- Molecular and Preclinical Imaging Centers, Department of Molecular Biotechnology and Health Sciences, University of Torino, Torino, Italy
One of the most challenging tasks of the cancer research is the enhancement of the amount of the chemotherapeutic agent that can reach the target site. To achieve this goal, nanovectors capable of encapsulating the drug and releasing it following a specific stimulus have been developed. In light of this, a key point is the necessity to monitor the effective drug release through a safe and highly performing imaging technology such as Magnetic Resonance Imaging (MRI). Liposomes are highly biocompatible nanovesicles that consist of bilayered phospholipid-based membrane encompassing an aqueous core. Almost 20 drug-loaded liposomes are currently approved for clinical use in USA and EU countries. If a liposomal nanomedicine is loaded with an MRI agent whose contrast is sensitive to the microenvironment and with a release kinetics similar to the co-transported drug, the system can act as an imaging reporter of drug release. This Perspective will offer a critical and brief overview of using MRI not only to verify and monitor the release process but also as a valuable tool to predict the therapeutic outcome. In particular, it will be presented representatives preclinical studies illustrating the in vivo potential of MRI-guided drug release protocols triggered by thermal and mechanical ultrasound-induced effects. Considering the therapeutic advantages of this approach, the possible benefits in reducing the side effects and the good results reported at preclinical level, there is a reasonable hope that the near future could witness the entrance in clinical routine of MRI-guided procedures supporting ultrasound-induced drug release protocols.
Introduction
Despite the increased understanding of the biological mechanisms underlying tumorigenesis and the latest developments in medical technologies, cancer is still the leading cause of death in wealthy countries [1]. Among the available treatments, chemotherapy is a valuable option, especially when surgery is not accessible. Chemotherapy is generally based on the systemic administration of an anticancer drug, which normally reaches the malignant lesion only in a limited amount. In fact, most of the injected drug is taken up by healthy tissues/organs, especially those with an accelerated metabolism, thus causing undesirable side effects [2]. Therefore, it is of paramount importance to search for innovative and improved therapeutic approaches capable of increasing the drug accumulation in the tumor and reducing the drug distribution in the healthy body districts. One of the most common strategies to achieve this task is to vehiculate the drug with a drug delivery system, typically a nanocarrier [3]. The resulting nanomedicine may accumulate in the tumor primarily by the Enhanced Permeability and Retention (EPR) effect [4], though active trans-endocytosis has been recently demonstrated the dominant mechanism involved in the nanoparticles tumor uptake. [5] However, once the nanomedicine has reached the target, the therapeutic efficiency of the drug is strongly affected by the release of the medicine from its carrier that, if not stimulated, occurs slowly with mechanisms and kinetics not always fully understood yet.
Among in vivo imaging technologies, Magnetic Resonance Imaging (MRI) represents a powerful tool to assess in real-time, low invasiveness, and great spatio-temporal resolution the biodistribution of a nanomedicine and the release of its payload. Specifically, the typical strategy for imaging drug release is to co-load the nanocarrier with the drug and an imaging probe, whose contrast is sensitive to the microenvironment changes associated with the release.
Due to the difficulty to make microbubbles, a widely used platform for ultrasound (US)-induced drug release, MRI visible, this Perspective offers a critical and brief overview of the use of MRI as imaging technology of election for the in vivo visualization of the release of drugs from nanoliposomes stimulated by US. It will come out that the advantages of using in vivo imaging technologies to support stimulated drug release are not only limited to verify and monitor the release process, but they may be extended to the prediction of the therapeutic outcome (Figure 1).
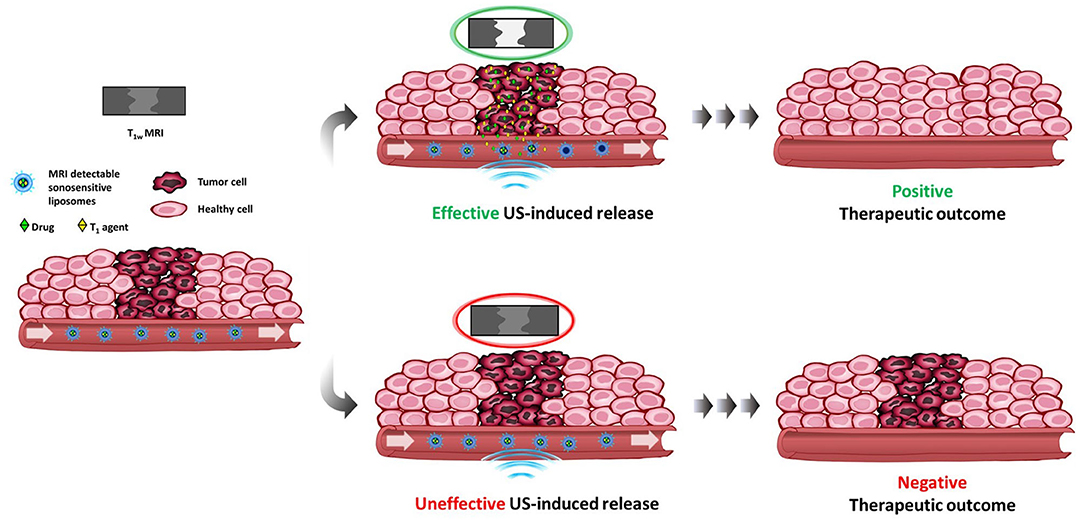
Figure 1. Schematic view of a theranostic protocol using MRI-detectable sonosensitive chemotherapeutic liposomes. If effective, the ultrasonic stimulus applied on the tumor triggers the release of both drug and MRI T1 agent with consequent contrast enhancement and positive therapeutic outcome. The lacking of contrast enhancement gives an immediate information on the ineffectiveness of the stimulus, which would result in a negative outcome, thus allowing to find a remediation (repeat the stimulation, change therapeutic protocol, …).
Despite the extremely high number of nanocarriers studied as drug-delivery systems, only very few entered in clinical trials and even less reached the market. Among them, liposomes certainly have a prominent role with the first product (AmBisome®) approved 30 years ago [6]. Presently, none nanocarrier designed for imaging drug delivery/release has been tested on humans. However, by considering the high number of preclinical studies reported in literature, it is highly probable that the first system (if any) that will enter in clinical trials will be based on liposomes.
Liposomes (typical diameter 80–200 nm) consist of bilayered phospholipid-based membrane encompassing an aqueous core. They can easily load hydrophilic therapeutics in the inner aqueous compartment (e.g., doxorubicin hydrochloride) or hydrophobic drug in the bilayered membrane (e.g., paclitaxel). Their surface can expose polyethylene glycol (PEG) moieties to improve the biocompatibility and prolong blood circulation time (“stealth effect”). Moreover, their surface can be also functionalized with targeting ligands that can improve the accumulation of the transported drug at the diseased site. The great versatility displayed by liposomes allows the (co)-loading of a wide array of chemicals with different physico-chemical properties including contrast agents for any kind of imaging technologies [7].
The most adopted strategy for designing a MRI-detectable liposome able to act as drug release reporter is the encapsulation of a high amount (hundreds of millimolar) of a MRI-T1 agent (e.g., a Gd(III) complex) in the core of a liposome having a low water-permeable bilayer. These conditions make the nanocarrier “MRI silent,” because the intraliposomal water protons fully relax before diffusing out through the membrane. Hence, the release of the T1 agent from the nanocarrier removes the quenching effect operated by the membrane, shortens the T1 of the sample and produces a signal enhancement in MRI T1-weighted images proportional to the release of the liposomal content [8, 9]. If the release kinetics of the T1-agent is similar to that one of the co-transported drug, then the liposome can be considered a good reporter of drug release.
MRI-Guided Drug Release Triggered by Ultrasound Stimulation
MRI-guided high-intensity focused ultrasound (MRgFUS) is an emerging non-invasive technological platform clinically used for therapeutic purposes. The beneficial effect is mediated by the heating induced by FUS that can precisely kill the damaged cells with minimal effects on healthy ones.
However, acoustic waves can also represent a trigger for inducing the release of a drug from a nanocarrier. This event may occur through two main mechanisms (even concomitant): one mediated by heating and the other involving mechanical effects.
Besides the MRI scanner and the US apparatus, which can be integrated or not in the scanner, the whole procedure is completed by a tool for controlling the temperature (for heating-induced release) and by administration of the stimulus-sensitive, MRI detectable, drug delivery system.
Sonosensitive MRI Detectable Liposomes
As reported above, the main effects generated by the interaction of USwith nanocarriers are of thermal or mechanical nature. This differentiation is relevant, because the design of the drug delivery nanosystem has to be tailored on the effect that mostly contribute to the release mechanism. The following sections will offer some representative examples of MRI-detectable nanosystems specifically designed for imaging the drug release triggered by US stimulation.
Thermal Effects: Thermosensitive Liposomes (TSLs)
Thermosensitive nanovectors should meet some general criteria: the drug loading has to be very stable at temperatures lower than the release temperature where the release should be instead sharp, fast, and quantitative [10].
The first example of thermosensitive liposomes was published by Yatvin and Weinstestein more than 40 years ago [11]. The membrane of these liposomes was composed by di-palmitoyl-phosphatidyl-choline (DPPC) with a gel-to-liquid crystalline phase transition temperature (Tm) of 41°C. This means that at this temperature the permeability of the bilayer enhances significantly due to grain boundaries between gel and liquid crystalline domains, thus allowing the release of hydrophilic solutes encapsulated in the inner aqueous core. Since then, many TSLs loaded with different drugs as doxorubicin [12], cisplatin [13], methotrexate [14], and paclitaxel [15] were developed. Among them, the most relevant example is certainly the thermosensitive liposomal formulation transporting doxorubicin and marketed as Thermodox® that is currently under investigations in Phase III clinical trials in combination with radiofrequency ablation, and in Phase I trials in combination with HIFU treatment [16]. The Tm value of this formulation is slightly lower than the traditional TSLs (39–41°C vs. 42–45°C) and more compatible with hyperthermia therapeutic protocols. The Tm values of TSLs can be modulated through a proper selection of the bilayer components. Furthermore, it has been demonstrated that the presence in the bilayer of small amount of a lysolipid (e.g., monostearoylphosphatidylcholine (MSPC) in Thermodox®) ensures a very fast release at Tm without compromising the stability of the nanocarrier at physiological temperature.
The first MRI-detectable TSL was developed by Viglianti et al. who prepared a liposomes incapsulating the drug doxorubicin and paramagnetic Mn(II) ions as T1 agents. As the release of the paramagnetic ions produces a T1-contrast enhancement, the system was preclinically validated on a murine tumor model showing the good potential of MRI to report about the intratumor release of doxorubicin [17]. A similar approach, but replacing Mn(II) with the clinically approved (and therefore safer) T1 agent gadoteridol (the active principle of ProHance®), was developed by De Smet et al. After having successfully demonstrated the potential of the Gd-loaded TSL in vitro [18], the same research team administered the Gd- and doxorubicin-loaded TSLs injected in a rat model of gliosarcoma [19]. The results obtained nicely demonstrated the good correlation between the MRI T1-contrast and the amount of the released drug in the lesion, thus allowing the assessment of the efficacy of the HIFU stimulation and the prediction of the therapeutic outcome on a personalized basis.
The same research group also published and interesting comparative study between TSLs loaded with Mn(II) ions or gadoteridol [20]. It was observed that the encapsulation of Mn(II) ions greatly enhanced the contrast response to the release, but, at the same time, it favored the release of doxorubicin (as Mn(II) complex) already at temperatures lower than Tm (60% at 37°C). Furthermore, the Mn-loaded liposomes showed a slower release kinetics of the drug (if compared to the gadoteridol-loaded TSLs) at the phase transition temperature. The high stability of gadoteridol-loaded thermosensitive liposomes, coupled to the very sharp and fast release at the target temperature, make this formulation very suitable for in vivo translation, as it has been further demonstrated in a study where this probe was used for testing the performance of different and combined therapeutic thermal treatments (hyperthermia and ablation) delivered in conjunction with the doxorubicin-(and gadoteridol) loaded TSLs in a rhabdomyosarcoma tumor model on rats [21].
An alternative to the MRI-detectable thermosensitive liposome based on T1-agents was proposed by Langereis et al. who designed a LipoCEST probe encapsulating a fluorine-rich small compound () for dual CEST/19F MR detection [22]. At temperature values lower than Tm the dual probe can be detected by CEST contrast only because the 19F signal is unobservable due to the signal broadening effect exerted by the paramagnetic shift reagent to the co-encapsulated . When Tm is reached, the release of the liposomal content removes the broadening effect and, consequently, the appearance of the 19F signal indicates the occurrence of the release. At the same time, the release nullifies the CEST contrast, and, therefore, the combined evaluation between 19F and CEST detection may give an indication about the extent of the release. Though this system was only validated in vitro and without a loaded drug, it has the potential advantage to be compatible with MRI tools (e.g., PRFS) to monitor the temperature rise [23]. Another approach to monitor local drug delivery/release by MRI was presented by De Senneville et al. [24], who formulated thermosensitive liposomes incapsulating iron oxide nanoparticles (the so-called magnetoliposomes) that can potentially act as T1 or T2/ MRI agents. The release triggered by FUS-induced heating led to a big decrease in the /r1 ratio originated by the release of the iron-based nanoparticles that reduces the efficiency of the relaxation mechanism and, contextually, causes a T1 shortening. Hence, this is another case of a single agent able to monitor either the delivery of the drug (by T2/ contrast) or the release process (by /r1 ratio). The performance of this probe, without loaded drug, was only tested in vitro by dynamic MRI.
Mechanical Effects: Sonosensitive Liposomes
Besides harnessing the thermal effect, US can also trigger drug release by mechanic stimulation. This mechanism is facilitated by using low intensity (≤ 5 W/cm2) US, reducing the duty cycle of the insonation scheme, and using intermediate US frequencies (1–3 MHz) to reduce the risk of cavitation effects. Low intensity US can be focused or unfocused, with the former that are utilized for stimulating drug release (generally co-administrating microbubbles) and/or switch on the contrast in US imaging using perfluorocarbons-based nanodroplets [25, 26]. Liposomes are the most investigated nanocarriers using unfocused stimulation. Moreover, it is noteworthy that TSLs can also be sensitive to US-induced mechanical effects, though in this latter case, the release may occur at physiological temperature, i.e., lower than Tm, and heating is not necessary [27]. The precise mechanism through which the liposome bilayer allows the release of the transported cargo under the US-induced mechanical stimulation is not fully understood yet.
However, it is likely that the release may occur through the formation of pores in the liposome membrane as a consequence of US-induced effects like shearing/radiation forces or stable cavitation (though this requires the co-presence of microbubbles) [28].
In any case, there is a general consensus that the physical characteristics of the bilayer play a prominent role. One of the factors involved is the packing parameter (PP) of the bilayer components. PP is the ratio between the section area of the hydrophobic portion over the area of the polar head and determines the molecular shape of the phospholipid. Conventional liposomes contain DPPC and DSPC phospholipids that have a PP-value around 1 and display a natural tendency to stabilize bilayers. However, other phospholipids are typically added in the formulation, especially those conjugated with PEG chains in virtue of their capability to make the nanoparticles stealth toward the immune system. DSPE-PEG and DOPE-PEG have pegylated moieties conjugated to the polar head of the molecule, thus lowering the PP-value. The presence of PEG chains on the liposomes surface may have contradictory effects. Lin and Thomas showed that liposomes containing DSPE-PEG are more prone to release their cargo under ultrasonic stimulation [29], whereas Giustetto et al. observed an opposite behavior [27]. However, it has been reported that liposomes formulated with phospholipids with PP-values higher than one, such as DSPE or DOPE, where the weight of the headgroups is small compared to the bulky hydrocarbon chains, display an enhanced release under US exposure due to the destabilization effect of the membrane induced by the inverted cone shape of the phospholipids [30]. The importance of the membrane composition was also highlighted by Kang et al. who have hypothesized that the release may occur through the formation of small pores in the membrane following the US-induced switch from the lamellar phase to the inverse hexagonal phase of the bilayer [31]. In a different study, Schroeder et al. proposed that the US-induced release involves the transient formation of “pore-like defects” in the liposomes membrane that are caused by reversible effects of the cavitation microstreaming that characterizes non-thermal US [32].
Differently from thermal stimulation, it has been observed that the release induced by sonomechanical stimulus is also dependent on liposomes shape and composition of the inner aqueous core [26].
One of the first studies illustrating the therapeutic advantages of using a mechanical sonostimulation for triggering drug release from liposomes has been reported in 2009 by Schroeder et al. who observed a stop in the progression of a colon adenocarcinoma grafted in mice after systemic administration of liposomes loaded with cisplatin and successive (24 h later) exposure of the lesion to low intensity US [33].
The use of in vivo MRI for guiding the release of the liposomal cargo after a sonomechanical stimulus was first reported by Rizzitelli et al. [34]. Using a liposome loaded with the T1 agent gadoteridol only, the authors observed a significant enhancement of the tumor MRI contrast following the injection of the nanocarrier in a syngeneic breast cancer murine model and immediate local application of the sonomechanical stimulus (intensity 5.3 W/cm2). It was observed that the combination of different type of US stimulus (e.g., release stimulus + sonoporation stimulus [35]) led to a further improvement in the therapeutic performance with a total regression of the tumor after 3 weeks of treatment (Figure 2) [36]. Finally, the MRI guidance allowed to predict the long-term therapeutic outcome on the basis of the imaging response of the first treatment.
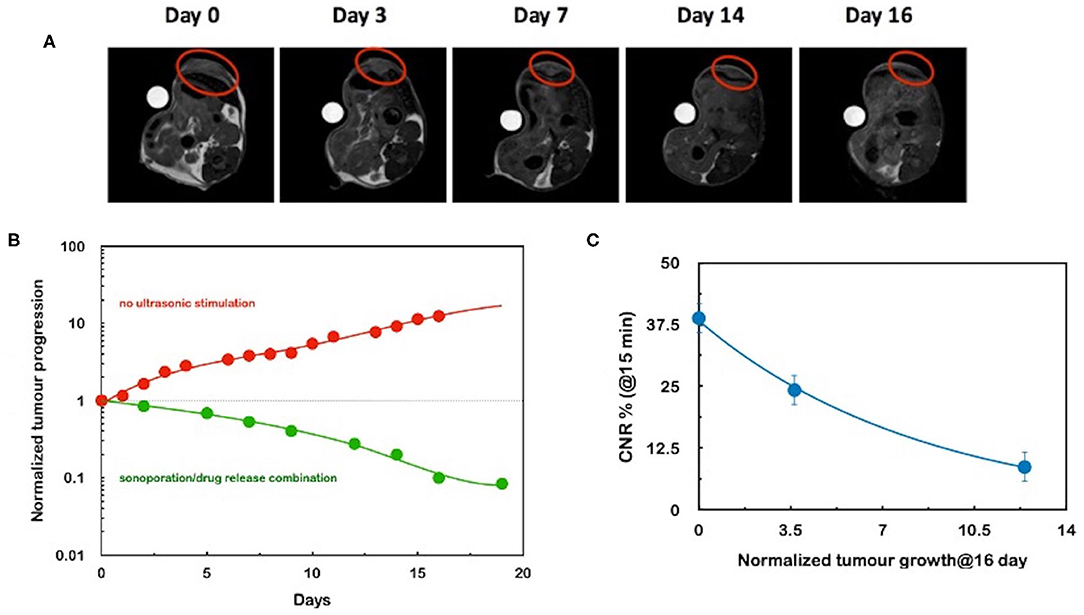
Figure 2. (A) series of morphological MR images acquired at different days after the administration of sonosensitive gadoteridol- and doxorubicin-loaded MRI-detectable liposomes. The red circle indicates the tumor. The stimulation of the tumor with a combination of acoustic waves for inducing sonoporation and triggering drug release led to an almost full regression of the lesion. Lines represent an interpolation of the data for eye-guiding purposes. (B) Time evolution of the tumor volume, normalized to the value at day 0. It is noteworthy that the red data refer to the nanomedicine very close to the clinically approved formulation. (C) Correlation between the MRI T1 contrast-to-noise ratio (CNR%) measured just after the first US stimulation at day 1 and the normalized tumor volume at day 16 for three experiments. The line is an interpolation of the data for eye-guiding purposes. This plot represents the experimental counterpart of the scheme illustrated in Figure 1. Adapted from ref. [31].
Conclusive Remarks
Most likely, Thermodox® will be the first “smart” nanomedicines, i.e., a nanocarrier where the drug is released reacting to an externally applied stimulus (i.e., heating), to be approved for clinical use.
The therapeutic advantages of this approach are unquestionable. The opportunity to release a large fraction of the drug specifically in the pathological district and shortly after the injection of the nanomedicine, while keeping the benefits in reducing the side effects of the drug due to the encapsulation of the drug in the nanocarrier, makes this approach extremely appealing and promising.
When the nanomedicine to make smart is very similar to an already approved formulation, as in the case of Doxil®/Thermodox® case, the approval is mostly conditioned by the technology necessary for triggering drug release and the modality of its application. In this regard, US stimulation is highly compatible with the clinical setting, being successfully used since a long time. Furthermore, as it has been described above, the interaction between US and matter is so complex that different release mechanisms based on thermal or mechanical (or both) effects can be harnessed. Dealing with liposomal nanocarriers, particularly interesting are the experimental observations that the US-induced release may be dependent on the particle size, shape, and composition of membrane and aqueous core. All these properties may offer valuable opportunities in the design of combination therapies with selective US-triggered drug release.
Of course, also the properties of both the acoustic waves and the transducer need to be optimized according to the characteristics of the pathology (especially its spatial location) in order to maximize the therapeutic performance of the protocol.
A crucial point in all the procedures based on the external application of a triggering stimulus is represented by the selection of the best timing for the stimulation. The US stimulus can be applied immediately after the injection, and in this case the release is defined intravascular, or it can be applied at a time in which the liposomes are accumulated in the tumor stroma, and in this case the release is called intratumor. Gasselhuber et al. analyzed these different types of release using a mathematical model that compared two thermosensitive liposomes with a fast (intravascular) and a slow (intratumor) release kinetics, respectively [37]. The intravascular release approach showed a better efficiency than the intratumor one, though both of them showed a similar therapeutic response.
Other important challenges that needs to be faced for the clinical translation of these protocols are intrinsically related to the US stimulation, which may suffer of poor reproducibility (with the consequent variable drug release efficiency) and limited application to the body regions.
Prospectively, the design of sonosensitive liposomes decorated with targeting vectors for endothelial tumor biomarkers should further improve the overall performance of the therapeutic protocol owing to the increase in the number of nanocarriers in the region exposed to the release stimulus.
It is also important to highlight that another advantage of stimulated drug release procedures based on US stimulation is the possibility to combine different types of acoustic waves in order to improve the release efficiency and the diffusion of the drug in the diseased region.
In this scenario, the opportunity to add in vivo imaging-detectability to the already smart nanomedicines and aimed at monitoring the effective drug release is of paramount importance.
Among the clinically available imaging technologies, MRI is certainly the best choice in virtue of the peculiar ability of some classes of MRI probes, especially the T1 agents, to generate an imaging response that can be made sensitive to the release of a drug from a nanocarrier.
MRI is also an excellent technique for monitoring temperature in vivo, and this property is clinically exploited to control HIFU-based protocols [38]. However, the fact that temperature is not linearly correlated to the extent of drug release, and the observation that there is no heating in the mechanical release, make therapeutic MRI detectable liposomes a valuable option.
However, despite the excellent results reported so far at preclinical level, the clinical translation of image-guided protocols for stimulated drug release therapies is limited by the difficulty in the approval of the theranostic nanomedicine, which is primarily due to the introduction of the imaging agent.
Gd(III)-based complexes are the almost exclusive class of T1 MRI agents currently used on humans worldwide. However, the last decade has witnessed a growing concern about their clinical use due to two distinct and negative clinical signs associated with the use of such agents: (i) the discovery of the Gd-related pathology Nephrogenic Systemic Fibrosis (NSF) [39], and (ii) the brain accumulation of Gd in specific regions of thalamus (dentate nucleus and globus pallidus) found in patients who were subjected to multiple Gd-based MRI sessions in their life [33]. Though only some of the clinically approved Gd(III) complexes (in particular those with low thermodynamic and kinetic stabilities) were correlated to these negative events, the loading to a nanocarrier invariably alters the biological fate of the Gd(III) complexes, causing a substantial elongation of their permanence in the body and promoting their intracellular uptake. Both these events may promote transmetalation processes that favor the toxic accumulation of gadolinium in the body.
However, it is very important to highlight that gadoteridol, one of the safer clinical agents and the most used T1 agent in MRI-guided drug release preclinical protocols, was only marginally involved in NSF and brain accumulation cases, because of the high thermodynamic and kinetic stabilities displayed by this macrocyclic complex.
Considering that in a stimulated drug release protocol most of the MRI agent is released from the nanocarrier and rapidly excreted without collateral effects, there is a reasonable hope that the near future could witness the entrance in clinical routine of MRI-guided procedures supporting US-induced drug release protocols.
Author Contributions
DP: literature search of the topic of the contribution and preparation of a draft of the article including graphics. DP and ET: organization of the paper structure. ET: revision and finalization of the article. All authors contributed to the article and approved the submitted version.
Funding
The financial supports from Università di Torino/Compagnia San Paolo (project CSTO165439), and Italian Association on Cancer Research (AIRC, project 22041) are gratefully acknowledged.
Conflict of Interest
The authors declare that the research was conducted in the absence of any commercial or financial relationships that could be construed as a potential conflict of interest.
Acknowledgments
The authors want to thank the Consorzio Interuniversitario di Ricerca sulla Chimica dei Metalli nei Sistemi Biologici (CIRCMSB).
References
1. Dagenais GR, Leong DP, Rangarajan S, Lanas F, Lopez-Jaramillo P, Gupta R, et al. Variations in common diseases, hospital admissions, and deaths in middle-aged adults in 21 countries from five continents (PURE): a prospective cohort study. Lancet. (2020) 395:785–94. doi: 10.1016/S0140-6736(19)32007-0
2. Senapati S, Mahanta AK, Kumar S, Maiti P. Controlled drug delivery vehicles for cancer treatment and their performance. Signal Transduct Target Ther. (2018) 3:7. doi: 10.1038/s41392-017-0004-3
3. Alexis F, Pridgen EM, Langer R, Farokhzad OC. Nanoparticle technologies for cancer therapy. Handb Exp Pharmacol. (2010) 197:55–86. doi: 10.1007/978-3-642-00477-3_2
4. Nichols JW, Bae YH. EPR: evidence and fallacy. J Control Release. (2014) 190:451–64. doi: 10.1016/j.jconrel.2014.03.057
5. Sindhwani S, Syed AM, Ngai J, Kingston BR, Maiorino L, Rothschild J, et al. The entry of nanoparticles into solid tumours Nat Mater. (2020) 19:566–75. doi: 10.1038/s41563-019-0566-2
6. Crommelin DJA, van Hoogevest P, Storm G. The role of liposomes in clinical nanomedicine development. What now? Now what? J Control Release. (2020) 318:256–63. doi: 10.1016/j.jconrel.2019.12.023
7. Torchilin VP. Recent advances with liposomes as pharmaceutical carriers. Nat Rev Drug Discov. (2005) 4:145–60. doi: 10.1038/nrd1632
8. Delli Castelli D, Dastrù W, Terreno E, Cittadino E, Mainini F, Torres E, et al. In vivo MRI multicontrast kinetic analysis of the uptake and intracellular trafficking of paramagnetically labeled liposomes. J Control Release. (2010) 144:271–79. doi: 10.1016/j.jconrel.2010.03.005
9. Gianolio E, Arena F, Strijkers GJ, Nicolay K, Högset A, Aime S. Photochemical activation of endosomal escape of MRI-Gd-agents in tumor cells. Magn Reson Med. (2011) 65:212–9. doi: 10.1002/mrm.22586
10. Hijnen N, Langereis S, Grüll H. Magnetic resonance guided high-intensity focused ultrasound for image-guided temperature-induced drug delivery. Adv Drug Deliv Rev. (2014) 72:65–81. doi: 10.1016/j.addr.2014.01.006
11. Yatvin MB, Weinstein JN, Dennis WH, Blumenthal R. Design of liposomes for enhanced local release of drugs by hyperthermia. Science. (1978) 202:1290–3. doi: 10.1126/science.364652
12. Tagami T, Ernsting MJ, Li SD. Efficient tumor regression by a single and low dose treatment with a novel and enhanced formulation of thermosensitive liposomal doxorubicin. J Control Release. (2011) 152:303–9. doi: 10.1016/j.jconrel.2011.02.009
13. Dou YN, Zheng J, Foltz WD, Weersink R, Chaudary N, Jaffray DA, et al. Heat-activated thermosensitive liposomal cisplatin (HTLC) results in effective growth delay of cervical carcinoma in mice. J Control Release. (2014) 178:69–78. doi: 10.1016/j.jconrel.2014.01.009
14. Kneidl B, Peller M, Winter G, Lindner LH, Hossann M. Thermosensitive liposomal drug delivery systems: state of the art review. Int J Nanomedicine. (2014) 9:4387–98. doi: 10.2147/IJN.S49297
15. Wang ZY, Zhang H, Yang Y, Xie XY, Yang YF, Li Z, et al. Preparation, characterization, and efficacy of thermosensitive liposomes containing paclitaxel. Drug Deliv. (2016) 23:1222–31. doi: 10.3109/10717544.2015.1122674
16. Nardecchia S, Sánchez-Moreno P, de Vicente J, Marchal JA, Boulaiz H. Clinical trials of thermosensitive nanomaterials: an overview. Nanomaterials. (2019) 9:191 doi: 10.3390/nano9020191
17. Viglianti BL, Abraham SA, Michelich CR, Yarmolenko PS, MacFall JR, Bally MB, et al. In vivo monitoring of tissue pharmacokinetics of liposome/drug using MRI: illustration of targeted delivery. Magn Reson Med. (2004) 51:1153–62. doi: 10.1002/mrm.20074
18. de Smet M, Langereis S, den Bosch S, van Grüll H. Temperature-sensitive liposomes for doxorubicin delivery under MRI guidance. J Control Release. (2010) 143:120–7. doi: 10.1016/j.jconrel.2009.12.002
19. De Smet M, Heijman E, Langereis S, Hijnen NM, Grüll H. Magnetic resonance imaging of high intensity focused ultrasound mediated drug delivery from temperature-sensitive liposomes: an in vivo proof-of-concept study. J Control Release. (2011) 150:102–10. doi: 10.1016/j.jconrel.2010.10.036
20. Yeo SY, De Smet M, Langereis S, Vander Elst L, Muller RN, Grüll H. Temperature-sensitive paramagnetic liposomes for image-guided drug delivery: Mn2 + versus [Gd(HPDO3A)(H2O)]. Biochim Biophys Acta. (2014) 1838:2807–16. doi: 10.1016/j.bbamem.2014.07.019
21. Hijnen N, Kneepkens E, De Smet M, Langereis S, Heijman E, Grüll H. Thermal combination therapies for local drug delivery by magnetic resonance-guided high-intensity focused ultrasound. Proc Natl Acad Sci USA. (2017) 114:E4802–11. doi: 10.1073/pnas.1700790114
22. Langereis S, Keupp J, Van Velthoven JLJ, De Roos IHC, Burdinski D, Pikkemaat JA, et al. A temperature-sensitive liposomal 1H CEST and19F contrast agent for MR image-guided drug delivery. J Am Chem Soc. (2009) 131:1380–1. doi: 10.1021/ja8087532
23. De Senneville BD, Quesson B, Moonen CTW. Magnetic resonance temperature imaging. Int J Hyperth. (2005) 21:515–31. doi: 10.1080/02656730500133785
24. Liu J, Xu F, Huang J, Xu J, Liu Y, Yao Y, et al. Low-intensity focused ultrasound (LIFU)-activated nanodroplets as a theranostic agent for noninvasive cancer molecular imaging and drug delivery. Biomater Sci. (2018) 6:2838–49. doi: 10.1039/C8BM00726H
25. Zhao XZ, Zhang W, Cao Y, Huang SS, Li YZ, Guo D, et al. A cleverly designed novel lipid nanosystem: targeted retention, controlled visual drug release, and cascade amplification therapy for mammary carcinoma in vitro. Int J Nanomedicine. (2020) 15:3953–64. doi: 10.2147/IJN.S244743
26. Giustetto P, Castelli DD, Boffa C, Rizzitelli S, Durando D, Cutrin JC, et al. Release of a paramagnetic magnetic resonance imaging agent from liposomes triggered by low intensity non-focused ultrasound. J Med Imaging Heal Informatics. (2013) 3:356–66. doi: 10.1166/jmihi.2013.1183
27. van Ballegooie C, Man A, Win M, Yapp DT. Spatially specific liposomal cancer therapy triggered by clinical external sources of energy. Pharmaceutics. (2019) 11:125. doi: 10.3390/pharmaceutics11030125
28. Lin HY, Thomas JL. PEG–lipids and oligo(ethylene glycol) surfactants enhance the ultrasonic permeabilizability of liposomes. Langmuir. (2003) 19:1098–105. doi: 10.1021/la026604t
29. Evjen TJ, Nilssen EA, Barnert S, Schubert R, Brandl M, Fossheim SL. Ultrasound-mediated destabilization and drug release from liposomes comprising dioleoylphosphatidylethanolamine. Eur J Pharm Sci. (2011) 42:380–6. doi: 10.1016/j.ejps.2011.01.002
30. Kang M, Huang G, Leal C. Role of lipid polymorphism in acoustically sensitive liposomes. Soft Matter. (2014) 10:8846–54. doi: 10.1039/C4SM01431F
31. Schroeder A, Avnir Y, Weisman S, Najajreh Y, Gabizon A, Talmon Y, et al. Controlling liposomal drug release with low frequency ultrasound: mechanism and feasibility. Langmuir. (2007) 23:4019–25. doi: 10.1021/la0631668
32. Schroeder A, Honen R, Turjeman K, Gabizon A, Kost J, Barenholz Y. Ultrasound triggered release of cisplatin from liposomes in murine tumors. J Control Release. (2009) 137:63–8. doi: 10.1016/j.jconrel.2009.03.007
33. Rizzitelli S, Giustetto P, Boffa C, Delli Castelli D, Cutrin JC, Aime S, et al. In vivo MRI visualization of release from liposomes triggered by local application of pulsed low-intensity non-focused ultrasound. Nanomedicine. (2014) 10:901–4. doi: 10.1016/j.nano.2014.03.012
34. Lentacker I, De Cock I, Deckers R, De Smedt SC, Moonen CTW. Understanding ultrasound induced sonoporation: definitions and underlying mechanisms. Adv Drug Deliv Rev. (2014) 72:49–64. doi: 10.1016/j.addr.2013.11.008
35. Rizzitelli S, Giustetto P, Faletto D, Delli Castelli D, Aime S, Terreno E. The release of Doxorubicin from liposomes monitored by MRI and triggered by a combination of US stimuli led to a complete tumor regression in a breast cancer mouse model. J Control Release. (2016) 230:57–63. doi: 10.1016/j.jconrel.2016.03.040
36. Gasselhuber A, Dreher MR, Rattay F, Wood BJ, Haemmerich D. Comparison of Conventional chemotherapy, stealth liposomes and temperature-sensitive liposomes in a mathematical model. PLoS ONE. (2012) 7:e47453. doi: 10.1371/journal.pone.0047453
37. Ishihara Y, Calderon A, Watanabe H, Okamoto K, Suzuki Y, Kuroda K, et al. A precise and fast temperature mapping using water proton chemical shift. Magn Reson Med. (1995) 34:814–23. doi: 10.1002/mrm.1910340606
38. Thomsen HS, Morcos SK, Almén T, Bellin MF, Bertolotto M, Bongartz G, et al. Nephrogenic systemic fibrosis and gadolinium-based contrast media: updated ESUR contrast medium safety committee guidelines. Eur Radiol. (2013) 23:307–18. doi: 10.1007/s00330-012-2597-9
Keywords: MRI, ultrasound, liposomes, drug release, nanomedicine
Citation: Patrucco D and Terreno E (2020) MR-Guided Drug Release From Liposomes Triggered by Thermal and Mechanical Ultrasound-Induced Effects. Front. Phys. 8:325. doi: 10.3389/fphy.2020.00325
Received: 25 April 2020; Accepted: 14 July 2020;
Published: 20 August 2020.
Edited by:
Allegra Conti, University of Rome Tor Vergata, ItalyReviewed by:
Maya Thanou, King's College London, United KingdomAndrea Cafarelli, Institute of BioRobotics, Italy
Copyright © 2020 Patrucco and Terreno. This is an open-access article distributed under the terms of the Creative Commons Attribution License (CC BY). The use, distribution or reproduction in other forums is permitted, provided the original author(s) and the copyright owner(s) are credited and that the original publication in this journal is cited, in accordance with accepted academic practice. No use, distribution or reproduction is permitted which does not comply with these terms.
*Correspondence: Enzo Terreno, ZW56by50ZXJyZW5vQHVuaXRvLml0