over 100,000 persons, consisting of both sexes and all ages, and a wide range of individually assessed doses. Radiation-associated excess rates of leukemia and solid cancers have schematically summarized in Figure 2.
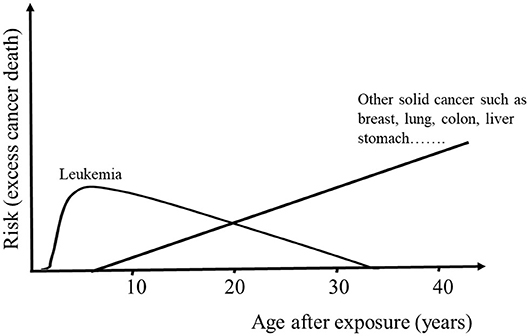
Figure 2. Schematic overview of the documented findings regarding the late effect of the bomb-released radiation on cancer mortality throughout life. Leukemia risk shows a linear-quadratic response; increased in the early period after bombing then decreased, whereas other solid cancers followed a linear manner; continuing to increase as the cohort ages.
Humanity has experienced these atomic bombs and other nuclear disasters, such as Chernobyl accident in 1986 and the latest devastating accident to date; Fukushima Daiichi NPP in 2011. Survivors of the nuclear bomb, who have not died from injuries produced by blast and heat from the bomb, have a radiation-related increased risk of cancer owing to late-onset effect of radiation, 60% of whom have doses of at least 5 mSv, and people exposed as children have a higher radiation-induced cancer risk than those exposed at older ages; the excess relative risk increased with dose for both utero and early childhood with values of 1.0 and 1.7 per Sv, respectively [16, 17]. Additionally, the cancer risk declines with increasing age; for those exposed at age 30, the solid cancer risk is elevated by 47% per Sv above those at age 70 [18]. In addition to breast, ovary, bladder, lung, liver, nervous system and thyroid [19], radiation-associated increase in risk was reported for digestive and other respiratory systems [17, 18]. On the other hand, no increased risks for malignancies or other diseases have been observed in children who were conceived after parental exposure to bomb-released radiation [20] but continuing investigations is indispensable since the large number of additional cases provides a more stable database, needed for establishing limits and recommendations for radiation protection.
2020 marked the 34th anniversary since the Chernobyl nuclear power plant explosion in northern Ukraine. An adequate number of publications are dedicated to observing the consequences of the Chernobyl disaster that resulted in a massive release of radionuclides into the environment, affecting large nearby areas, Ukraine, Belarus and Russian Federation. Environmental exposure to 131I carries an increased risk of thyroid cancer [21] and the risk is the greatest to those who were children at the time of exposure [22]. So, studies in clinical and pathological features of patients with post-Chernobyl papillary thyroid carcinoma have focused on children, who were 2 years old or less at the time of Chernobyl accident [23], as the most vulnerable group with the highest risk of developing cancer. Data came from Tronko et al. [24] demonstrating a strong association between 131I and thyroid neoplasia risk including thyroid cancer and follicular adenoma (FA) for individuals who were <18 years old at the time of the accident with an excess odd ratio per Gy of 1.36 and 2.03, respectively. The excess risk is set to persist nearly three decades after exposure and underscore the importance of continued follow-up of this cohort to characterize long term patterns of 131I risk. Finally, lens opacities were observed, particularly among interventional radiologists who may receive substantial lens doses. Evidence for genetic effects among exposed persons was inconsistent [22]. Finland and Sweden were among the countries most heavily affected by the radioactive fallout that spread out after the Chernobyl crisis. Many papers have appeared and claim to analyse the overall cancer incidence in relation to radiation dose from the Chernobyl accident in both the Finnish and Swedish populations [25–27]. Comprehensive cohort analysis did not show variation in the cancer incidence in relation to radiation exposure in any calendar period, or any subgroup by sex or age at the time of the accident. An analogous study failed to distinguish the effect of 137Cs, released from Chernobyl accident, on cancer incidence in Sweden.
The United States carried out numerous nuclear weapon tests (>800 underground and >200 atmospheric atomic detonations) of the over 2,000 nuclear explosions that were conducted worldwide in the five decades from 1945 to 1996. A cohort of 115,329 American veterans has been assembled for the purpose of epidemiological research and compensation. Both red bone marrow and male breast doses have been estimated for approximately a 2,000-person subset of the veteran cohort to perform risk analyses for leukemia and male breast cancer mortality [28] but the results have not yet been published. Approximately two-thirds of participants received a total dose to red bone marrow of 5 mGy with little variability between test site or among military branches. Male breast doses were ~20% higher than those of red bone marrow [29]. These dosimetry results indicate a need to continue close monitoring of this cohort for better understanding and prediction of disease risk following low dose exposures and to develop biologically-based dose response models [30].
Occupational Exposure
Researchers have been trying to estimate the cancer risks of prolonged exposure to very low doses of ionizing radiation, which might be received from medical scans or from nuclear industry related work. Occupational doses from five different job categories are assessed and summarized in Table 1. Developed nuclear programs in USA, UK and France have employed hundred thousand of workers over the past years. The primary quantitative basis for radiation protection standard comes from epidemiological studies of survivors of atomic bombing of Hiroshima and Nagasaki in which people were exposed to varying doses of ionizing radiation. The National Radiological Protection Board (NRPB) defined “low dose” as values below 100 mGy for acute low dose exposures and below 5 mGy per hour for low dose rate. National Registry for Radiation Workers (NRRW-3) reported workers with individual accumulative dose value above 100 mGy, higher than the upper limit for “low dose” delivered acutely because no deviation in the dose-response from linearity has been reported, additionally total individual dose has accumulated over a prolonged time interval.
Risks associated with protracted low dose exposure are more relevant to health practitioners and nuclear-industry workers. Many of these workers have received low (an average of 11 mSv/y), above background doses of radiation which itself is about 2.3 mSv/y from sources, such as cosmic rays and radon [3], and their radiation doses have been monitored carefully overtime through the use of personal dosimeters.
The International Nuclear Workers study (INWORKS) was conducted in order to strengthen the scientific basis for protecting people from Low dose protracted or intermittent radiation exposure. This cohort includes workers from USA, UK and France who have received a precisely known dose and have been followed up to 60 years after exposure. The linear increase in the relative rate of cancer with a cumulative dose by 48% per Gy was summarized; of 66,632 known death by the end of fellow-up, 17,957 were due to solid cancer [32]. Strikingly, the cancer risk per unit of radiation dose among radiation workers was similar to the estimate that comes from studies of Japanese atomic bomb survivors [32]. Leuraud et al.'s [33] study confirmed that the risk of leukemia rose with prolonged low dose radiation exposure, although the rise was minuscule. This study provided very strong evidence of positive association between long term low dose radiation exposure and leukemogenesis; the excess relative risk of leukemia mortality excluding chronic lymphocytic leukemia was 2.96 per Gy. The International Commission on Radiological Protection (ICRP) recommendations, which most radiation-protection authorities follow, call for the monitoring of individuals whose annual exposure exceed 6 mSv. They restrict exposure to 20 mSv annually over 5 years, with maximum of 50 mSv in any 1 year [34]. These low dose limits are adopted by ICRP to ascertain that risks and benefits of practices on ionizing radiation are balanced and to provide a border between tolerable and intolerable radiation doses.
Space Exploration
On 1992, the Chinese government announced the manned space exploration program and approved the “3 steps” development strategy which planned to end by building a space station to conduct experiments on a large scale with long-term human participation. Between 1999 and 2002, Four preparatory unmanned spacecrafts, SZ-1 to SZ-4, have been successfully launched to test key equipment and technology in the spacecraft and assess the space environment risk on representative living systems, from the cellular level to the whole organism. Exploration activity has dramatically increased over the past 20 years. To date, 11 “Shenzhou” spacecrafts, Tiangong-1 aircraft, and Tiangong-2 Space Laboratory have been successfully launched. A large number of scientific experiments have been carried out smoothly, such as monitoring space radiation doses, assessing radiation health risks, and other exploratory studies which are considered to be a technical platform for the successful establishment of the Chinese Space Station (CSS) in 2022 [35]. There are many destinations for human space exploration, including the moon, low earth orbit (LEO), and Mars. Space radiation, isolation (Psychosocial problems) and microgravity are the main health problems associated with human exploratory missions in outer space [36–38]. Whole body doses of 1–2 mSv per day accumulate in interplanetary space and about 0.5–1 mSv per day on the planetary surface. Effective doses for 6-months space station missions are about 0.08 Sv and could exceed 1 Sv for a Mars mission [39–41]. Different national space agencies have issued specific recommendations for accumulative dose limits for LEO astronauts, such as ISS crew members in order to prevent unacceptable deterministic effects for red blood cells-forming organs, bone marrow, spleen and lymphatic tissue. CSA, ESA and RFSA adopt a single career dose limit of 1 Sv for all genders and ages while NASA and JAXA apply different exposure limitation, summarized in Table 2. The Chinese Space Agency set 0.15 and 0.2 Sv skin dose limits for 3- and 7-days missions and a relatively low limit for 30-days missions, 0.4 Sv compared to 1.5 Sv adopted by ESA and RFSA.
Space radiation comprises galactic cosmic rays (GCR), solar particle events (SPE), and trapped belt radiation. GCR originate from outside of the solar system and consist of 2% electrons and 98% baryons, which in turn are composed of 87% proton, 12% alpha-particle and 1% of heavy ions with high energy and charge [43]. The energy spectrum of GCR peaks near 1,000 MeV/u. Space flights in low earth orbit, such as missions on space shuttles, are protected by geomagnetic field and solid shielding of the Earth [44]. Thick shielding cannot be regarded as a solution for the issue of radiation in space; the very high energy of cosmic rays and the severe mass constraints in space flight represent a serious hindrance of effective shielding [45]. Radiation in space is substantially different from earth; high energy and charge particles (HZE) dominate the exposure in deep space, whereas γ-rays and low energy alpha-particles are the major contributors on Earth. This difference causes high uncertainty on the estimated radiation health risk [46–48]. Major uncertainties include radiation quality factors, dose-rate modifiers, the transfer of risk from one population to another and uncertainties related to radiation quality dependence of tumor lethality and non-targeted effects [46–48]. Only a few sources of HZE particles are currently available in the world for experimental studies. Ground-based research into space radiation is necessary to improve the understanding of biological effects of densely ionizing heavy ions, which in turn has a useful impact in predicting and reducing health risks for exposed individuals [49].
Chromosomal aberrations in peripheral blood lymphocytes is an important biomarker in predicting space radiation risk, as it provides simultaneous information on dose, and it has been measured extensively in astronauts during the past 10 years. The main contribution of biomarkers to manned space exploration is in reducing risk uncertainties that are estimated to be between 200 and 400% [50]. Upper 95% confidence intervals for cancer fatality could exceed 20% when non-targeted effects are included in risk estimates [51, 52]. Several reports have been published on chromosomal rearrangements in human cells induced by accelerated particles and other types of HZE [49, 53] and further contribute to carcinogenic risk in astronauts [43]. Some investigators have provided clear evidence for development and progression of intestinal tumors [54], hepatocellular carcinoma [55] and lung cancer [56] in response to HZE exposure.
The radiation environment in space is complex and contains mixture of charged particles with a range of energy. It was reported that a low dose of proton protects cells against chromosomal damage induced by subsequent exposure to doses from 1 GeV/u iron ions [57]. This phenomenon is well-known in radiation biology literature as an adaptive response that is classically defined as the ability of low dose radiation exposure to partially ameliorate the effect of subsequent exposure to high challenge doses of radiation. This adaptive response is temporary and does not last for a long time, maximizing within a few hours of exposure and decaying within 48 h. Upregulation of DNA repair, antioxidant status and the immune system are the main contributors for this property. Unirradiated (bystander) cells with which the proton-irradiated cells were co-cultured were also significantly protected from the DNA-damaging effects of the challenge dose. These results show that the protective adaptive responses can spread from cells targeted by low-LET space radiation to bystander cells in their vicinity [58]. However, it is not clear if it will hold up for the lower space doses and dose-rates compared to experimental doses.
Upon traveling to deep space (interplanetary travels), beside HZE particles, astronaut's bodies would also be hit by secondary radiation including neutrons and recoil nuclei produced by nuclear reactions in spacecraft walls. Hu et al. [59] compared the biological effect of an iron beam with a shielded beam of the same average energy on cells in different cell cycle conditions. The conclusion that has been drawn from his study is that the biological effect of secondary particles should be examined for improved shielding design. Exposure of human and mice cells to simulated space radiation to measure the frequency of malignant transformation will aid in developing efficient countermeasures against space radiation-induced adverse effects. For example, Selenomethionine was shown to be a very promising countermeasure against HZE- induced cytotoxicity by enhancing DNA repair machinery in irradiated cells [60]. The oncogenic potential of cosmic rays is the main hindrance to interplanetary travel, ground-based research into space radiation plays a key role in reducing projected cancer risk uncertainty and development of physical (shielding) and biomedical (radioprotectors) countermeasures.
High Background Radiation Area
High natural radiation background areas (HBRA) have been of special interest as they provide opportunity for the study of biological effects of an environment that resembles the chronic exposure of future space colonists to doses of ionizing radiation of several orders of magnitude higher-than-normal levels [61]. Radionuclides, 232Th, 235U, 238U, and radioisotope of Potassium (40K), are the major sources of outdoor natural radiation. The knowledge of their distribution in soil, sand and rock plays an important role in protecting humans from serious health hazards.
Ramsar, Iran, due to the concentration of 226Ra and its daughters which were brought to the earth's surface by hot springs, also Kerala, in India [62], and certain beaches in Brazil [63], due to radioactive mineral-rich sand, all are examples of regions with higher level of natural radiation (Table 3).
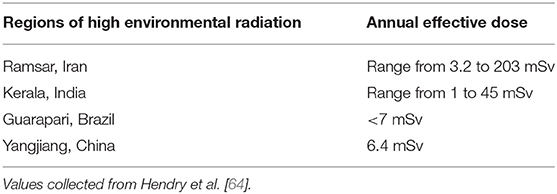
Table 3. Estimated annual effective doses to persons living in areas of high natural radiation background.
Guarapari region of Brazilian coast is a famous tourist attraction where thousands of people try to cure disease by lying on or cover themselves with black beach sand. Vasconcelos et al. [65] began to determine the reference level of this region using gamma spectrometry and compared their results with internationally accepted values. These authors observed that Areia preta beach in Guarapari has dose rate up to 87 μSv/h; the same dose rate that can be encountered in the 1 km vicinity of the Chernobyl power plant. Areia Preta may therefore has the highest background found in beaches in world, possibly due to activity concentration of 232Th. It has been suggested to get rid of dark-yellow to brown monazite from noxiously radioactive spot to minimize risk of radiation injury and keep the black sand as attraction for tourism; the activity concentrations found in the mainly monazitic (dark yellow) sand fraction are up to 1000 times higher than the normal soil values. High natural radiation environment of Guarapari stimulate researchers to warn visitors from potential health risks at staying longer.
The southwest coastal line of the Kerala state in India is one of such regions known to have elevated levels of background radioactivity mainly due to monazite sand available with a high abundance of thorium. Inhalation, external exposure and ingestion are three main pathways of nature radiation exposure to human beings. It was reported that the inhalation dose varies from 0.1 to 3.53 mSv/y and the inhalation dose imported by indoor radon and its progeny is >50% of the total radiation dose [62]. Even if chromosomal aberrations were seen in the lymphocytes of exposed persons, the carcinogenicity has still not been established. A cohort study conducted in this region, the southwest coastal area of Kerala, during 2006–2009 to assess the role of high level natural radiation (≥0.1 mSv/y) on congenital mental radiation and cleft lip/palate has shown that the prevailing high natural radiation exposure does not increase the risk of these malfunction [66]. However, its widely known that stable translocation aberration is associated with human malignancies; certain types of leukemia are examples of this. Therefore, recent data are necessary to confirm whether high background induced unnatural aberrations activate oncogenes.
On the same hand, Yangjiang in Guangdong province, China, is categorized as a high background radiation area. It was reported that the average annual effective dose to residents in HBRAs of Yangjiang was 6.2 mSv, about three times higher than that of the control area [67–69]. It was reported the annual dose received by the 0–7 age group was the highest among all age groups [70]. The individual cumulative dose to inhabitants living in houses built over 30 years ago was relatively low, compared with those living in houses built more recently [70]. However, this difference was not revealed in the control area. Yong-ling et al. [68] estimated that 88% of total amount of internal radiation dose to the residents in HBRA arose from the inhalation of 222Rn, 220Rn, and their products. An appropriate number of epidemiological studies were carried out to explore the cancer risk associated with low level radiation exposure [67, 70]. These studies did not find any statistically significant differences in all cancer mortality between control and high natural radiation area. Further, the relative cancer incidence risks of stomach, colon, liver, lung, bone, female breast and thyroid in Yangajiang were also not statistically different from the area with normal radiation levels. Thus, the typical level of natural radiation background in Yangjiang is insufficient to trigger a carcinogenesis risk increase in humans, and this conclusion may be partially owing to the enhanced immune function in the human body after long-term exposure in Yangjiang [69].
Nuclear Medicine and Radiotherapy
A double-edged sword is considered the best description for the status of ionizing radiation. It is harmful to health from its role as a carcinogen. However, it is beneficial for the use in both diagnostic and therapeutic medical application [71].
Radiotherapy is one of the most common and effective therapeutic modalities for the treatment of cancer. Usually 50% of all patients with localized malignant tumors are treated with radiation. Radiotherapy for cancer allows for the killing of the cancer cells but also presents a risk to the normal tissue surrounding the tumors and forming secondary malignant neoplasms at the same organ or at a distant part of the body. subsequent malignancies risk is the most significant late effect of radiation treatment experienced by cancer survivors [72]. Because of longer life expectancies, younger patients are certainly at greater risk [73, 74]. A large cohort study includes 5,798 Hodgkin's lymphoma patients treated with chemotherapy in Britain from 1963 to 2001—the majority of whom, 3,432, also received radiotherapy—has been conducted to assess secondary malignancy risks. Chemotherapy alone led to a raised risk of second cancer (RR, 2.0). However, this risk is lower and affects fewer anatomic sites than that of combined modalities (RR, 3.9) [74]. de Gonzalez et al. [75] performed a large-scale Surveillance, Epidemiology and End Results (SEER) analysis on cancer survivors who were treated with radiotherapy and documented a small increase in the risk of developing a second cancer. Other treated sites which have been investigated, including breast radiation treatment, again demonstrating the risk of second cancer development. The lungs and heart are likely to receive an amount of stray radiation during radiotherapy to breast cancers as they lie underneath the irradiated area. It has been reported that heart disease and lung cancer risks gradually increased after breast irradiation [76, 77]. Countermeasures are likely to be beneficial for cancer survivors after radiotherapy as they are capable of mitigating radiation-induced biological effects including damage of normal tissue surrounding tumors and radiation-induced secondary malignancies [78].
Many years ago, researchers proposed that accelerated proton and heavy ions could be used for localized cancer therapy based on their depth-dose distribution compared to photon radiation including X-ray and γ-rays [1]. Heavy ions are more effective than X-rays for killing cells as well as other endpoints, such as causing mutation [79]. Sethi et al. [80] published a retrospective review to see the incidence of second malignant neoplasms among retinoblastoma patients who received either photon (31 patients) or proton (55 patients) beams radiation. Cumulative incidence of second malignancies was significantly higher among the photon cohort (14% vs. 0; p = 0.015). Similarly, a retrospective study investigating the risk of secondary malignancies in prostate cancer patients found a lower risk with carbon ion radiotherapy (CIRT) compared to photon-based therapy [81], possibly due to the “Bragg peak” characteristic of particle therapy where low levels of energy are deposited outside of the target volume.
Radiation can induce apoptosis or trigger a DNA repair mechanism. In general, minor DNA damage is thought to temporarily halt the cell cycle to allow effective repair, while more severe damage can induce an apoptotic cell death program [82]. DNA is the quintessential target; the deleterious effects of radiation, mutation and carcinogenesis, are mainly due to irreparable damage to DNA. Wu et al. [83] provided evidence suggesting that extranuclear targets play a role in such damage. His data demonstrated that irradiation of cytoplasm produce gene mutation in nucleus through free radicals. His conclusion was that cytoplasmic traversal by ionizing radiation may be more dangerous than nuclear traversal, because the mutagenicity is accomplished by little or no killing of target cells. Radiation-induced carcinogenesis is a highly modifiable phenomenon by a non-carcinogenic process [84, 85]. The agents include the specific characteristics of the radiation, radiation type, dose rate, dose fractionation, dose distribution, etc., as well as many other contributing elements that are not specific to the radiation exposure, such as animal genetic characteristics, environment of the animal and animal age at exposure, as found from radiation-carcinogenesis studies in animals.
Conclusion
In this review, we provide discussion of the cancer risk that may arise following exposure to low dose ionizing radiation. Radiation-related cancer risk in the life span study (LSS) cohort of atomic bombs has been reported to continue raising throughout life. Significant dose response (ERR for all solid cancer) is observed even over 0–0.2 Gy dose range; supporting the hypothesis that there is no threshold below which cancers are not induced. Identification of non-cancer disease risks, psychological consequences of nuclear disaster for instance is one of several important steps to accomplish a comprehensive exposure outcome study. Million Worker Study (MWS) includes many subjects, 12 times higher than Japanese bomb survivors, as well as covering the issues faced today concerning exposures delivered over years, such as medical, occupational and environmental exposure. This large number of cases along with accurate individual exposure information will reduce uncertainty in the calculation of excess relative risk per Gray (ERR/Gy) and thus provide more reliable assessment of the long term effects of radiation exposure.
During low Earth orbit, shuttle crew members experience 90-min light-dark cycles. In addition, light intensity aboard ISS, space radiation, gravity and magnetic field also greatly differ from those on the ground. Numerous ground-based studies into the biological threats of these environmental stressors are needed to predict and reduce health risks for exposed individuals. Benefits from lunar mission and deep-space human exploration to Mars must be balanced between cost and the safety of astronauts.
Data collected so far suggest that particle therapy leads to a lower risk of secondary malignancies than conventional X-ray techniques. Moreover, ion beam therapy characterized by a low therapeutic dose to healthy tissue and a neutron production even lower than from photon therapy. Therefore, it provides a promising tumor treatment choice.
Author Contributions
GZ formed the work idea. YA literature collect and draft the article. FC and LN-A did the critical revision of the article. Eventually, FC, LN-A, and GZ gave their final approval of the version to be published. All authors contributed to the article and approved the submitted version.
Funding
This work was supported by the China National Natural Science Foundation awards (Nos. 11405235 and 81872622), National Key R&D Program of China (Nos. 2018YFC0115704, 2018YFC0115703, and 2018YFC0115705), China Postdoctoral Science Foundation (2018M632367), Jiangsu Postdoctoral Science Foundation (1701176B), and the Program of the Network-Type Joint Usage/Research Center for Radiation Disaster Medical Science.
Conflict of Interest
The authors declare that the research was conducted in the absence of any commercial or financial relationships that could be construed as a potential conflict of interest.
References
1. Hall EJ, Giaccia AJ. Radiobiology for the Radiologist. Philadelphia, PA: Lippincottt Williams & Wilkins (2012).
2. Imaoka T, Nishimura M, Iizuka D, Daino K, Takabatake T, Okamoto M, et al. Radiation-induced mammary carcinogenesis in rodent models: what's different from chemical carcinogenesis? J Radiat Res. (2009) 50:281–93. doi: 10.1269/jrr.09027
3. Abbott A. Researchers pin down risks of low-dose radiation. Nature. (2015) 523:17–8. doi: 10.1038/523017a
4. Tubiana M, Aurengo A, Averbeck D, Masse R. Recent reports on the effect of low doses of ionizing radiation and its dose–effect relationship. Radiat Environ Biophys. (2006) 44:245–51. doi: 10.1007/s00411-006-0032-9
5. Brenner DJ, Doll R, Goodhead DT, Hall EJ, Land CE, Little JB, et al. Cancer risks attributable to low doses of ionizing radiation: assessing what we really know. Proc Natl Acad Sci USA. (2003) 100:13761–6. doi: 10.1073/pnas.2235592100
6. Brenner DJ, Sachs RK. Brenner DJ. Estimating radiation-induced cancer risks at very low doses: rationale for using a linear no-threshold approach. Radiat Environ Biophys. (2006) 44:253–6. doi: 10.1007/s00411-006-0029-4
7. ICRP. Low-dose extrapolation of radiation-related cancer risk. ICRP Publication 99. Ann ICRP. (2005) 35:1–140. doi: 10.1016/j.icrp.2005.11.002
8. ICRP. The 2007 recommendations of the International Commission on Radiological Protection. ICRP Publication 103. Ann ICRP. (2007) 37:1–332. doi: 10.1016/j.icrp.2007.10.003
9. UNSCEAR. Sources, Effects Risks of Ionizing Radiation. Report to the General Assembly Scientific Annexes AB. UNSCEAR 2012 Report. United Nations Scientific Committee on the Effects of Atomic Radiation. United Nations sales publication E.16.IX.1. New York, NY: United Nations (2015).
10. UNSCEAR. Sources, Effects Risks of Ionizing Radiation. Report to the General Assembly Scientific Annexes AB. UNSCEAR 2017 report. United Nations Scientific Committee on the Effects of Atomic Radiation. New York, NY: United Nations (2018).
11. National Research Council. Health Risks from Exposure to Low Levels of Ionizing Radiation: BEIR VII Phase 2. Washington, DC: The National Academies Press (2006).
12. Tubiana M, Feinendegen LE, Yang C, Kaminski JM. The linear no-threshold relationship is inconsistent with radiation biologic and experimental data. Radiology. (2009) 251:13. doi: 10.1148/radiol.2511080671
13. Portess DI, Bauer G, Hill MA, O'Neill P. Low-dose irradiation of nontransformed cells stimulates the selective removal of precancerous cells via intercellular induction of apoptosis. Cancer Res. (2007) 67:1246–53. doi: 10.1158/0008-5472.CAN-06-2985
14. Mullenders L, Atkinson M, Paretzke H, Sabatier L, Bouffler S. Assessing cancer risks of low-dose radiation. Nat Rev Cancer. (2009) 9:596–604. doi: 10.1038/nrc2677
15. Seong KM, Kwon TW, Seo S, Lee D, Park S, Jin YW, et al. Perception of low dose radiation risks among radiation researchers in Korea. PLoS ONE. (2017) 12:e0171777. doi: 10.1371/journal.pone.0171777
16. Preston DL, Cullings H, Suyama A, Funamoto S, Nishi N, Soda M, et al. Solid cancer incidence in atomic bomb survivors exposed in utero or as young children. J Natl Cancer Inst. (2008) 100:428–36. doi: 10.1093/jnci/djn045
17. Preston DL, Shimizu Y, Pierce DA, Suyama A, Mabuchi K. Studies of mortality of atomic bomb survivors. Report 13: solid cancer and noncancer disease mortality: 1950–1997. Radiat Res. (2003) 160:381–407. doi: 10.1667/RR3049
18. Kamiya K, Ozasa K, Akiba S, Niwa O, Kodama K, Takamura N, et al. Long-term effects of radiation exposure on health. Lancet. (2015) 386:469–78. doi: 10.1016/S0140-6736(15)61167-9
19. Preston D, Ron E, Tokuoka S, Funamoto S, Nishi N, Soda M, et al. Solid cancer incidence in atomic bomb survivors: 1958–1998. Radiat Res. (2007) 168:1–64. doi: 10.1667/RR0763.1
20. Ozasa K, Cullings HM, Ohishi W, Hida A, Grant EJ. Epidemiological studies of atomic bomb radiation at the Radiation Effects Research Foundation. Int J Radiat Biol. (2019) 95:879–91. doi: 10.1080/09553002.2019.1569778
21. Yamashita S, Takamura N, Ohtsuru A, Suzuki S. Radiation exposure and thyroid cancer risk after the fukushima nuclear power plant accident in comparison with the chernobyl accident. Radiat Prot Dosimetry. (2016) 171:41. doi: 10.1093/rpd/ncw189
22. Hatch M, Cardis E. Somatic health effects of Chernobyl: 30 years on. Eur J Epidemiol. (2017) 32:1047–54. doi: 10.1007/s10654-017-0303-6
23. Fridman M, Lam AKY, Krasko O. Characteristics of young adults of Belarus with post-chernobyl papillary thyroid carcinoma: a long-term follow-up of patients with early exposure to radiation at the 30th anniversary of the accident. Clin Endocrinol. (2016) 85:971–8. doi: 10.1111/cen.13137
24. Tronko M, Brenner AV, Bogdanova T, Shpak V, Oliynyk V, Cahoon EK, et al. Thyroid neoplasia risk is increased nearly 30 years after the chernobyl accident. Int J Cancer. (2017) 141:1585. doi: 10.1002/ijc.30857
25. Kurttio P, Seppä K, Pasanen K, Patama T, Auvinen A, Pukkala E, et al. Fallout from the chernobyl accident and overall cancer incidence in Finland. Cancer Epidemiol. (2013) 37:585. doi: 10.1016/j.canep.2013.05.006
26. Alinaghizadeh H, Tondel M, Walinder R. Cancer incidence in northern Sweden before and after the Chernobyl nuclear power plant accident. Radiat Environ Biophys. (2014) 53:495–504. doi: 10.1007/s00411-014-0545-6
27. Alinaghizadeh H, Wålinder R, Vingård E, Tondel M. Total cancer incidence in relation to137Cs fallout in the most contaminated counties in Sweden after the Chernobyl nuclear power plant accident: a register-based study. BMJ Open. (2016) 6:e011924. doi: 10.1136/bmjopen-2016-011924
28. Till JE, Beck HL, Aanenson JW, Grogan HA, Mohler HJ, Mohler SS, et al. Military participants at US atmospheric nuclear weapons testing—methodology for estimating dose and uncertainty. Radiat Res. (2014) 181:471–84. doi: 10.1667/RR13597.1
29. Beck HL, Till JE, Grogan HA, Aanenson JW, Mohler HJ, Mohler SS, et al. Red bone marrow and male breast doses for a cohort of atomic veterans. Radiat Res. (2017) 187:221–8. doi: 10.1667/RR14458.1
30. Boice JD Jr, Ellis ED, Golden AP, Girardi DJ, Cohen SS, et al. The past informs the future: an overview of the million worker study and the Mallinckrodt chemical works cohort. Health Phys. (2018) 114:381–5. doi: 10.1097/HP.0000000000000825
31. UNSCEAR. Sources and Effects of Ionizing Radiation. Volume I, Report to the General Assembly, With Scientific Annexes A and B–Sources. United Nations Scientific Committee on the Effects of Atomic Radiation (2010).
32. Richardson DB, Cardis E, Daniels RD, Gillies M, O'Hagan JA, Hamra GB, et al. Risk of cancer from occupational exposure to ionising radiation: retrospective cohort study of workers in France, the United Kingdom, and the United States (INWORKS). BMJ. (2015) 351:h5359. doi: 10.1136/bmj.h5359
33. Leuraud K, Richardson DB, Cardis E, Daniels RD, Gillies M, O'hagan JA, et al. Ionising radiation and risk of death from leukaemia and lymphoma in radiation-monitored workers (INWORKS): an international cohort study. Lancet Haematol. (2015) 2:e276–81. doi: 10.1016/S2352-3026(15)00094-0
34. Thorne M. ICRP publication 60: 1990 recommendations of the international commission on radiological protection. Ann ICRP. (1991) 21:1–201. doi: 10.1016/0306-4549(92)90053-E
35. Pei W, Hu W, Chai Z, Zhou G. Current status of space radiobiological studies in China. Life Sci Space Res. (2019) 22:1–7. doi: 10.1016/j.lssr.2019.05.001
36. Durante M, Cucinotta FA. Heavy ion carcinogenesis and human space exploration. Nat Rev Cancer. (2008) 8:465. doi: 10.1038/nrc2391
37. Lei R, Zhou G, Hong MA, Zhuang F, Deng Y. Space life science of China in 2013. Chin J Space Sci. (2014) 34:747–56. doi: 10.11728/cjss2014.05.747
38. Xu D, Zhao X, Li Y, Ji Y, Zhang J, Wang J, et al. The combined effects of X-ray radiation and hindlimb suspension on bone loss. J Radiat Res. (2014) 55:720. doi: 10.1093/jrr/rru014
39. Cucinotta FA, Wu H, Shavers MR, George K. Radiation dosimetry and biophysical models of space radiation effects. Gravit Space Biol. (2003) 16:11–8.
40. Cucinotta FA, Kim M-HY, Willingham V, George KA. Physical and biological organ dosimetry analysis for International Space Station Astronauts. Radiat Res. (2008) 170:127–38. doi: 10.1667/RR1330.1
41. Kim M-HY, Cucinotta FA, Nounu HN, Zeitlin C, Hassler DM, Rafkin SCR, et al. Comparison of Martian surface ionizing radiation measurements from MSL-RAD with Badhwar-O'Neill 2011/HZETRN model calculations. J Geophys Res Planets. (2014) 119:1311–21. doi: 10.1002/2013JE004549
42. McKenna-Lawlor S. Feasibility study of astronaut standardized career dose limits in LEO the outlook for BLEO. Acta Astron. (2014) 104:565–73. doi: 10.1016/j.actaastro.2014.07.011
43. Zhou Z, Ware JH, Kennedy AR. Carbon and iron ion radiation-induced cytotoxicity and transformation in vitro. Oncol Lett. (2011) 2:915–8. doi: 10.3892/ol.2011.342
44. Cucinotta FA, Durante M. Cancer risk from exposure to galactic cosmic rays: implications for space exploration by human beings. Lancet Oncol. (2006) 7:431. doi: 10.1016/S1470-2045(06)70695-7
45. Durante M, Cucinotta FA. Physical basis of radiation protection in space travel. Rev Mod Phys. (2011) 83:1245–81. doi: 10.1103/RevModPhys.83.1245
46. Cucinotta FA. A new approach to reduce uncertainties in space radiation cancer risk predictions. PLoS ONE. (2015) 10:e0120717. doi: 10.1371/journal.pone.0120717
47. Cucinotta FA. Space radiation risks for astronauts on multiple International Space Station missions. PLoS ONE. (2014) 9:e96099. doi: 10.1371/journal.pone.0096099
48. Cucinotta FA, To K, Cacao E. Predictions of space radiation fatality risk for exploration missions. Life Sci Space Res. (2017) 13:1–11. doi: 10.1016/j.lssr.2017.01.005
49. George K, Durante M, Willingham V, Wu H, Yang TC, Cucinotta FA. Biological effectiveness of accelerated particles for the induction of chromosome damage measured in metaphase and interphase human lymphocytes. Radiat Res. (2003) 160:425–35. doi: 10.1667/RR3064
50. Durante M. Biomarkers of space radiation risk. Radiat Res. (2005) 164:467–73. doi: 10.1667/RR3359.1
51. Cucinotta FA, Cacao E. Non-targeted effects models predict significantly higher mars mission cancer risk than targeted effects models. Sci Rep. (2017) 7:1832. doi: 10.1038/s41598-017-02087-3
52. Cucinotta FA, Cacao E, Kim MY, Saganti PB. Cancer and circulatory disease risks for a human mission to mars: private mission considerations. Acta Astron. (2018) 166:529–36. doi: 10.1016/j.actaastro.2018.08.022
53. Hada M, Meador JA, Cucinotta FA, Gonda SR, Wu H. Chromosome aberrations induced by dual exposure of protons and iron ions. Radiat Environ Biophys. (2007) 46:125–9. doi: 10.1007/s00411-006-0083-y
54. Trani D, Datta K, Doiron K, Kallakury B, Fornace AJ. Enhanced intestinal tumor multiplicity and grade in vivo after HZE exposure: mouse models for space radiation risk estimates. Radiat Environ Biophys. (2010) 49:389–96. doi: 10.1007/s00411-010-0292-2
55. Weil MM, Ray FA, Genik PC, Yu Y, Mccarthy M, Fallgren CM, et al. Effects of 28Si ions, 56Fe ions, and protons on the induction of murine acute myeloid leukemia and hepatocellular carcinoma. PLoS ONE. (2014) 9:e104819. doi: 10.1371/journal.pone.0104819
56. Ding LH, Park S, Xie Y, Girard L, Minna JD, Story MD. Elucidation of changes in molecular signalling leading to increased cellular transformation in oncogenically progressed human bronchial epithelial cells exposed to radiations of increasing LET. Mutagenesis. (2015) 30:685–94. doi: 10.1093/mutage/gev028
57. Elmore E, Lao XY, Kapadia R, Swete M, Redpath JL. Neoplastic transformation in vitro by mixed beams of high-energy iron ions and protons. Radiat Res. (2011) 176:291–302. doi: 10.1667/RR2646.1
58. Buonanno M, Toledo SMD, Howell RW, Azzam EI. Low-dose energetic protons induce adaptive and bystander effects that protect human cells against DNA damage caused by a subsequent exposure to energetic iron ions. J Radiat Res. (2015) 56:502–8. doi: 10.1093/jrr/rrv005
59. Hu W, Pei H, Li H, Ding N, He J, Wang J, et al. Effects of shielding on the induction of 53BP1 foci and micronuclei after Fe ion exposures. J Radiat Res. (2013) 55:10–6. doi: 10.1093/jrr/rrt078
60. Kennedy AR, Ware JH, Guan J, Donahue JJ, Biaglow JE, Zhou Z, et al. Selenomethionine protects against adverse biological effects induced by space radiation. Free Radic Biol Med. (2004) 36:259–66. doi: 10.1016/j.freeradbiomed.2003.10.010
61. Durante M, Manti L. Human response to high-background radiation environments on earth and in space. Adv Space Res. (2008) 42:999–1007. doi: 10.1016/j.asr.2007.02.014
62. Ben Byju S, Koya P, Sahoo B, Jojo P, Chougaonkar M, Mayya Y. Inhalation and external doses in coastal villages of high background radiation area in Kollam, India. Radiat Protec Dosimetry. (2012) 152:154–8. doi: 10.1093/rpd/ncs213
63. Costa-de-Moura J, Wallfass CM, Bossew P. Health hazards of radioactive sands along the Coast of Espírito Santo/Brazil. N. Jb. Geol. Paläont. Abh. (2002) 225:127–36. doi: 10.1127/njgpa/225/2002/127
64. Hendry JH, Simon SL, Wojcik A, Sohrabi M, Burkart W, Cardis E, et al. Human exposure to high natural background radiation: what can it teach us about radiation risks? J Radiol Prot. (2009) 29:A29–42. doi: 10.1088/0952-4746/29/2A/S03
65. Vasconcelos DC, Reis PAL, Pereira C, Oliveira AH, Santos TO, Rocha Z. Modelling natural radioactivity in sand beaches of Guarapari, Espírito Santo State, Brazil. World J Nucl Sci Technol. (2013) 3:65–71. doi: 10.4236/wjnst.2013.32011
66. Koya PK, Chougaonkar MP, Predeep P, Jojo PJ, Cheriyan VD, Mayya YS, et al. Effect of low and chronic radiation exposure: a case-control study of mental retardation and cleft lip/palate in the monazite-bearing coastal areas of southern Kerala. Radiat Res. (2012) 177:109–16. doi: 10.1667/RR2699.1
67. Tao Z, Zha Y, Akiba S, Sun Q, Zou J, Li J, et al. Cancer mortality in the high background radiation areas of Yangjiang, China during the period between 1979 and 1995. J Radiat Res. (2000) 41:31. doi: 10.1269/jrr.41.S31
68. Yuan YL, Morishima H, Shen H. The estimation of doses to the inhabitants arising from natural radiation source in the high background radiation area of Yangjiang, China. Chin J Radiol Health. (2004) 13:1–7. Available online at: https://inis.iaea.org/search/search.aspx?orig_q=RN:36029092
69. Zou JM. Major results of the study on cancer mortality and its related factors among inhabitants in the high background radiation area (HBRA) of Yangjiang, Guangdong. Chin Occup Med. (2004) 3. Available online at: http://www.en.cnki.com.cn/Article_en/CJFDTOTAL-XYYX200403001.htm
70. Yuan Y, Shen H, Changsha Zhao S. Recent advances of dosimetry investigation in the high background radiation area in Yangjiang, China. Chin J Rodiat Mediat Protec. (1995) 6:77–100.
71. Christensen R, Alsner J, Brandt SF, Dagnaeshansen F, Kolvraa S, Serakinci N. Transformation of human mesenchymal stem cells in radiation carcinogenesis: long-term effect of ionizing radiation. Regen Med. (2008) 3:849–61. doi: 10.2217/17460751.3.6.849
72. Newhauser WD, Durante M. Assessing the risk of second malignancies after modern radiotherapy. Nat Rev Cancer. (2011) 11:438–48. doi: 10.1038/nrc3069
73. Jin F, Luo H-L, Zhou J, He Y-N, Liu X-F, Zhong M-S, et al. Cancer risk assessment in modern radiotherapy workflow with medical big data. Cancer Manag Res. (2018) 10:1665–75. doi: 10.2147/CMAR.S164980
74. Swerdlow AJ, Higgins CD, Smith P, Cunningham D, Hancock BW, Horwich A, et al. Second cancer risk after chemotherapy for Hodgkin's lymphoma: a collaborative British cohort study. J Clin Oncol. (2011) 29:4096–104. doi: 10.1200/JCO.2011.34.8268
75. de Gonzalez AB, Curtis RE, Kry SF, Gilbert E, Lamart S, Berg CD, et al. Proportion of second cancers attributable to radiotherapy treatment in adults: a cohort study in the US SEER cancer registries. Lancet Oncol. (2011) 12:353–60. doi: 10.1016/S1470-2045(11)70061-4
76. Dracham CB, Shankar A, Madan R. Radiation induced secondary malignancies: a review article. Radiat Oncol J. (2018) 36:85–94. doi: 10.3857/roj.2018.00290
77. Henson KE, McGale P, Taylor C, Darby SC. Radiation-related mortality from heart disease and lung cancer more than 20 years after radiotherapy for breast cancer. Br J Cancer. (2013) 108:179–82. doi: 10.1038/bjc.2012.575
78. Ware J, Zhaozong Zhou, Romero-Weaver A, Stevenwan X, Newberne P, Kennedy A. Effects of selenomethionine in irradiated human thyroid epithelial cells and tumorigenicity studies. Nutr Cancer Int J. (2011) 63:1114–21. doi: 10.1080/01635581.2011.605981
79. Durante M, Loeffler JS. Charged particles in radiation oncology. Nat Rev Clin Oncol. (2010) 7:37. doi: 10.1038/nrclinonc.2009.183
80. Sethi RV, Shih HA, Yeap BY, Mouw KW, Petersen R, Kim DY, et al. Second nonocular tumors among survivors of retinoblastoma treated with contemporary photon and proton radiotherapy. Cancer. (2014) 120:126–33. doi: 10.1002/cncr.28387
81. Mohamad O, Tabuchi T, Nitta Y, Nomoto A, Sato A, Kasuya G, et al. Risk of subsequent primary cancers after carbon ion radiotherapy, photon radiotherapy, or surgery for localised prostate cancer: a propensity score-weighted, retrospective, cohort study. Lancet Oncol. (2019) 20:674–85. doi: 10.1016/S1470-2045(18)30931-8
82. Baskar R. Emerging role of radiation induced bystander effects: cell communications and carcinogenesis. Genome Integr. (2010) 1:13. doi: 10.1186/2041-9414-1-13
83. Wu LJ, Randerspehrson G, Xu A, Waldren CA, Geard CR, Yu Z, et al. Targeted cytoplasmic irradiation with alpha particles induces mutations in mammalian cells. Proc Natl Acad Sci USA. (1999) 96:4959–64. doi: 10.1073/pnas.96.9.4959
84. Kennedy AR. Factors that modify radiation-induced carcinogenesis. Health Phys. (2009) 97:433–45. doi: 10.1097/HP.0b013e3181ac9262
Keywords: low dose exposure, HBRL inhabitants, space exploration missions, nuclear industry workers, cancer risk
Citation: Ali YF, Cucinotta FA, Ning-Ang L and Zhou G (2020) Cancer Risk of Low Dose Ionizing Radiation. Front. Phys. 8:234. doi: 10.3389/fphy.2020.00234
Received: 03 February 2020; Accepted: 28 May 2020;
Published: 12 August 2020.
Edited by:
Vincenzo Patera, Sapienza University of Rome, ItalyReviewed by:
Dimitris Emfietzoglou, University of Ioannina, GreeceMarco Durante, GSI Helmholtz Center for Heavy Ion Research, Germany
Copyright © 2020 Ali, Cucinotta, Ning-Ang and Zhou. This is an open-access article distributed under the terms of the Creative Commons Attribution License (CC BY). The use, distribution or reproduction in other forums is permitted, provided the original author(s) and the copyright owner(s) are credited and that the original publication in this journal is cited, in accordance with accepted academic practice. No use, distribution or reproduction is permitted which does not comply with these terms.
*Correspondence: Guangming Zhou, Z216aG91QHN1ZGEuZWR1LmNu