Immunological Changes During Space Travel: A Ground-Based Evaluation of the Impact of Neutron Dose Rate on Plasma Cytokine Levels in Human Whole Blood Cultures
- 1Radiation Biophysics Division, iThemba LABS (Laboratory for Accelerator Based Sciences), Nuclear Medicine Department, National Research Foundation, Cape Town, South Africa
- 2Radiobiology Unit, Institute for Environment, Health and Safety, Belgian Nuclear Research Center, SCK CEN (Studiecentrum voor Kernenergie Centre d'Étude de l'énergie Nucléaire), Mol, Belgium
- 3BioSkin Lab, Department of Medical Biosciences, Faculty of Natural Sciences, University of the Western Cape, Cape Town, South Africa
- 4Department of Medical Biosciences, Faculty of Natural Sciences, University of the Western Cape, Cape Town, South Africa
A better understanding of the combined impact of different space stressors on human health is urgently warranted, considering the upcoming long-duration missions beyond lower Earth orbit. Therefore, a growing number of particle accelerator facilities implement ground-based set-ups to study the effect of simulated space radiation with simulated psychological or physical stressors. The immune system is highly sensitive to these types of stressors and limited information is currently available on the impact of the complex space radiation environment on the astronauts' immune function. This pilot study presents a first step in the implementation of a ground-based set-up with neutron irradiation, which is considered to be an important secondary component in space radiation fields. The effect of dose rate on immune alterations was studied using the in vitro cytokine release assay. Whole blood samples (n = 8) were exposed to 0.125 or 1 Gy of neutron irradiation (fluence-weighted average energy: 29.8 MeV) at a lower dose rate (LDR) of 0.015 Gy/min and a higher dose rate (HDR) of 0.400 Gy/min. Immediately post-irradiation, blood samples were stimulated with lipopolysaccharide (LPS), heat-killed Listeria monocytogenes (HKLM) or lectin pokeweed mitogen (PWM), and incubated for 24 h. Cell-mediated immunity was examined by analysing interleukin-2 (IL-2), interferon-gamma (IFN-γ), tumour necrosis factor-alpha (TNF-α), and interleukin-10 (IL-10) plasma levels. Stimulants significantly increased all cytokine levels except IL-2, where only PWM induced a significant increase. In general, no statistically significant changes were observed in IL-2, IFN-γ, and TNF-α concentrations at different neutron doses and dose rates when compared to their stimulated, sham-irradiated controls. After PWM-stimulation, IL-10 levels were significantly increased at 0.125 Gy HDR and 1 Gy LDR. In a pooled analysis, the HDR significantly increased IL-2 titres (under PWM-stimulation) and IFN-γ titres (with all stimulants), but significantly decreased TNF-α secretion in unstimulated cultures. Due to the limited sample number, no strong conclusions could be made in this pilot study on the effect of neutron radiation as a single stressor on cytokine secretion in response to different stimuli. However, some interesting trends and dose rate effects were observed, which pave the way for future investigations on the synergistic effects of multiple space stressors on immune cell function.
Introduction
Space travel comprises a unique and complex stress model composed of both physical (cosmic radiation and microgravity) and psychological stress factors, known to induce a large variety of systemic physiological effects that are proven to be detrimental to astronaut's health [1–3]. Of the observed outcomes, a dysfunctional immune system remains a major concern for future manned exploration beyond lower Earth orbit (LEO) or to Mars [4]. Recent studies confirm that immunological changes are an in-flight phenomenon, observed throughout short-duration Space Shuttle missions or a long-duration stay on the International Space Station (ISS) [5–8]. During interplanetary missions, crewmembers will spend an unprecedented amount of time in space, where the synergy of the elevated radiation exposure coupled with persisting immune alterations could potentially increase the cancer incidence, jeopardising astronaut's health and mission success [7, 9–12]. For this reason, the potential impact of space radiation on the dysregulation of normal immune function will be the focus of this pilot study [13, 14].
The innate and adaptive immunity form the pillars of the human immune system and work on both the humoral and cell-mediated level. Humoral immunity has been less extensively studied in astronauts leading to rather inconsistent results [15, 16], while the impairment of cell-mediated immunity has been confirmed during and after spaceflights [4]. NASA's analysis of cell-mediated immunity in the Twin Study, revealed that 50 of the 62 studied cytokines were differentially abundant pre-, in-, and post-flight, between the spaceflight and ground-based subject [8]. Cytokines are produced by virtually all innate and adaptive immune cells, but especially by T-helper (Th) lymphocytes. The measurement of plasma cytokine concentrations in the mitogen-stimulated blood of astronauts has been used to monitor immune function and specific alterations in lymphocyte subpopulations [17]. A cells inability to produce specific cytokines is indicative of a potentially significant immune alteration that could reduce immune defences during spaceflight [18]. Important for effective cell-mediated immunity are the pro-inflammatory interleukin-2 (IL-2), interferon gamma (IFN-γ), and tumour necrosis factor alpha (TNF-α) cytokines, and the anti-inflammatory cytokine interleukin-10 (IL-10). However, these cytokines are not easily classed since they could be capable of inducing both pro-and-anti-inflammatory effects [19].
Mechanisms that produce immune system dysregulation during spaceflight have not been clearly identified, mainly due to the high costs of spaceflight experiments, the scarcity of missions and experimental variations between flights [20]. Terrestrial spaceflight analogues are a more accessible alternative to study spaceflight-related health effects, their causative factors and to test potential countermeasures [21]. For example, several ground-based studies could clearly demonstrate the dramatic impact of microgravity on the immune system [21–28]. However, during space travel, radiation and psychological stress factors (e.g., isolation, sleep deprivation, and heavy workload) may have a significant synergistic or antagonistic effect on human immunity [29, 30]. Here, terrestrial analogues offer not only financial and repeatability advantages but also the opportunity to determine the relative contribution of the individual space stressors to the observed health effect by simulating them both individually and in combination.
Space radiation has been recognized as a major health risk for astronauts as current estimates suggest a round-trip to Mars would result in >0.6 Sv or 60% of an astronaut's career dose [31, 32]. However, uncertainties on the existing biological data limit the risk assessments for manned, deep space mission [33]. To improve the current radiation risk estimation models, a growing number of particle accelerator facilities configure ground-based analogues for biology experiments [34, 35]. The iThemba Laboratory for Accelerator Based Sciences (LABS) is such a particle accelerator facility in South Africa, with a rather unique, well-characterized quasi-monoenergetic neutron beam line, covering an energy range from 30 up to 200 MeV for metrology purposes, with great potential for space radiobiology studies [36]. The space radiation environment is a complex mixture of particles of galactic (galactic cosmic rays or GCR) and solar (solar particle events or SPEs) origin, as well as their secondaries (such as neutrons), that are produced by interactions with the spacecraft materials and astronaut's bodies. Secondary neutrons may cover a complete energy range from thermal neutrons (0.5–1 MeV) up to several GeV and are considered to be highly carcinogenic and far more effective to induce biological damage than low linear energy transfer (LET) radiation [37–40]. Unique patterns of DNA damage, gene expression, repair proteins mobilization, cytokine activation, and cellular microenvironment remodelling are observed following exposure to high-LET radiation.
Particle accelerators are the only facilities where the health risks of high energy neutrons (>20 MeV) can be studied [41]. Therefore, limited information is currently available on the impact of higher energy neutron irradiation on human health, which has been identified as a particular concern for civil aviation [42–44]. During long-term manned missions, both the magnitude and duration of space radiation exposure will increase, subjecting the flight crew to chronic, low doses and low dose rates of secondary neutrons, for which there is limited biological data pertaining to the immune impact. Recently, there has been a growing interest to study the interaction between high-LET radiation and human immunity, to explore the combined use of particle therapy and immunotherapy in cancer treatments [45, 46]. However, the exposure conditions in space and particle therapy are quite different. During particle therapy, an acute, high and localized radiation dose will be delivered to the tumour. In contrast, the space radiation environment is characterised by a chronic, low dose and dose rate of high-LET particles that impact the astronauts whole body and could adversely affect their immune system [10].
New insights and radiobiology data on high-LET radiation effects at low dose rates will improve current risk projections for space exploration and hopefully aid in the development and evaluation of possible countermeasures [47]. With this goal, the “Optimization and validation of a unique ground-based in vitro model to study space health effects” (INVEST) project was launched, which aims to implement a ground-based in vitro model to study space health effects at iThemba LABS, with a specific emphasis on neutrons at low doses and low dose rates. The focus of this first pilot study was to optimize the in vitro cytokine-release assay in collaboration with the project partner SCK CEN, to investigate the impact of low dose rate neutrons on cell-mediated immunity. For this pilot experiment, the clinical fast neutron therapy beam line was used to irradiate whole blood samples of healthy adult volunteers, at a lower dose rate (LDR) or a higher (or normal clinical) dose rate (HDR).
Recall antigens and mitogens were used in this study to assess the impact of neutron irradiation on the cytokine production capacity of activated lymphocytes. A previous study observed no increase in any adaptive immunity cytokines in astronauts 6 months aboard the ISS, suggesting that the astronauts were simply free from infectious diseases while in flight [48]. A potential alternative explanation for this observation is the diminished T lymphocyte function and reduced activation in astronauts due to the spaceflight, which will hinder their cytokine response [49]. Here, the lymphocytes were intentionally stimulated ex vivo to mimic a challenged immune scenario, to monitor the disruptive effect of neutron dose and dose rate on the cytokine release profile. This method has been extensively used to study immune system dysregulation in several ex vivo studies related to short and long duration spaceflights and in terrestrial analogues [13, 29, 50, 51].
Materials and Methods
Sample Collection
Ethical approval was obtained from the South African Human Sciences Research Council (protocol number REC 3/23/10/19) in accordance with the 1975 Declaration of Helsinki, as revised in 2000. Participants were recruited via an institution-wide email addressed to iThemba LABS employees, in Cape Town, South Africa. Written, informed consent was obtained from non-smoking, non-medicating, male volunteers with no diagnosed history of chronic illness (n = 8; age range: 22–63 years). Due to the known variability in cytokine response between individual subjects and the small sample size of this pilot study, the study was restricted to male adult volunteers to limit the potential influence of gender on the circulating cytokines levels in baseline samples [52]. Peripheral blood samples were collected into heparin-treated vacutainers (Becton Dickinson Company, New Jersey, USA) and stored at room temperature before irradiation.
In vitro Irradiation
Whole blood samples were retained at room temperature for ~24 h before being exposed to a clinical fast neutron beam generated by bombarding 66 MeV protons on a Beryllium target (p + 9Be → n + 9B-1.85 MeV, plus several breakup reactions) [53] in sterile 2.0 mL cryogenic vials (NEST Biotechnology Co., Ltd., Wuxi, China). A hydrogenous filter reduced the contribution of thermal and epithermal neutrons. This results in a neutron spectrum with a fluence-weighted average energy of ~29.8 MeV for the 290 mm × 290 mm field that was used for the irradiations [53]. The source-to-phantom surface distance was 1,500 mm and irradiations were carried out at a gantry angle of 270°, resulting in a horizontal beam directed on the water tank containing the blood samples at 37°C. Two radiation doses of 0.125 or 1 Gy were administered at two different dose rates: a lower dose rate (LDR) of 0.015 Gy/min and a higher dose rate (HDR) of 0.400 Gy/min. Sham-irradiated samples were retained in the control room, receiving only ambient radiation. The output factor (1.097 Gy/Monitor Unit) was measured with an Exradin T2 thimble ionization chamber placed at the same position as the blood samples (at a depth of 52 mm in the water tank). The wall of this chamber is made from A-150 tissue-equivalent (TE) plastic and the 0.53 cm3 active chamber volume was filled with a propane-based TE gas. Calibrations were performed according to the neutron dosimetry protocol of the ICRU Report 45 [54].
Whole Blood Immune Cell Stimulation
The in vitro cytokine release assay is a modified version of the methods described by Feuerecker et al. [51] and Van Walleghem et al. [22]. Post-exposure, irradiated whole blood was distributed equally amongst culture tubes and diluted 1 to 1 in 750 μl Roswell Park Memorial Institute (RPMI) medium (Gibco, Massachusetts, USA), supplemented with 10% foetal calf serum (Lonza, Basel, Switzerland), 1% penicillin and streptomycin (Lonza), with-or-without an immune cell stimulant, in sterile 2.0 ml cryovials. Pokeweed mitogen (PWM) (Sigma-Aldrich, Missouri, USA), heat-killed Listeria monocytogenes (HKLM) (InvivoGen, Toulouse, France), or lipopolysaccharide (LPS) (Sigma-Aldrich) were used as immune stimulants at final concentrations of 0.5, 5, and 5 μg/ml, respectively, as previously optimized [21]. Unstimulated samples were included to provide a baseline control condition for every stimulated sample and to confirm that the stimulation achieved the desired effect. Post-irradiation and stimulation (or sham-irradiation and no stimulation, in the case of controls), whole blood cultures were maintained at 37°C for 24 h and the plasma was separated by 1,500 g centrifugation for 15 min before the supernatant was transferred to cryovials and stored at −80°C. The complete experiment is outlined in Figure 1.
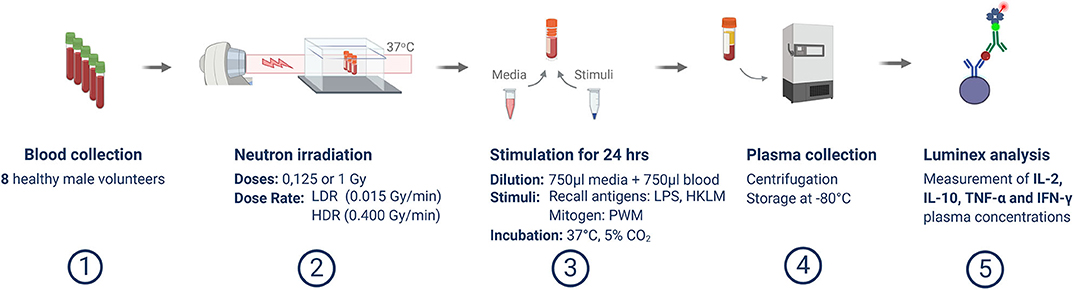
Figure 1. Overview of experimental methodology. This schematic template illustrates the study process from blood sample collection, irradiation and stimulation, up to Luminex analysis.
Luminex Measurement of Cytokine Concentrations
For this pilot study, four key cell-mediated immunity cytokines, IFN-γ, IL-10, IL-2, and TNF-α were quantified down to their respective assay limits of 0.4, 1.6, 1.8, and 1.2 pg/ml. The R&D systems (Minnesota, USA) Luminex assay was conducted exactly to the manufacturer's instructions as described elsewhere [29] with two exceptions: only four cytokines were analysed here and the Luminex assays were performed on a MAGPIX® system (Luminex Corp., Texas, USA). A further 1 to 1 dilution of the plasma in assay diluent was performed to render analyte concentrations within the assay's linear range.
Data Analysis
Statistics
Statistical analysis was conducted using IBM SPSS Statistics 19 (New York, USA) while figures were plotted using GraphPad Prism version 8.0 (California, USA). Cytokine data was presented as grouped subject data with means and standard error of the mean (SEM). In cases where the cytokine concentration could not be confidently extrapolated from the standard curve, the MAGPIX® system reported an “out of range” value. In these instances, the assay's minimum detection limit value was substituted for the “out of range” samples to enable statistical analysis. To ensure normal distribution, data was log10-transformed and verified by a Shapiro Wilk test (data not shown). A repeated measures one-way ANOVA was used to identify significant relationships between the unstimulated and stimulated, irradiated and sham-irradiated samples as well as the relationships between HDR and LDR irradiated samples. An overall dose effect was determined using repeated measures one-way ANOVA analysis on the pooled data of all irradiated samples (0, 0.125, or 1 Gy total dose) for each cytokine-stimulant pair. This elucidates the effect of a specific dose on cytokine secretion compared to the sham-irradiated control, disregarding the dose rate. Similarly, the dose rate effect was determined using the same analysis on the pooled dose rate results within each cytokine-stimulant pair, regardless of the administered radiation dose. Relationships were deemed significant when P < 0.05.
Effect Size Calculations
Due to the small sample size of this study and the resulting low power to test statistical significance, effect size (ES) values were calculated to determine the size by which the experimental group varies from the control groups, expressed as the number of standard deviations. A negative ES value specifies that the experimental group mean is “x” amount of standard deviations below the control mean, while a positive value indicates the contrary. The ES assists in describing trends and relationships between groups and is defined by the formula:
In the present study, the ES was calculated to identify the effect of various neutron doses in reference to the sham-irradiated control. However, in order to determine the effect of neutron dose rate, the lower, 0.015 Gy/min dose rate was compared to the standard higher, 0.400 Gy/min dose rate, so the HDR group is used as the “control” in the formula above.
Results
Validation of the Cytokine Release Assay
Cytokines are soluble molecules which play a key role in innate and adaptive immune responses. To validate the in vitro cytokine release assay used for this pilot study, cytokine titres in the unstimulated (baseline) samples were compared to the stimulated samples. With a few exceptions, only minimal detectable cytokine concentrations were observed at baseline for all donors and the majority of the basal IL-2 and IFN-γ cytokine levels were even below the detection limit for most donors (Figure 2). Based on previous studies, it was expected that the different stimuli would up-regulate plasma cytokine levels in whole blood cultures [55]. This was indeed observed for three of the four measured cytokines (IFN-γ, IL-10, and TNF-α), irrespective of the stimulant used for lymphocyte activation (Figure 2). IL-2 concentrations deviated from this trend. For some of the donors, the measured values were still below the assay detection limit of 1.8 pg/ml after 24 h of stimulation and out of the normal physiological range for healthy adults (9.4–15.9 pg/ml) [56]. Minor, non-significant increases in IL-2 concentration were observed after 24-h stimulation with HKLM or LPS for 50 and 25% of the donors, respectively. PWM-stimulation on the contrary, induced a significant IL-2 increase for all donors compared to the basal levels (P < 0.001; Figure 2C).

Figure 2. Cytokine release assay validation—recall antigen and mitogen stimulation of whole blood samples. The graphs display the increase in log10-transformed, cytokine titres between the unstimulated (Basal) and the recall antigen- or mitogen-stimulated (PWM, HKLM, or LPS) samples. The dots represent the measurements of the individual participants and the horizontal lines denote means. The asterisks indicate various levels of significant difference (***P < 0.001 and *P < 0.05). (A) All three stimuli significantly increased IFN-γ secretion. (B) All three stimuli result in a significant increase of IL-10 secretion compared to the basal level. (C) Only PWM significantly increased IL-2 titres. (D) All three stimuli resulted in a significant increase in TNF-α levels.
Impact of Neutron Dose and Dose Rate on Cytokine Release
In most cases, neutron irradiation had no statistically significant impact on cytokine secretion in stimulated and unstimulated whole blood cultures, as depicted by the comparison of group means in Figure 3. Under PWM-stimulation however, exceptions to the norm were observed when the measured IL-10 concentration in the sham-irradiated cultures was compared to the concentration in either the 1 Gy LDR-treated group, or the 0.125 Gy HDR-treated group (P < 0.05; Figure 3E). The lack of statistical significance under the other stimulant or neutron treatment conditions might be attributable to the low participant numbers, resulting in a lack of statistical power to test the hypothesis. Nonetheless, some interesting but non-significant trends were observed, that allude to response patterns.
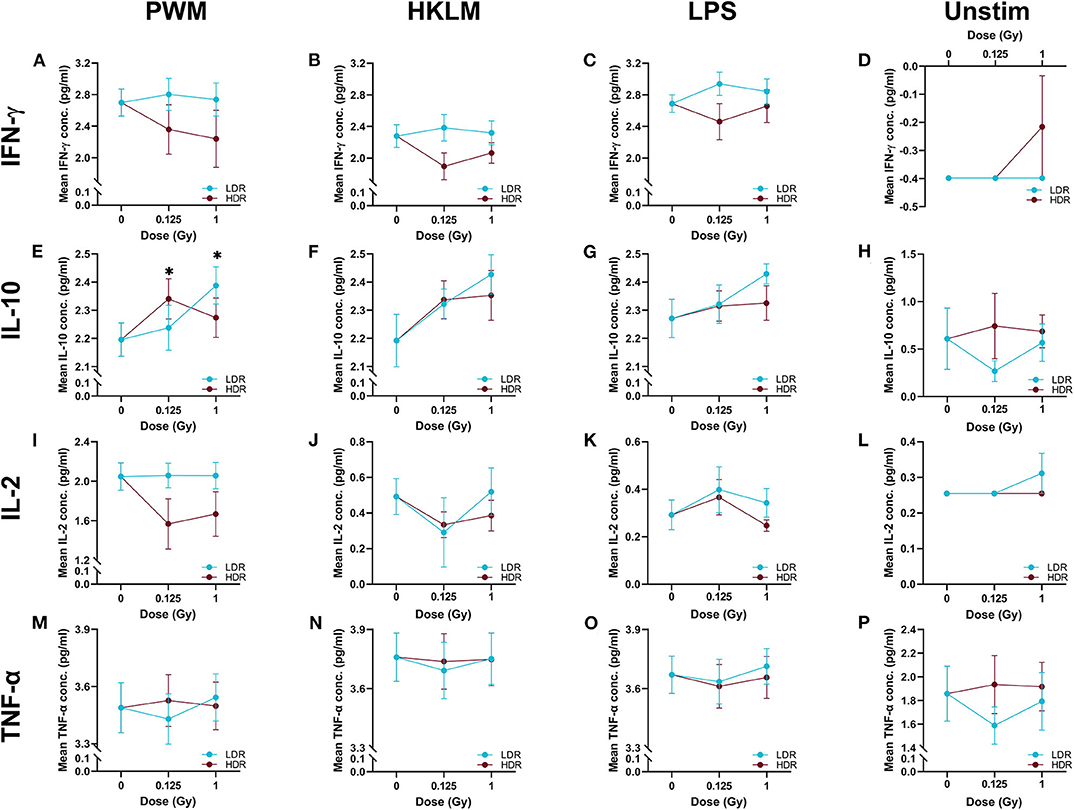
Figure 3. The log10-transformed mean cytokine concentrations. The above figures display the mean IFN-γ, IL-10, IL-2, and TNF-α titres in the stimulated (n = 8) and unstimulated (n = 6), irradiated and sham-irradiated groups, with error bars indicating the SEM. When stimulated with PWM, 0.125 Gy neutrons given at the higher dose rate and 1 Gy given at the lower dose rate, significantly increase IL-10 secretion (*P < 0.05) when compared to the sham-irradiated control (Graph E). Graphs (A) to (D) and (F) to (P) display no significant relationships.
To independently monitor the effect of individual neutron doses and dose rates on cell-mediated immunity parameters, cytokine concentrations from various groups were pooled by disregarding either the dose rate (for dose comparisons) or the dose (for dose rate comparisons), within each stimulant and cytokine group. Table 1 presents the observations from these pooled comparisons. Again, a significant increase was observed in IL-10 secretion in PWM-stimulated samples compared to the stimulated control group concentration, for both the low and higher neutron dose of 0.125 (P < 0.05) and 1 Gy (P < 0.01). Moreover, the pooled comparison between the LDR and HDR highlights significantly suppressed IFN-γ secretion after HDR exposure in all stimulated cultures (P < 0.01) and a significantly suppressed IL-2 secretion in PWM-stimulated cultures (P < 0.05; Table 1). A “native” response to HDR neutron treatment was observed by a significant increase in TNF-α in unstimulated cultures (P < 0.01; Table 1).
Effect Size
Effect size (ES) calculations were an eloquent solution to highlight trends between normally distributed groups, in light of the limited sample size and the large intra-participant cytokine response variation (Table 2). To see the influence of the neutron radiation dose and dose rate on in vitro cytokine secretion, the data analysis strategy detailed in the Effect Size Calculations section, was employed.
Dose Effect Size
“Dose ES” calculations revealed a downregulation of IL-2 secretion compared to the unirradiated samples for most stimuli conditions, except for LPS, after both the 0.125 and 1 Gy dose, delivered at the HDR. This effect was most pronounced for PWM-stimulated cultures and it is advisable to only focus on the PWM results since IL-2 levels in LPS and HKLM stimulated cultures were below the detection limit in most cases (Figure 2). There was a general increase in IL-10 concentration in all irradiated conditions compared to the unirradiated samples, regardless of the stimulant used. However, the LDR minimally suppressed IL-10 secretion in the unchallenged immune system at 0.125 Gy (Figure 3H).
Considering the IFN-γ ES data, the HDR seemed to have a more pronounced impact on the cytokine secretion levels at both 0.125 and 1 Gy, regardless of the stimulant employed (Table 2). The LDR-treated samples typically showed higher, slight increases in IFN-γ plasma levels at 0.125 and 1 Gy. Unfortunately, no ES could be identified for unstimulated (basal) samples since IFN-γ baseline values were below the detectable limit of the assay. Neutron treatment had minimal impact on the TNF-α concentrations except in the unstimulated groups where the 0.125 Gy LDR marginally reduced TNF-α secretion in comparison to the same HDR dose point.
Dose Rate Effect
While Tables 1, 2 mainly focus on the statistical significance of the observed effects and the size of the shifts in cytokine levels between the LDR and HDR, it is advisable to consult (Figure 3) which illustrates the potential decrease or increase of the observed trend. The “Dose rate ES” analysis indicates consistently higher IL-2 and IL-10 levels at the 1 Gy neutron dose in the LDR treatment arm compared to HDR arm for all stimuli. For IL-2, the combination of the LDR-0.125 Gy and PWM-stimulation resulted in higher cytokine levels compared to the HDR-0.125 Gy condition, while the opposite effect on IL-10 levels. The most reliable IL-2 conclusions can be drawn on the PWM stimulation, which suggest a dose rate effect, whereas the effects on IL-10 levels seem to be dose-specific with no conclusive dose rate effect. ES calculations confirmed the pooled statistical analysis of IFN-γ measurements as there was a clear trend in dose rate effect (Table 2), where the HDR exposures gave rise to a decrease in IFN-γ secretion for the three different stimuli at both neutron doses (Figures 3A–C) when compared to the LDR treatment group and the unirradiated control samples. Considering TNF-α dose rate ES data, the LDR seems to reduce cytokine secretion in the unchallenged immune system but the dose rate had marginal impact on the cytokine levels in stimulated cultures.
Discussion
Although it is well-known that dysregulation of the immune function occurs and persists during spaceflight, the exact nature of the immunological changes and their specific cause has not yet been fully elucidated. Therefore, the present study used the in vitro cytokine release assay to monitor alterations in the plasma levels of pro- (IFN-γ, IL-2, and TNF-α) and anti-inflammatory (IL-10) cytokines induced by low (0.125 Gy) or high (1 Gy) neutron doses administered at two different dose rates (0.400 or 0.015 Gy/min). The cytokine response of the immune cells was measured after 24 h of stimulation with mitogens or recall antigens. To the best of our knowledge, this is the first study measuring the effect of neutron irradiation and potential dose rate effects on the cytokine release capacity of immune cells, to address the knowledge gap on chronic exposure to neutrons in light of the anticipated interplanetary travel and exploration of the Martian surface [57–59]. In general, this pilot study did not reveal statistically significant changes in cytokine release after neutron irradiation. However, some first trends after neutron exposure were observed depending on the cytokine and the stimuli used.
During the in vitro cytokine release assay, whole blood samples were supplemented with recall antigens or mitogens (PWM, HKLM, and LPS). The addition of the stimuli resulted in an expected upregulation of plasma cytokine levels (Figure 2) in line with previous observations, confirming the efficacy of the assay [55]. Basal IL-2 and IFN-γ concentrations were below the assay detection limit, indicating that the study population was indeed healthy and there was no sign of infection [56]. In a previous study, IL-2 secretion was only increased for 50% of the subjects after exposure to LPS [22]. We observed an increase for only 25% of the donors after LPS stimulation. Moreover, the only statistically significant increase in IL-2 concentration was observed after PWM stimulation. IL-2 acts by priming CD8+ T cells to eliminate virus-infected cells. The low IL-2 secretion following stimulation with HKLM and LPS are possibly due to these mitogens mimicking a bacterial, rather than a viral challenge.
At the unstimulated baseline, TNF-α concentrations were within the expected normal physiological range with one participant showing a titre roughly 50% higher than the other study participants. Studies conducted to investigate cytokine levels in healthy subjects have established differing “normal” baseline cytokine profiles based on the characteristics of their study populations and the method of cytokine measurement [19, 60]. Based on the great variation in baseline cytokine levels amongst donors and the limited availability of comparative study data, it was decided that none of the donors could be excluded from the statistical analysis in this study based on a literature comparison alone. In addition, a large intra-individual variation was also observed in the exposed samples which is reflected by the relatively broad error bars in Figure 3. While no conclusive results could be obtained in the present study, it might be valuable to investigate cytokines responses at individual level after irradiation exposure in future studies.
Evidence suggests that IL-2 stimulation during the primary immune response is critical for secretion of CD8+ T lymphocytes to combat viral infections [61]. During an infection, a co-ordinated action by pro-inflammatory cytokines must guarantee the clearing of the invading pathogen. Once this is achieved, they are downregulated by anti-inflammatory cytokines such as IL-10, before tissue damage or pathology occurs. A timely and dynamic balance exists between pro- and anti-inflammatory cytokines to ensure an infection is resolved [62]. In this study, only the effects of neutron irradiation on the PWM-induced IL-2 secretion can be taken in consideration, since all the other stimulation conditions produced IL-2 concentrations that were below the assay detection limit (Figure 2). A non-statistically significant trend was observed for the dose rate in the PWM-stimulated group, where the HDR suppressed IL-2 secretion compared to LDR exposure for both 0.125 and 1 Gy (Figure 3I). In a challenged immune system, a potential neutron-induced IL-2 suppression could give rise to reduced primary and secondary antigen-specific CD8+ expansion and an inefficient immune response [63].
IL-10 is described as a pleiotropic cytokine, since it acts in both innate and adaptive immunity as an anti-inflammatory molecule [64] and is involved in the prevention and limitation of immune reactions [65]. A significant increase was observed in IL-10 secretion under PWM-stimulation and neutron treatment at LDR 1 Gy and HDR 0.125 Gy (Figure 3E). IL-10 was the only anti-inflammatory cytokine included in this study and the only cytokine that showed a consistent, but not statistically significant upregulation after neutron irradiation and stimulation (Table 2—left side). These results indicate that neutron irradiation could induce anti-inflammatory activity, independent of the dose rate.
Previous research conducted on rats flown on the US Space Shuttle revealed that the animals had suppressed IFN-γ levels which could be associated with a heightened risk to viral infections [66]. IFN-γ plays an essential role in the innate and adaptive immune response against pathogens and tumour development but unregulated IFN-γ secretion can cause pathological inflammatory conditions [67]. The grouped dose rate analysis highlighted an inverse relationship between IFN-γ levels and dose rate, suggesting that the LDR may facilitate a competent immune response to viral challenge, while the HDR resulted in a non-statistically significant downregulation after stimulation. This trend, which can be observed in Figures 3A–C, and was confirmed by the effect size calculations that showed a suppression of the IFN-γ secretion after HDR irradiation in response to stimulation (Table 2—left side).
TNF-α is an important factor for T lymphocyte signalling and activation to induce an appropriate response from initiation to pathogen elimination and has a well-defined role in the defence against several bacterial pathogens [68, 69]. In this study, TNF-α is the only cytokine for which no real trends could be observed in the stimulated, irradiated whole blood cultures. However, the upregulation of IL-10 secretion might have antagonised the TNF-α levels in the stimulated cultures. An interesting yet not statistically significant response was observed in the unstimulated group, where LDR neutrons downregulated the TNF-α secretion after 0.125 Gy compared to the HDR (Figure 3P). This suggests that, even before mitogen stimulation, a dose rate-dependent response is visible and LDR neutron radiation might induce a suppressed ability to mount an effective immune response.
Significant reductions in mitogen-stimulated production of different cytokines was observed in peripheral blood mononuclear cells isolated from whole blood samples, that were collected during long-duration spaceflight [7]. The inability of the dysregulated immune system to respond and clear infections or pre-cancerous cells is concerning in light of the confined living condition and radiation-rich environment that astronauts will inhabit during interplanetary trips. While most of the observed immune alterations up to now have been linked and attributed to microgravity conditions in ground-based set-up, some components of the immune system are among the most radiosensitive tissues in the body. Unfortunately, results on the effect of cosmic radiation on the immune system remain limited [10, 20]. Most evidence for the effects of space radiation exposures in humans has been derived from epidemiological studies on the atomic-bomb survivors, radiotherapy patients, and occupationally exposed workers. While these studies provide valuable insights, our knowledge on the true risks from low dose rate exposure to high-LET radiation relevant to space is limited and remains one of the main challenges to predict space radiation health risks for exploration astronauts [70]. Currently, NASA's most advanced GCR simulators provides some insight into the effect of GCR on biological systems [70]. A recent study by Moreno-Villanueva and colleagues examined the combined and individual effects of γ-radiation (radiation source undefined), simulated microgravity and physiological stress on isolated, unstimulated, peripheral blood mononuclear cells [29]. They concluded that radiation significantly influenced cytokine secretion but only under simulated microgravity conditions. In addition, pre-exposure to simulated psychological stress mitigated the effects of microgravity. These important findings suggest possible synergistic effects that may offer some level of protective compensation during spaceflight. Since no additional space stressors were used in the current pilot study, this could also be one of the reasons why almost no significant effects on cytokine secretion could be observed with neutron irradiation alone.
When astronauts go beyond LEO, they are exposed to high doses of space radiation, consisting of protons and heavy charged nuclei, as well as secondary neutrons of a wide spectrum of energies produced by interaction of the charge particles with the human body, spacecraft and other material surroundings [71]. Most existing studies on neutron radiation focus on their carcinogenic risks and are primarily based on experiments with exposures to neutron energies below 20 MeV, while simulation and dosimetry studies illustrate that neutron energies in space environment go up to much higher energies [58]. In addition, measurements on the Martian surface show that neutrons will be a significant contributor to measured absorbed dose and it is estimated that they contribute ~15% toward the intravehicular dose to astronauts [57, 58, 72]. Though the immune impact of neutron irradiation is poorly studied, a gene expression study by Broustas et al. illustrated a clear suppression of immune cell function after 1 Gy neutron irradiation and downregulation of genes that were related to the immune system response and B and T cell physiology [73].
This pilot study has several limitations, where the small study population presented one of the main constraints to draw statistically significant conclusions. However, it is a first illustration of the size of variation, which can form the basis for appropriate sample sizes calculation and to test the feasibility of future experiments [74, 75]. The LDR in the current study is still much higher than the expected intravehicular dose rate during cosmic travel, which is estimated be 0.3–0.6 mGy per day or 2.08 × 10−4 to 4.17 × 10−4 mGy/min [58]. The measured dose rate for the Martian neutron spectrum ranges from 8 to 740 MeV was 0.014 ± 0.004 mGy/day [57, 59]. We were unfortunately limited to a dose rate of 0.015 Gy/min due to the detection limits of the electronics of the clinical neutron therapy beam line that was used in this study. However, this 25-time reduction in dose rate compared to the HDR of 0.400 Gy/min, was considered to be sufficient for this proof-of-principle study. Moreover, we were able to demonstrate a neutron dose rate effect on DNA double strand break induction as early as 30 min after irradiation with the same irradiation set-up [50]. It should also be noted that an absorbed neutron dose of 1 Gy as used in this pilot study is not trivial and is possibly much higher than the expected absorbed neutron doses for astronauts on a Mars mission. However, since this was a pilot study, it was decided to include a low (0.125 Gy) and high (1 Gy) neutron dose in order to evaluate the effect of the dose in this first step of the project. Neutron exposures of this magnitude are expected to induce apoptosis in a substantial proportion of the lymphocyte population and the lack of viability or apoptosis data can be seen as a limitation in this study.
Despite the limited number of study participants, the results demonstrate some first trends in certain cytokine secretions after irradiation with fast neutrons, where dose rate only seems to have an impact on the IFN-γ and IL-2 secretion after specific stimulation. However, the majority of these observations were not statistically significant. Another important limitation of this pilot study is the use of whole blood samples and not highly purified subpopulations. Therefore, this method does not provide the ability to identify the specific population of cells responsible for the observed increase or decrease in cytokine secretion. The use of whole blood may also be advantageous because it can display differences in cytokine secretion due to complex cell–cell interactions and the plasma, thereby better reflecting the in vivo environment. Notwithstanding these limitations and with great caution taken to not generalise the effects from a finite sample population, we can conclude that there is future scope for these types of experiments.
An important objective for future experiments is to take the additional layers of spaceflight complexity into consideration by mimicking the synergistic effect of multiple space stressors in a ground-based experiment, as outlined by Moreno-Villanueva et al. [29]. In addition, the future goal should also include in vivo experiments with neutrons and other high-LET radiation qualities relevant to the space radiation environment, with special emphasis for spaceflight-relevant low dose rates. Moreover, future studies should include cell viability and cell cycle assessments in order to take into consideration the starting concentration of cells in each sample, which can have a significant effect on the cytokine measurements. Unfortunately, lower dose rates remain technically challenging to administer to biological samples and beam time limitations at accelerator facilities might hinder the administration of the ultra-low dose rates observed in space. In spite of these limitations, the INVEST collaboration endeavours to further ground-based space research in Africa and to examine the individual and combined effects of spaceflight stressors (radiation, physiological stress, and microgravity) on the human immune system. Given the intended future of human spaceflight and the rapid expansion of the capabilities for human missions to the moon and Mars, there is a pressing need to improve the understanding of the space radiation risk, predict likely clinical outcomes of interplanetary radiation exposure, and develop appropriate and effective mitigation strategies for future missions [70]. The current study on low dose and dose rate neutron irradiation presents a first small step toward the giant leap still needed to achieve this goal.
Data Availability Statement
The raw data supporting the conclusions of this article will be made available by the authors, without undue reservation.
Ethics Statement
The studies involving human participants were reviewed and approved by the South African Human Sciences Research Council (HSRC) Research Ethic Committee in affiliation with the South African National Health Research Ethics Council of the SA National Department of Health (NHREC No. 290808-015) and also has US OHRP Federal-wide Assurance (FWA) accreditation (FWA 00006347, IRB No. 00003962). The patients/participants provided their written informed consent to participate in this study.
Author Contributions
CV, MM, and BB conceptualised and designed the experiments. JN-C, EK, XM, SN, PP, ME, RN, JB, CV, and RF performed the irradiation experiments, dosimetry, and laboratory work. BB and RV provided training to RF in the in vitro cytokine release assay. CV, BB, RV, and RF analysed the data and FR performed the statistical analysis. CV, FR, and RF wrote the paper. CV, MM, and SB were the grant holders of the INVEST project. CV was the principal investigator in South Africa and secured funding for the experiments. All authors contributed to and approved the final version of the article.
Funding
Funding for the collaborative networking between South Africa and Belgium for this study was made available by the joint SA-NRF and Belgian Federal Science Policy Office (BELSPO) funding program under grant reference number BELS180425324336. Research consumables and beam time costs were funded by NRF iThemba LABS institutional grant. EU Horizon 2020 - EURopeAn MEDical application and Radiation prOteCtion Concept: strategic research agenda aNd ROadmap interLinking to heaLth and digitization aspects (EURAMED Rocc-n-roll) research funding, with grant agreement number 899995 of SB.
Conflict of Interest
The authors declare that the research was conducted in the absence of any commercial or financial relationships that could be construed as a potential conflict of interest.
Acknowledgments
The authors would like to thank the study participants for their gracious blood donations, as well as Sister Yvette Macdonald, for blood sample collection. We must thank the National Research Foundation of South Africa for funding this collaboration and the Physics Advisory Committee of iThemba LABS for beam time approval.
References
2. Taylor GR, Dardano JR. Human cellular immune responsiveness following space flight. Aviat Space Environ Med. (1983) 54:S55–9.
4. Frippiat JP, Crucian BE, de Quervain DJ, Grimm D, Montano N, Praun S, et al. Towards human exploration of space: the THESEUS review series on immunology research priorities. NPJ Microgr. (2016) 2:16040. doi: 10.1038/npjmgrav.2016.40
5. Nagasawa H, Huo L, Little JB. Increased bystander mutagenic effect in DNA double-strand break repair-deficient mammalian cells. Int J Radiat Biol. (2003) 79:35–41. doi: 10.1080/713864984
6. Taylor GR, Neale LS, Dardano JR. Immunological analyses of US space shuttle crewmembers. Aviat Space Environ Med. (1986) 57:213–7.
7. Crucian B, Stowe RP, Mehta S, Quiriarte H, Pierson D, Sams C. Alterations in adaptive immunity persist during long-duration spaceflight. NPJ Microgr. (2015) 1:15013. doi: 10.1038/npjmgrav.2015.13
8. Garrett-Bakelman FE, Darshi M, Green SJ, Gur RC, Lin L, Macias BR, et al. The NASA twins study: a multidimensional analysis of a year-long human spaceflight. Science. (2019) 364:eaau8650. doi: 10.1126/science.aau8650
9. Crucian BE, Choukèr A, Simpson RJ, Mehta S, Marshall G, Smith SM, et al. Immune system dysregulation during spaceflight: potential countermeasures for deep space exploration missions. Front Immunol. (2018) 9:1437. doi: 10.3389/fimmu.2018.01437
10. Fernandez-Gonzalo R, Baatout S, Moreels M. Impact of particle irradiation on the immune system: from the clinic to mars. Front Immunol. (2017) 8:177. doi: 10.3389/fimmu.2017.00177
11. Fernandes JV, Cobucci RN, Jatoba CA, Fernandes TA, de Azevedo JW, de Araujo JM. The role of the mediators of inflammation in cancer development. Pathol Oncol Res. (2015) 21:527–34. doi: 10.1007/s12253-015-9913-z
12. Grivennikov SI, Greten FR, Karin M. Immunity, inflammation, and cancer. Cell. (2010) 140:883–99. doi: 10.1016/j.cell.2010.01.025
13. Gueguinou N, Huin-Schohn C, Bascove M, Bueb JL, Tschirhart E, Legrand-Frossi C, et al. Could spaceflight-associated immune system weakening preclude the expansion of human presence beyond earth's orbit? J Leukoc Biol. (2009) 86:1027–38. doi: 10.1189/jlb.0309167
14. Akiyama T, Horie K, Hinoi E, Hiraiwa M, Kato A, Maekawa Y, et al. How does spaceflight affect the acquired immune system? NPJ Microgr. (2020) 6:14. doi: 10.1038/s41526-020-0104-1
15. Rykova MP, Antropova EN, Larina IM, Morukov BV. Humoral and cellular immunity in cosmonauts after the ISS missions. Acta Astronaut. (2008) 63:697–705. doi: 10.1016/j.actaastro.2008.03.016
16. Konstantinova IV, Rykova MP, Lesnyak AT, Antropova EA. Immune changes during long-duration missions. J Leukoc Biol. (1993) 54:189–201. doi: 10.1002/jlb.54.3.189
17. Crucian BE, Cubbage ML, Sams CF. Altered cytokine production by specific human peripheral blood cell subsets immediately following space flight. J Interferon Cytokine Res. (2000) 20:547–56. doi: 10.1089/10799900050044741
18. Sonnenfeld G. Effect of space flight on cytokine production. Acta Astronaut. (1994) 33:143–7. doi: 10.1016/0094-5765(94)90119-8
19. Monastero RN, Pentyala S. Cytokines as biomarkers and their respective clinical cutoff levels. Int J Inflam. (2017) 2017:4309485. doi: 10.1155/2017/4309485
20. Moreels M, de Saint-Georges L, Vanhavere F, Baatout S. Stress and radiation responsiveness. In: Choukèr A, editor. Stress Challenges and Immunity in Space: From Mechanisms to Monitoring and Preventive Strategies. Cham: Springer Nature Switzerland AG (2011). p. 239. doi: 10.1007/978-3-642-22272-6_17
21. Crucian B, Simpson RJ, Mehta S, Stowe R, Chouker A, Hwang S-A, et al. Terrestrial stress analogs for spaceflight associated immune system dysregulation. Brain Behav Immun. (2014) 39:23–32. doi: 10.1016/j.bbi.2014.01.011
22. Van Walleghem M, Tabury K, Fernandez-Gonzalo R, Janssen A, Buchheim J-I, Choukèr A, et al. Gravity-related immunological changes in human whole blood cultured under simulated microgravity using an in vitro cytokine release assay. J Interferon Cytokine Res. (2017) 37:531–40. doi: 10.1089/jir.2017.0065
23. Morey-Holton E, Globus R, Kaplansky A, Durnova G. The hindlimb unloading rat model: literature overview, technique update and comparison with space flight data. Adv Space Biol Med. (2005) 10:7–40. doi: 10.1016/S1569-2574(05)10002-1
24. Hoff P, Belavý DL, Huscher D, Lang A, Hahne M, Kuhlmey A-K, et al. Effects of 60-day bed rest with and without exercise on cellular and humoral immunological parameters. Cell Mol Immunol. (2015) 12:483–92. doi: 10.1038/cmi.2014.106
25. Shearer WT, Ochs HD, Lee B-N, Cohen EN, Reuben JM, Cheng I, et al. Immune responses in adult female volunteers during the bed-rest model of spaceflight: antibodies and cytokines. J Allergy Clin Immunol. (2009) 123:900–5. doi: 10.1016/j.jaci.2008.12.016
26. Sonnenfeld G. The immune system in space and microgravity. Med Sci Sports Exerc. (2002) 34:2021–7. doi: 10.1097/00005768-200212000-00024
27. Sonnenfeld G, Butel JS, Shearer WT. Effects of the space flight environment on the immune system. Rev Environ Health. (2003) 18:1–17. doi: 10.1515/REVEH.2003.18.1.1
28. Cogoli A, Bechler B, Cogoli-Greuter M, Criswell SB, Joller H, Joller P, et al. Mitogenic signal transduction in tlymphocytes in microgravity. J Leukoc Biol. (1993) 53:569–75. doi: 10.1002/jlb.53.5.569
29. Moreno-Villanueva M, Feiveson AH, Krieger S, Kay Brinda A, von Scheven G, Bürkle A, et al. Synergistic effects of weightlessness, isoproterenol, and radiation on DNA damage response and cytokine production in immune cells. Int J Mol Sci. (2018) 19:3689. doi: 10.3390/ijms19113689
30. Yatagai F, Honma M, Dohmae N, Ishioka N. Biological effects of space environmental factors: a possible interaction between space radiation and microgravity. Life Sci Space Res. (2019) 20:113–23. doi: 10.1016/j.lssr.2018.10.004
31. Zeitlin C, Hassler D, Cucinotta F, Ehresmann B, Wimmer-Schweingruber R, Brinza D, et al. Measurements of energetic particle radiation in transit to Mars on the Mars science laboratory. Science. (2013) 340:1080–4. doi: 10.1126/science.1235989
32. Cucinotta FA, Chappell LJ. Updates to astronaut radiation limits: radiation risks for never-smokers. Rad Res. (2011) 176:102–14. doi: 10.1667/RR2540.1
33. Chancellor JC, Scott GB, Sutton JP. Space radiation: the number one risk to astronaut health beyond low earth orbit. Life. (2014) 4:491–510. doi: 10.3390/life4030491
34. Walsh L, Schneider U, Fogtman A, Kausch C, McKenna-Lawlor S, Narici L, et al. Research plans in Europe for radiation health hazard assessment in exploratory space missions. Life Sci Space Res. (2019) 21:73–82. doi: 10.1016/j.lssr.2019.04.002
35. Durante M, Golubev A, Park W-Y, Trautmann C. Applied nuclear physics at the new high-energy particle accelerator facilities. Phys Rep. (2019) 800:1–37. doi: 10.1016/j.physrep.2019.01.004
36. Mosconi M, Musonza E, Buffler A, Nolte R, Röttger S, Smit F. Characterisation of the high-energy neutron beam at iThemba LABS. Radiat Measure. (2010) 45:1342–5. doi: 10.1016/j.radmeas.2010.06.044
37. Broerse J. Biological effects of neutrons. In: Thomas RH, Perez-Mendez V, editors. Advances in Radiation Protection and Dosimetry in Medicine. Boston, MA: Springer Science & Business Media (1980). p. 415–29.
38. Goodhead DT. Neutrons are forever! historical perspectives. Int J Radiat Biol. (2019) 95:957–84. doi: 10.1080/09553002.2019.1569782
40. Armstrong T, Colborn B. Predictions of secondary neutrons and their importance to radiation effects inside the International Space Station. Radiat Measure. (2001) 33:229–34. doi: 10.1016/S1350-4487(00)00152-9
42. Heilbronn LH, Borak TB, Townsend LW, Tsai P-E, Burnham CA, McBeth RA. Neutron yields and effective doses produced by Galactic Cosmic Ray interactions in shielded environments in space. Life Sci Space Res. (2015) 7:90–9. doi: 10.1016/j.lssr.2015.10.005
43. Goldhagen P, Clem J, Wilson J. The energy spectrum of cosmic-ray induced neutrons measured on an airplane over a wide range of altitude and latitude. Radiat Protect Dosim. (2004) 110:387–92. doi: 10.1093/rpd/nch216
44. O'sullivan D. Exposure to galactic cosmic radiation and solar energetic particles. Radiat Protect Dosim. (2007). 125:407–11. doi: 10.1093/rpd/ncm317
45. Helm A, Ebner DK, Tinganelli W, Simoniello P, Bisio A, Marchesano V, et al. Combining heavy-ion therapy with immunotherapy: an update on recent developments. Int J Part Ther. (2018) 5:84–93. doi: 10.14338/IJPT-18-00024.1
46. Ebner DK, Tinganelli W, Helm A, Bisio A, Yamada S, Kamada T, et al. The immunoregulatory potential of particle radiation in cancer therapy. Front Immunol. (2017) 8:99. doi: 10.3389/fimmu.2017.00099
47. Takahashi A, Ikeda H, Yoshida Y. Role of high-linear energy transfer radiobiology in space radiation exposure risks. Int J Part Ther. (2018) 5:151–9. doi: 10.14338/IJPT-18-00013.1
48. Crucian BE, Zwart SR, Mehta S, Uchakin P, Quiriarte HD, Pierson D, et al. Plasma cytokine concentrations indicate that in vivo hormonal regulation of immunity is altered during long-duration spaceflight. J Interferon Cytokine Res. (2014) 34:778–86. doi: 10.1089/jir.2013.0129
49. Crucian BE, Stowe RP, Pierson DL, Sams CF. Immune system dysregulation following short-vs long-duration spaceflight. Aviat Space Environ Med. (2008) 79:835–43. doi: 10.3357/ASEM.2276.2008
50. Nair S, Engelbrecht M, Miles X, Ndimba R, Fisher R, du Plessis P, et al. The impact of dose rate on DNA double-strand break formation and repair in human lymphocytes exposed to fast neutron irradiation. Int J Mol Sci. (2019) 20:5350. doi: 10.3390/ijms20215350
51. Feuerecker M, Mayer W, Kaufmann I, Gruber M, Muckenthaler F, Yi B, et al. A corticoid-sensitive cytokine release assay for monitoring stress-mediated immune modulation. Clini Exp Immunol. (2013) 172:290–9. doi: 10.1111/cei.12049
52. Koelman L, Pivovarova-Ramich O, Pfeiffer AF, Grune T, Aleksandrova K. Cytokines for evaluation of chronic inflammatory status in ageing research: reliability and phenotypic characterisation. Immun Ageing. (2019) 16:11. doi: 10.1186/s12979-019-0151-1
53. Jones D, Symons J, Fulcher T, Brooks F, Nchodu M, Allie M, et al. Neutron fluence and kerma spectra of ap (66)/Be (40) clinical source. Med Phys. (1992) 19:1285–91. doi: 10.1118/1.596922
54. Mijnheer BJ, Goodman LJ, Hall EJ, Menzel HG, Parnell CJ, Rassow J, et al. Report 45. J Int Commission Radiat Units Measure. (2016) os23:NP. doi: 10.1093/jicru/os23.2.Report45
55. Duffy D, Rouilly V, Libri V, Hasan M, Beitz B, David M, et al. Functional analysis via standardized whole-blood stimulation systems defines the boundaries of a healthy immune response to complex stimuli. Immunity. (2014) 40:436–50. doi: 10.1016/j.immuni.2014.03.002
56. Kleiner G, Marcuzzi A, Zanin V, Monasta L, Zauli G. Cytokine levels in the serum of healthy subjects. Mediat Inflamm. (2013) 2013:434010. doi: 10.1155/2013/434010
57. Köhler J, Ehresmann B, Zeitlin C, Wimmer-Schweingruber R, Hassler D, Reitz G, et al. Measurements of the neutron spectrum in transit to Mars on the Mars Science laboratory. Life Sci Space Res. (2015) 5:6–12. doi: 10.1016/j.lssr.2015.03.001
58. Norbury JW, Schimmerling W, Slaba TC, Azzam EI, Badavi FF, Baiocco G, et al. Galactic cosmic ray simulation at the NASA Space Radiation Laboratory. Life Sci Space Res. (2016) 8:38–51. doi: 10.1016/j.lssr.2016.02.001
59. Guo J, Zeitlin C, Wimmer-Schweingruber R, Hassler DM, Köhler J, Ehresmann B, et al. Measurements of the neutral particle spectra on Mars by MSL/RAD from 2015-11-15 to 2016-01-15. Life Sci Space Res. (2017) 14:12–7. doi: 10.1016/j.lssr.2017.06.001
60. Farber DL. Biochemical signaling pathways for memory T cell recall. Semin Immunol. (2009) 21:84–91. doi: 10.1016/j.smim.2009.02.003
61. Sa Q, Woodward J, Suzuki Y. IL-2 produced by CD8+ immune T cells can augment their IFN-γ production independently from their proliferation in the secondary response to an intracellular pathogen. J Immunol. (2013) 190:2199–207. doi: 10.4049/jimmunol.1202256
62. Cicchese JM, Evans S, Hult C, Joslyn LR, Wessler T, Millar JA, et al. Dynamic balance of pro-and anti-inflammatory signals controls disease and limits pathology. Immunol Rev. (2018) 285:147–67. doi: 10.1111/imr.12671
63. Boyman O, Sprent J. The role of interleukin-2 during homeostasis and activation of the immune system. Nat Rev Immunol. (2012) 12:180–90. doi: 10.1038/nri3156
64. Fiorentino DF, Bond MW, Mosmann T. Two types of mouse T helper cell. IV. Th2 clones secrete a factor that inhibits cytokine production by Th1 clones. J Exp Med. (1989) 170:2081–95. doi: 10.1084/jem.170.6.2081
65. Moore KW, de Waal Malefyt R, Coffman RL, O'Garra A. Interleukin-10 and the interleukin-10 receptor. Ann Rev Immunol. (2001) 19:683–765. doi: 10.1146/annurev.immunol.19.1.683
66. Berry WD, Murphy JD, Smith BA, Taylor GR, Sonnenfeld G. Effect of microgravity modeling on interferon and interleukin responses in the rat. J Interferon Res. (1991) 11:243–9. doi: 10.1089/jir.1991.11.243
67. Alspach E, Lussier DM, Schreiber RD. Interferon γ and its important roles in promoting and inhibiting spontaneous and therapeutic cancer immunity. Cold Spring Harbor Perspect Biol. (2019) 11:a028480. doi: 10.1101/cshperspect.a028480
68. Ye L-L, Wei X-S, Zhang M, Niu Y-R, Zhou Q. The significance of tumor necrosis factor receptor type II in CD8+ regulatory T cells and CD8+ effector T cells. Front Immunol. (2018) 9:583. doi: 10.3389/fimmu.2018.00583
69. Thakur A, Mikkelsen H, Jungersen G. Intracellular pathogens: host immunity and microbial persistence strategies. J Immunol Res. (2019) 2019:1356540. doi: 10.1155/2019/1356540
70. Chancellor JC, Blue RS, Cengel KA, Aunon-Chancellor SM, Rubins KH, Katzgraber HG, et al. Limitations in predicting the space radiation health risk for exploration astronauts. NPJ Microgr. (2018) 4:8. doi: 10.1038/s41526-018-0043-2
71. Cucinotta FA, Durante M. Cancer risk from exposure to galactic cosmic rays: implications for space exploration by human beings. Lancet Oncol. (2006) 7:431–5. doi: 10.1016/S1470-2045(06)70695-7
72. Wilson JW, Townsend LW. A benchmark for galactic cosmic-ray transport codes. Radiat Res. (1988) 114:201–6. doi: 10.2307/3577217
73. Broustas CG, Xu Y, Harken AD, Garty G, Amundson SA. Comparison of gene expression response to neutron and x-ray irradiation using mouse blood. BMC Genomics. (2017) 18:2. doi: 10.1186/s12864-016-3436-1
74. In J. Introduction of a pilot study. Korean J Anesthesiol. (2017) 70:601. doi: 10.4097/kjae.2017.70.6.601
Keywords: radiation in space, immune system, space radiobiology, terrestrial analog, cytokine release assay in vitro, dose rate effect, astronaut health, neutron radiation
Citation: Fisher R, Baselet B, Vermeesen R, Moreels M, Baatout S, Rahiman F, Miles X, Nair S, du Plessis P, Engelbrecht M, Ndimba RJ, Bolcaen J, Nieto-Camero J, de Kock E and Vandevoorde C (2020) Immunological Changes During Space Travel: A Ground-Based Evaluation of the Impact of Neutron Dose Rate on Plasma Cytokine Levels in Human Whole Blood Cultures. Front. Phys. 8:568124. doi: 10.3389/fphy.2020.568124
Received: 31 May 2020; Accepted: 13 August 2020;
Published: 30 September 2020.
Edited by:
Marco Durante, Gesellschaft für Schwerionenforschung (GSI) Helmholtz Center for Heavy Ion Research, GermanyReviewed by:
Alexander Helm, Gesellschaft für Schwerionenforschung (GSI) Helmholtz Center for Heavy Ion Research, GermanyDörthe Schaue, UCLA David Geffen School of Medicine, United States
Lynnette H. Cary, Uniformed Services University of the Health Sciences, United States
Copyright © 2020 Fisher, Baselet, Vermeesen, Moreels, Baatout, Rahiman, Miles, Nair, du Plessis, Engelbrecht, Ndimba, Bolcaen, Nieto-Camero, de Kock and Vandevoorde. This is an open-access article distributed under the terms of the Creative Commons Attribution License (CC BY). The use, distribution or reproduction in other forums is permitted, provided the original author(s) and the copyright owner(s) are credited and that the original publication in this journal is cited, in accordance with accepted academic practice. No use, distribution or reproduction is permitted which does not comply with these terms.
*Correspondence: Randall Fisher, rfisher@tlabs.ac.za