- 1NanoScience Technology Center, University of Central Florida, Orlando, FL, United States
- 2Burnett School of Biomedical Sciences, College of Medicine, University of Central Florida, Orlando, FL, United States
- 3Department of Materials Science and Engineering, University of Central Florida, Orlando, FL, United States
- 4Department of Physics, University of Central Florida, Orlando, FL, United States
The mechanical and structural properties of actin cytoskeleton drive various cellular processes, including structural support of the plasma membrane and cellular motility. Actin monomers assemble into double-stranded helical filaments as well as higher-ordered structures such as bundles and networks. Cells incorporate macromolecular crowding, cation interactions, and actin-crosslinking proteins to regulate the organization of actin bundles. Although the roles of each of these factors in actin bundling have been well-known individually, how combined factors contribute to actin bundle assembly, organization, and mechanics is not fully understood. Here, we describe recent studies that have investigated the mechanisms of how intracellular environmental factors influence actin bundling. This review highlights the effects of macromolecular crowding, cation interactions, and actin-crosslinking proteins on actin bundle organization, structure, and mechanics. Understanding these mechanisms is important in determining in vivo actin biophysics and providing insights into cell physiology.
Introduction
The dynamic assembly of actin monomers into higher-ordered structures such as bundles and networks is vital to many eukaryotic cell functions. Actin bundle mechanics and structure play essential roles in the formation of filopodia [1, 2], structural support of plasma membrane [3], force generation [4], cell division, and cell motility [2, 5, 6]. Recent studies demonstrate that actin bundles can function as mechanosensors displaying mechanical responses to external stimuli and mechanical deformation [7, 8]. Bundle assembly dynamics are tightly regulated by intracellular environmental factors, contributing to changes in cell mechanics as well as physiology.
Actin bundle formation can be achieved by macromolecular crowding, electrostatic interactions, and various actin-crosslinking and/or bundling proteins (Figure 1A) [10–17]. Bundles are formed in highly crowded intracellular environments consisting of various macromolecules and ions that limit available cytoplasmic space [18–20]. The presence of macromolecular crowding promotes steric exclusion (“hard”) and/or non-specific (“soft”) effects [21, 22]. Depletion forces induced by macromolecular crowding lead to bundle formation through excluded volume effects, which can overcome repulsive interaction between negatively charged actin filaments [10, 23, 24]. In comparison, cation interactions result in actin bundle formation through counterion condensation
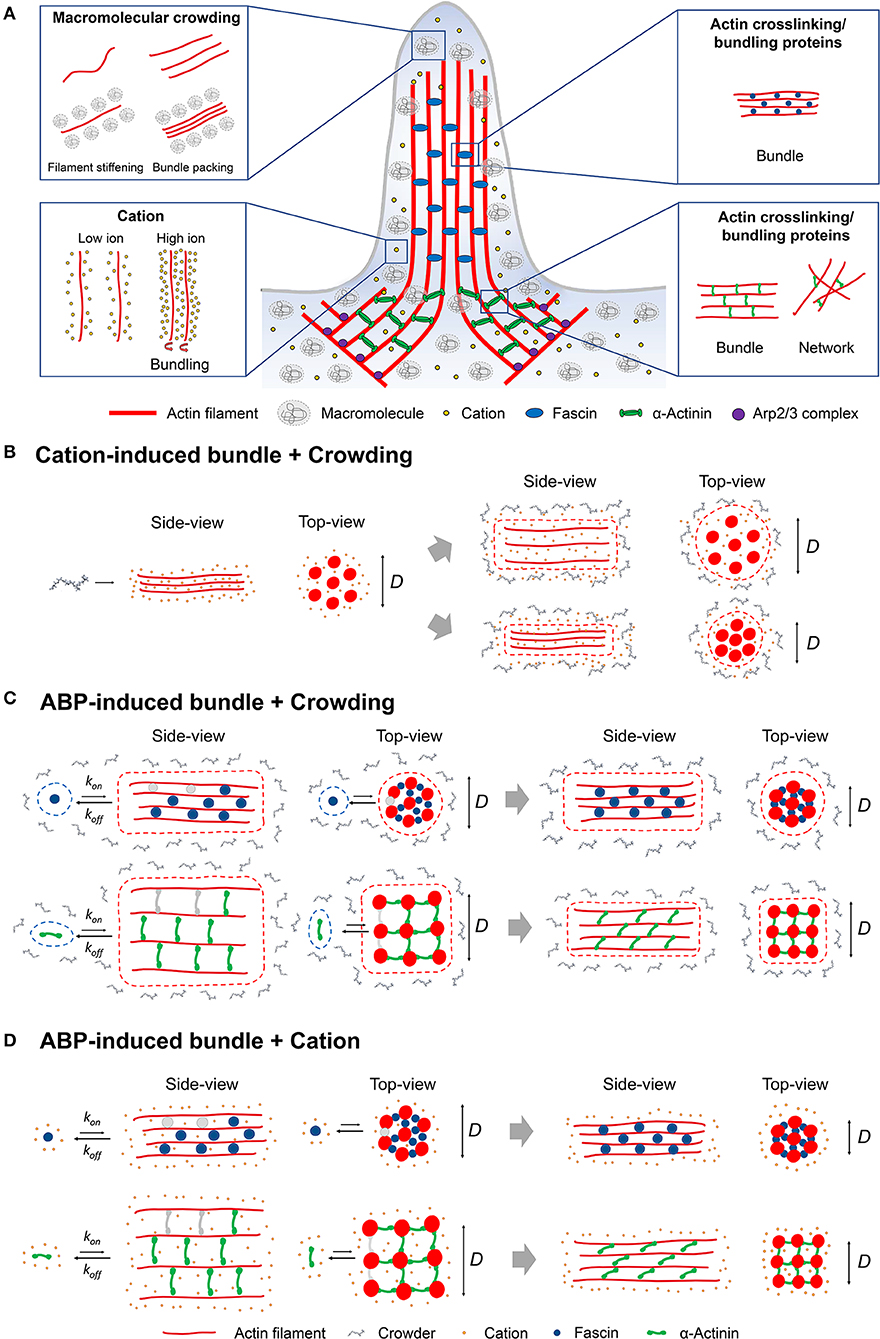
Figure 1. (A) Schematic representation of the various intracellular factors such as macromolecular crowding, cation interactions, and actin-crosslinking/bundling proteins (fascin or α-actinin) that can induce actin bundling at the leading edge of a cell. (B) Cation-induced actin bundle formation in the presence of macromolecular crowding. Potential interactions between cations and crowding may affect the organization of bundles with a different bundle diameter (D). (C) Bundles induced by actin-crosslinking proteins [actin-binding proteins (ABPs)] in crowded environments. Potential competitive interactions between ABPs and crowding may affect the binding of ABPs to filaments as well as bundle organization. Actin bundling proteins bind to actin filament (gray; unbound state, blue and green; bound state) during bundle formation with kon, which is slower than koff due to reduced bundling interactions between actin filament and bundling proteins. Compared with small crosslinkers (e.g., fascin; blue), longer crosslinkers (e.g., α-actinin; green) yield a significant decrease in D since their orientation switches from perpendicular to angled on filament, affecting bundle compactness [9]. (D) ABP-induced bundle formation in the presence of cations can result in modulations to association/dissociation constant kon and koff by cations influencing bundle organization.
[11, 25], similar to DNA condensation [26]. In addition to depletion and electrostatic interactions, cells utilize various actin-crosslinking proteins to form crosslinked bundles or networks [2]. These actin-crosslinking proteins bind actin filaments with different on- and off-rates, influencing the dynamic organization of bundles [15, 27, 28].
The main goal of this review is to summarize major findings on how macromolecular crowding, cation interactions, and actin-crosslinking proteins influence the assembly, organization, and mechanics of actin bundles. In the first part, we describe the effects of depletion and cation interactions on bundle mechanics and structure. In the second part, we introduce the influence of both crowding and cation interactions on the organization and mechanics of bundles crosslinked by actin-binding proteins (ABPs). While the effects of either crowding, cations, or ABPs on actin bundle assembly and mechanics are well-characterized individually, we mainly focus on recent studies demonstrating the potential interplay between these factors on actin-bundling mechanisms.
Effects of Depletion and Cation Interactions on Actin Bundle Mechanics and Structure
Macromolecular crowding induces actin bundle assembly by generating depletion interactions [10, 12, 29, 30] through excluded volume effects [31]. Macromolecular crowding promotes attractive interactions between filaments by reducing the free energy required for bundle formation [10, 32, 33]. Depletion forces maximize the overlap between filaments by minimizing the system free energy [33] and generating sliding of filaments [34]. Crowding has been demonstrated to affect actin filament assembly kinetics [35–37], and filament stability has been evidenced by altered critical concentration of actin [38]. A recent study indicates that crowding enhances filament bending stiffness and alters filament conformations, including filament helical twist [39]. Although the effects of crowding on actin filament assembly are known, how crowding modulates bundle assembly kinetics is not well-understood. Changes to bundle assembly by crowding can potentially influence the mechanical properties of actin bundles.
The mechanical properties of depletion-induced actin bundles have been determined by measuring bundle bending stiffness, elastic moduli, and force between filaments (summarized in Table 1) [12, 50]. Previous in vitro studies have demonstrated that non-specific depletion forces can function as effective crosslinkers [10, 12, 40]. Bundle bending stiffness depends on the number of filaments per bundle and crosslinker effectiveness demonstrated by mechanical modeling as well as previous experimental evidence [12, 51]. Bundle bending stiffness was shown to quadratically scale with the number of filaments within the bundle [12]. Increasing polyethylene glycol (PEG) concentrations result in enhanced local elastic moduli of actin bundles and networks as well as an increase in bundle diameter, which were determined by microrheological analysis (Table 1) [40]. A recent study using optical tweezers determined the force exerted on two bundling filaments [23] by PEG, resulting in weaker bundling (~0.07 ± 0.006 pN) as compared to divalent cations (Mg2+) (~0.20 ± 0.094 pN) (Table 1). Bundles induced by depletion interactions display distinct elastic responses to external forces, such as bending deformations, with minimal evidence of permanent damage [52]. Recently, in vitro motility assay and mathematical modeling have demonstrated that depletion-induced bundles exhibit a critical buckling length, which affects the bundle structure and is dependent on the bundle rigidity and the number of filaments in bundles [53]. Martiel et al. [53] demonstrated that as depletion-induced bundles increase in length, they reach a boundary transition that allows for bundle deformations (i.e., loops) although this boundary can be extended depending on bundle stiffness [53]. The relationship displayed between critical buckling length and persistence length as well as the number of filaments in a bundle is a key determinant in bundle deformation [53].
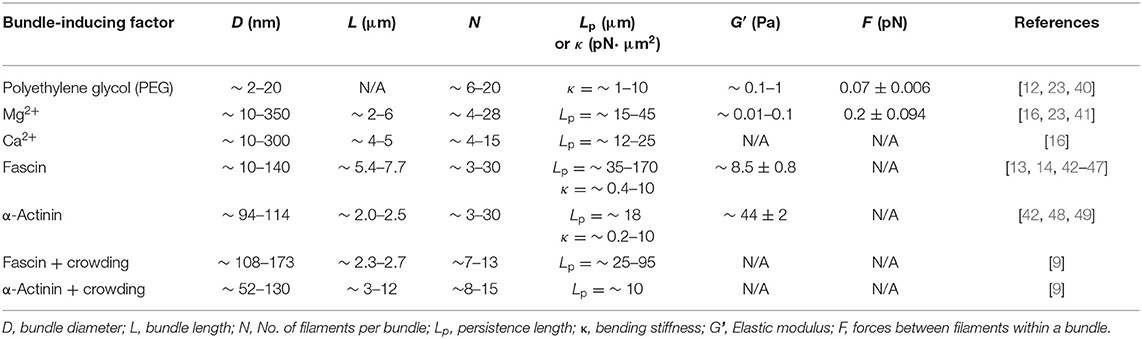
Table 1. The geometric and mechanical properties of actin bundles induced by crowding, cations, and actin-crosslinking proteins.
Cation interactions (non-specific electrostatic and/or specific ion binding) promote bundling of actin filaments, which are linear polyelectrolytes, through a reduction in electrostatic repulsion between filaments above a threshold cation concentration required for actin polymerization [17, 25, 54, 55]. High concentrations of divalent cations (e.g., Mg2+ and Ca2+) were shown to condense actin filaments to bundles and induce over twisting of filaments in bundles, increasing bundle bending persistence length ranging from ~15 to 45 μm (Table 1) [16]. Cation binding modulates the mechanics and structure of actin filaments, potentially affecting bundle mechanics and structure. Hocky et al. [56]. demonstrated, through molecular dynamics (MD) simulations that binding of divalent cations at the “stiffness cation site [57],” along an actin filament, induced the reorganization of the DNase-I binding loop (D-loop). Cation binding at the stiffness site generates a tighter twist angle distribution and affects filament torsional stiffness [56]. The addition of counterions further alters the structure of bundled filaments by changing the contact angle per monomer of the filament helices, obtaining an additional twist of ~3.8° [25]. Recently, Gurmessa et al. [41] have shown the effects of varying concentrations of Mg2+ on the stiffness and elasticity of bundled networks using optical tweezers microrheology and confocal microscopy imaging. They demonstrated that the stiffness, elasticity, and non-linear force response of the actin network increase with increasing concentration of Mg2+ (≥10 mM) (Table 1) [41]. Cation binding at discrete sites along actin filaments can lead to bundle formation and promote modulations to bundle structural properties, such as helical twist [16, 25, 41, 58, 59]. Small-angle x-ray scattering (SAXS) showed that actin filaments condensed into bundles display an over twisting of filaments within the bundles [25]. The observed helical twisting of bundles due to cation interactions was corroborated in a recent study that showed cations specifically bind between filaments at key amino acid residues promoting helical twist of bundles [16]. Cation-induced bundles were shown to retain their secondary structures under high pressures (up to ~5 kbar) and temperatures (up to ~60°C), evidenced by Fourier transform infrared (FTIR) spectroscopy [36].
Although investigations into the effects of cations and crowding on actin bundling have been individually shown, the interplay of both factors together has not been well-established. A previous study demonstrated that the onset of bundling promoted by depletion (PEG) and electrostatic interactions exhibits opposite dependence on cation (K+) concentrations [29]. A possible competition between electrostatic and depletion interactions can modulate the assembly and organization of actin bundles (Figure 1B). Experimental evidence has demonstrated the individual impacts of both crowding and ionic interactions on actin filament or bundle structure [16, 25]. For example, high divalent cation concentrations condense actin filaments to form bundles, resulting in changes to filament helical symmetry [25]. A potential competition between the bending energy of helical filaments and the binding energy of crosslinkers can contribute to finite bundle sizes [60]. We speculate that the concentrations, types, and size of crowding agents and cations contribute to alterations in actin bundle structure by changes in bending and/or binding energy. A recent study on PEG-induced microtubule bundling indicated that cohesive interactions between microtubules depend on the attractive depletion interactions and electrostatic repulsion [61]. Investigations into the opposite effects of depletion and cation interactions have been performed with DNA [62]. Krotova et al. [62] demonstrated competition, upon an increase in salt concentration between entropy and ionic interactions, of DNA undergoing an unfolding transition in crowded environments. These studies illustrate the counteracting effects of crowding and cation interactions on bundling of cytoskeletal biopolymers as well as DNA condensation. Further studies on the combined effects of both crowding and cation interactions are necessary to determine their impacts on actin bundle mechanics and structures.
The Influence of Crowding and Cation Interactions on the Organization and Mechanics of Actin Bundles Crosslinked by Actin-Binding Proteins
Actin-crosslinking and bundling proteins can assemble filaments into higher-ordered structures such as bundles and networks [2, 4, 63]. The size of the crosslinker size, the kinetics of crosslinkers, and the binding affinity of crosslinkers, along with competitive or cooperative interaction between crosslinkers can influence the architecture as well as mechanical properties of actin bundles [15, 27, 28, 42, 51]. The size of actin-crosslinking proteins determines the architecture and compactness of bundles. For example, fascin is an crosslinking protein (diameter ~6 nm), which forms tightly packed bundles, whereas α-actinin is a larger-sized crosslinker (diameter ~35 nm) inducing widely spaced bundles and/or networks [12, 15]. The bending stiffness of fascin- and α-actinin-induced bundles depends on interfilament spacing, supporting an important role of bundle architecture and compactness in bending mechanics of ABP-crosslinked bundles (Table 1) [12, 51]. Binding kinetics (on- and off-rates) and binding affinity of both fascin and α-actinin to filaments have been shown to affect actin bundle assembly and architecture [27, 28]. Competitive interactions between fascin and α-actinin have been shown in a recent study, where fascin-induced bundles, in the presence of α-actinin, were observed to have a reduction in bundle stiffness and filopodia protrusions with varying concentrations of α-actinin [43]. In comparison, combining α-actinin and filamin results in more enhanced elastic moduli of actin filament networks formed by each crosslinker, supporting their cooperative interactions [64].
In a living cell, actin bundles induced by ABPs are formed in a crowded cytoplasm; therefore, it is important to understand how crowding modulates ABP-induced bundling. Changes in filament bending stiffness and conformations in crowded environments [39] can influence interactions between filaments and ABPs, including actin-crosslinking proteins (e.g., fascin and α-actinin) [9] and severing proteins (e.g., gelsolin) [65]. Crowders with different sizes and concentrations [PEG and methylcellulose (MC)] have been shown to affect the organization patterns and potentially nucleation/growth of microtubule bundles cross-linked with microtubule-associated protein (MAP65) [66]. Potential competitive interactions between crowding (PEG, sucrose, and Ficoll) and actin-crosslinking proteins, fascin, and α-actinin, have recently begun to be explored [9]. Macromolecular crowding influences the organization of either fascin or α-actinin bundles by reducing binding interactions between actin filaments and crosslinking proteins (Figure 1C and Table 1), evidenced by fluorescence microscopy and atomic force microscopy imaging along with MD simulations [9]. MD simulations indicated that macromolecular crowding increases interaction energy between fascin or α-actinin and filaments, and reduces the number of hydrogen bonds [9].
Competitive binding of actin-crosslinking proteins, such as fascin and α-actinin, affects their sorting in a size-dependent manner, thereby influencing the actin bundle structure [15]. Furthermore, recent studies suggest that physical confinement has a significant impact on the mechanics and structure of bundles induced by these actin-crosslinking proteins [43, 67]. Giant unilamellar vesicles (GUVs) were used to demonstrate the impacts of enclosed boundary conditions on self-assembly of actin networks and competition between fascin and α-actinin [43]. The physical confinement has a drastic impact on the formation of actin networks, where larger-diameter (>16 μm) GUV resulted in a greater probability of actin network/aster formation and reductions in ring formation, directly driving the sorting of fascin [43] and α-actinin [67]. Live cells can introduce a boundary of lipid bilayers that potentially interact with the formation of networks by ABPs [42, 43, 68]. The effects of macromolecular crowding on the self-organization of actin rings by heavy meromyosin (HMM) and α-actinin in confinement have been previously investigated [69]. Crowding agent MC was shown to hinder the contraction of actin rings formed by either α-actinin or HMM in GUVs [69]. Overall, the encapsulation of the actin cytoskeleton can be a regulatory mechanism that facilitates the reorganization of actin bundled networks and potential interactions with lipid membranes.
Cation interactions impact the conformations of actin-crosslinking proteins as well as actin filaments, potentially influencing the mechanics and structure of ABP-induced bundles and/or networks (Figure 1D). The actin filament-binding domain, calponin-homology (CH) domain, is found in various types of actin-crosslinking proteins such as α-actinin and filamin [70]. Divalent cation binding has been shown to induce structural transitioning of the CH domain, impacting actin bundle formation by ABPs. For example, Pinotsis et al. demonstrated that Ca2+ binding to α-actinin increases the rigidity of α-actinin, leading to the hindrance of actin bundle formation [71]. Cation binding modulates the bending stiffness of actin filaments [57] and the rheological properties of actin networks [72]. Bidone et al. [72] showed that changes in filament rigidity incurred by specific cation binding result in different strain-stiffening responses of actin networks that depend on the flexibility of actin crosslinkers. Overall, these studies indicate that cation interactions with actin filaments and crosslinking proteins are key modulators in bundle formation as well as mechanics.
Summary and Outlook
In this review, we gave an overview of the growing body of work demonstrating how intracellular environmental factors, specifically macromolecular crowding and cation interactions, modulate the assembly, mechanics, and structure of both non-crosslinked actin bundles and ABP-induced bundles. Studies highlighted that both depletion and cation interactions are key players in the tight regulation of actin bundling. Given that actin bundles respond to changes in intracellular environmental factors, it is important to understand (1) whether combined environmental factors act synergistically or competitively to control bundle assembly and (2) how the interactions between actin crosslinkers and crowding and/or cation binding modulate bundle mechanics and structure. Knowledge gained from in vitro studies on actin-bundling mechanisms will enhance our understanding of how complex cellular environments influence actin cytoskeleton organization and mechanics. Future studies will benefit from investigating how the actin cytoskeleton actively responds to local changes in intracellular environments as well as external stimuli shaping its architecture, organization, function, and mechanical properties.
Author Contributions
All authors contributed to the analysis, organizing, writing of the manuscript, commented on, and approved the submitted version.
Conflict of Interest
The authors declare that the research was conducted in the absence of any commercial or financial relationships that could be construed as a potential conflict of interest.
Acknowledgments
The authors would like to thank the financial support provided by the National Science Foundation under Grant No. 1943266 (to EHK) and the National Institute of Allergy and Infectious Diseases of the National Institutes of Health under Award Number R01AI139242, as well as the funds from the University of Central Florida (UCF) awarded to EHK.
References
1. Mogilner A, Rubinstein B. The physics of filopodial protrusion. Biophys J. (2005) 89:782–95. doi: 10.1529/biophysj.104.056515
2. Blanchoin L, Boujemaa-Paterski R, Sykes C, Plastino J. Actin dynamics, architecture, and mechanics in cell motility. Physiol Rev. (2014) 94:235–63. doi: 10.1152/physrev.00018.2013
3. Zsolnay V, Katkar HH, Chou SZ, Pollard TD, Voth GA. Structural basis for polarized elongation of actin filaments. Proc Natl Acad Sci USA. (2020) 117:30458–64. doi: 10.1073/pnas.2011128117
4. De La Cruz EM, Gardel ML. Actin mechanics and fragmentation. J Biol Chem. (2015) 290:17137–44. doi: 10.1074/jbc.R115.636472
5. Mitchison TJ, Cramer LP. Actin-based cell motility and cell locomotion. Cell. (1996) 84:371–9. doi: 10.1016/S0092-8674(00)81281-7
6. Pollard TD, Cooper JA. Actin, a central player in cell shape and movement. Science. (2009) 326:1208–12. doi: 10.1126/science.1175862
7. Narayanan P, Chatterton P, Ikeda A, Ikeda S, Corey DP, Ervasti JM, et al. Length regulation of mechanosensitive stereocilia depends on very slow actin dynamics and filament-severing proteins. Nat Commun. (2015) 6:6855. doi: 10.1038/ncomms7855
8. Rückerl F, Lenz M, Betz T, Manzi J, Martiel J-L, Safouane M, et al. Adaptive response of actin bundles under mechanical stress. Biophys J. (2017) 113:1072–9. doi: 10.1016/j.bpj.2017.07.017
9. Park J, Lee M, Lee B, Castaneda N, Tetard L, Kang EH. Crowding tunes the organization and mechanics of actin bundles formed by crosslinking proteins. FEBS Lett. (2021) 595:26–40. doi: 10.1002/1873-3468.13949
10. Hosek M, Tang JX. Polymer-induced bundling of F-actin and the depletion force. Phys Rev E Stat Nonlin Soft Matter Phys. (2004) 69:051907. doi: 10.1103/PhysRevE.69.051907
11. Angelini TE, Sanders LK, Liang H, Wriggers W, Tang JX, Wong GC. Structure and dynamics of condensed multivalent ions within polyelectrolyte bundles: a combined x-ray diffraction and solid-state NMR study. J Phys Condens Matter. (2005) 17:S1123–35. doi: 10.1088/0953-8984/17/14/001
12. Claessens MM, Bathe M, Frey E, Bausch AR. Actin-binding proteins sensitively mediate F-actin bundle stiffness. Nat Mater. (2006) 5:748–53. doi: 10.1038/nmat1718
13. Jansen S, Collins A, Yang C, Rebowski G, Svitkina T, Dominguez R. Mechanism of actin filament bundling by fascin. J Biol Chem. (2011) 286:30087–96. doi: 10.1074/jbc.M111.251439
14. Takatsuki H, Bengtsson E, Mansson A. Persistence length of fascin-cross-linked actin filament bundles in solution and the in vitro motility assay. Biochim Biophys Acta. (2014) 1840:1933–42. doi: 10.1016/j.bbagen.2014.01.012
15. Winkelman JD, Suarez C, Hocky GM, Harker AJ, Morganthaler AN, Christensen JR, et al. Fascin-and α-actinin-bundled networks contain intrinsic structural features that drive protein sorting. Curr Biol. (2016) 26:2697–706. doi: 10.1016/j.cub.2016.07.080
16. Castaneda N, Zheng T, Rivera-Jacquez HJ, Lee H-J, Hyun J, Balaeff A, et al. Cations modulate actin bundle mechanics, assembly dynamics, and structure. J Phys Chem B. (2018) 122:3826–35. doi: 10.1021/acs.jpcb.8b00663
17. Schnauß J, Händler T, Käs JA. Semiflexible biopolymers in bundled arrangements. Polymers. (2016) 8:274. doi: 10.3390/polym8080274
18. Minton AP. Implications of macromolecular crowding for protein assembly. Curr Opin Struct Biol. (2000) 10:34–9. doi: 10.1016/S0959-440X(99)00045-7
19. Kuznetsova IM, Turoverov KK, Uversky VN. What macromolecular crowding can do to a protein. Int J Mol Sci. (2014) 15:23090–140. doi: 10.3390/ijms151223090
20. Shahid S, Hassan MI, Islam A, Ahmad F. Size-dependent studies of macromolecular crowding on the thermodynamic stability, structure and functional activity of proteins: in vitro and in silico approaches. Biochim Biophys Acta General Subjects. (2017) 1861:178–97. doi: 10.1016/j.bbagen.2016.11.014
21. Sarkar M, Li C, Pielak GJ. Soft interactions and crowding. Biophys Rev. (2013) 5:187–94. doi: 10.1007/s12551-013-0104-4
22. Sarkar M, Lu J, Pielak GJ. Protein crowder charge and protein stability. Biochemistry. (2014) 53:1601–6. doi: 10.1021/bi4016346
23. Streichfuss M, Erbs F, Uhrig K, Kurre R, Clemen AE, Bohm CH, et al. Measuring forces between two single actin filaments during bundle formation. Nano Lett. (2011) 11:3676–80. doi: 10.1021/nl201630y
24. Huber F, Strehle D, Schnauß J, Käs J. Formation of regularly spaced networks as a general feature of actin bundle condensation by entropic forces. New J Phys. (2015) 17:043029. doi: 10.1088/1367-2630/17/4/043029
25. Angelini TE, Liang H, Wriggers W, Wong GC. Like-charge attraction between polyelectrolytes induced by counterion charge density waves. Proc Natl Acad Sci USA. (2003) 100:8634–37. doi: 10.1073/pnas.1533355100
26. Manning GS. Counterion binding in polyelectrolyte theory. Acc Chem Res. (1979) 12:443–9. doi: 10.1021/ar50144a004
27. Courson DS, Rock RS. Actin cross-link assembly and disassembly mechanics for alpha-Actinin and fascin. J Biol Chem. (2010) 285:26350–7. doi: 10.1074/jbc.M110.123117
28. Falzone TT, Lenz M, Kovar DR, Gardel ML. Assembly kinetics determine the architecture of α-actinin crosslinked F-actin networks. Nat Commun. (2012) 3:1–9. doi: 10.1038/ncomms1862
29. Tang JX, Ito T, Tao T, Traub P, Janmey PA. Opposite effects of electrostatics and steric exclusion on bundle formation by F-actin and other filamentous polyelectrolytes. Biochemistry. (1997) 36:12600–7. doi: 10.1021/bi9711386
30. Köhler S, Lieleg O, Bausch AR. Rheological characterization of the bundling transition in F-actin solutions induced by methylcellulose. PLoS ONE. (2008) 3:2736. doi: 10.1371/journal.pone.0002736
31. Minton AP. The influence of macromolecular crowding and macromolecular confinement on biochemical reactions in physiological media. J Biol Chem. (2001) 276:10577–80. doi: 10.1074/jbc.R100005200
32. Lau AW, Prasad A, Dogic Z. Condensation of isolated semi-flexible filaments driven by depletion interactions. Europhys Lett. (2009) 87:48006. doi: 10.1209/0295-5075/87/48006
33. Braun M, Lansky Z, Hilitski F, Dogic Z, Diez S. Entropic forces drive contraction of cytoskeletal networks. BioEssays. (2016) 38:474–81. doi: 10.1002/bies.201500183
34. Ward A, Hilitski F, Schwenger W, Welch D, Lau AW, Vitelli V, et al. Solid friction between soft filaments. Nat Mater. (2015) 14:583–8. doi: 10.1038/nmat4222
35. Drenckhahn D, Pollard TD. Elongation of actin filaments is a diffusion-limited reaction at the barbed end and is accelerated by inert macromolecules. J Biol Chem. (1986) 261:12754–8. doi: 10.1016/S0021-9258(18)67157-1
36. Rosin C, Erlkamp M, Ecken JV, Raunser S, Winter R. (2014). Exploring the stability limits of actin and its suprastructures. Biophys. J. 107:2982–92. doi: 10.1016/j.bpj.2014.11.006
37. Rosin C, Estel K, Halker J, Winter R. Combined effects of temperature, pressure, and co-solvents on the polymerization kinetics of actin. ChemPhysChem. (2015) 16:1379–85. doi: 10.1002/cphc.201500083
38. Frederick KB, Sept D, De La Cruz EM. Effects of solution crowding on actin polymerization reveal the energetic basis for nucleotide-dependent filament stability. J Mol Biol. (2008) 378:540–50. doi: 10.1016/j.jmb.2008.02.022
39. Castaneda N, Lee M, Rivera-Jacquez HJ, Marracino RR, Merlino TR, Kang H. Actin filament mechanics and structure in crowded environments. J Phys Chem B. (2019) 123:2770–9. doi: 10.1021/acs.jpcb.8b12320
40. Tharmann R, Claessens M, Bausch A. Micro-and macrorheological properties of actin networks effectively cross-linked by depletion forces. Biophys J. (2006) 90:2622–7. doi: 10.1529/biophysj.105.070458
41. Gurmessa B, Francis M, Rust MJ, Das M, Ross JL, Robertson-Anderson RM. Counterion crossbridges enable robust multiscale elasticity in actin networks. Phys Rev Res. (2019) 1:013016. doi: 10.1103/PhysRevResearch.1.013016
42. Tseng Y, Kole TP, Lee JS, Fedorov E, Almo SC, Schafer BW, et al. How actin crosslinking and bundling proteins cooperate to generate an enhanced cell mechanical response. Biochem Biophys Res Commun. (2005) 334:183–92. doi: 10.1016/j.bbrc.2005.05.205
43. Bashirzadeh Y, Wubshet NH, Liu AP. Confinement geometry tunes fascin-actin bundle structures and consequently the shape of a lipid bilayer vesicle. Front Molec Biosci. (2020) 7:610277. doi: 10.3389/fmolb.2020.610277
44. Lieleg O, Claessens MM, Heussinger C, Frey E, Bausch AR. Mechanics of bundled semiflexible polymer networks. Phys Rev Lett. (2007) 99:088102. doi: 10.1103/PhysRevLett.99.088102
45. Claessens MM, Semmrich C, Ramos L, Bausch AR. Helical twist controls the thickness of F-actin bundles. Proc Natl Acad Sci USA. (2008) 105:8819–22. doi: 10.1073/pnas.0711149105
46. Ishikawa R, Sakamoto T, Ando T, Higashi-Fujime S, Kohama K. Polarized actin bundles formed by human fascin-1: their sliding and disassembly on myosin II and myosin V in vitro. J Neurochem. (2003) 87:676–85. doi: 10.1046/j.1471-4159.2003.02058.x
47. Haviv L, Gov N, Ideses Y, Bernheim-Groswasser A. Thickness distribution of actin bundles in vitro. Eur Biophys J. (2008) 37:447–54. doi: 10.1007/s00249-007-0236-1
48. Ghosh S, Park J, Thomas M, Cruz E, Cardona O, Kang H, et al. Biophysical characterization of actin bundles generated by the Chlamydia trachomatis Tarp effector. Biochem Biophys Res Commun. (2018) 500:423–8. doi: 10.1016/j.bbrc.2018.04.093
49. Kang B, Jo S, Baek J, Nakamura F, Hwang W, Lee H. Role of mechanical flow for actin network organization. Acta Biomater. (2019) 90:217–24. doi: 10.1016/j.actbio.2019.03.054
50. Schnauß J, Golde T, Schuldt C, Schmidt BS, Glaser M, Strehle D, et al. Transition from a linear to a harmonic potential in collective dynamics of a multifilament actin bundle. Phys Rev Lett. (2016) 116:108102. doi: 10.1103/PhysRevLett.116.108102
51. Bathe M, Heussinger C, Claessens MM, Bausch AR, Frey E. Cytoskeletal bundle mechanics. Biophys J. (2008) 94:2955–64. doi: 10.1529/biophysj.107.119743
52. Strehle D, Mollenkopf P, Glaser M, Golde T, Schuldt C, Käs JA, et al. Single actin bundle rheology. Molecules. (2017) 22:1804. doi: 10.3390/molecules22101804
53. Martiel J-L, Michelot A, Boujemaa-Paterski R, Blanchoin L, Berro J. Force production by a bundle of growing actin filaments is limited by its mechanical properties. Biophys J. (2020) 118:182–92. doi: 10.1016/j.bpj.2019.10.039
54. Tang JX, Wong S, Tran PT, Janmey PA. Counterion induced bundle formation of rodlike polyelectrolytes. Ber Bunsenges Phys Chem. (1996) 100:796–806. doi: 10.1002/bbpc.19961000620
55. Kang H, Bradley MJ, Elam WA, De La Cruz EM. Regulation of actin by ion-linked equilibria. Biophys J. (2013) 105:2621–8. doi: 10.1016/j.bpj.2013.10.032
56. Hocky GM, Baker JL, Bradley MJ, Sinitskiy AV, De La Cruz EM, Voth GA. Cations stiffen actin filaments by adhering a key structural element to adjacent subunits. J Phys Chem B. (2016) 120:4558–67. doi: 10.1021/acs.jpcb.6b02741
57. Kang H, Bradley MJ, Mccullough BR, Pierre A, Grintsevich EE, Reisler E, et al. Identification of cation-binding sites on actin that drive polymerization and modulate bending stiffness. Proc Natl Acad Sci USA. (2012) 109:16923–7. doi: 10.1073/pnas.1211078109
58. Gao M, Berghaus M, Von Der Ecken J, Raunser S, Winter R. Condensation agents determine the temperature–pressure stability of F-actin bundles. Angewandte Chem Int Ed. (2015) 54:11088–92. doi: 10.1002/anie.201504247
59. Ricketts S, Khanal P, Rust M, Das M, Ross J, Robertson-Anderson R. Triggering cation-induced contraction of cytoskeleton networks via microfluidics. J Front Phys. (2020) 2020:596699. doi: 10.3389/fphy.2020.596699
60. Gov NS. Packing defects and the width of biopolymer bundles. Phys Rev E Stat Nonlin Soft Matter Phys. (2008) 78:011916. doi: 10.1103/PhysRevE.78.011916
61. Hilitski F, Ward AR, Cajamarca L, Hagan MF, Grason GM, Dogic Z. Measuring cohesion between macromolecular filaments one pair at a time: depletion-induced microtubule bundling. Phys Rev Lett. (2015) 114:138102. doi: 10.1103/PhysRevLett.114.138102
62. Krotova M, Vasilevskaya V, Makita N, Yoshikawa K, Khokhlov A. DNA compaction in a crowded environment with negatively charged proteins. Phys Rev Lett. (2010) 105:128302. doi: 10.1103/PhysRevLett.105.128302
63. Pollard TD. Actin and actin-binding proteins. Cold Spring Harb Perspect Biol. (2016) 8:a018226. doi: 10.1101/cshperspect.a018226
64. Esue O, Tseng Y, Wirtz D. Alpha-actinin and filamin cooperatively enhance the stiffness of actin filament networks. PLoS ONE. (2009) 4:e4411. doi: 10.1371/journal.pone.0004411
65. Heidings JB, Demosthene B, Merlino TR, Castaneda N, Kang EH. Gelsolin-mediated actin filament severing in crowded environments. Biochem Biophys Res Commun. (2020) 532:548–54. doi: 10.1016/j.bbrc.2020.08.041
66. Sahu S, Herbst L, Quinn R, Ross JL. Crowder and surface effects on self-organization of microtubules. arXiv preprint arXiv, 2009.04669. (2020) 7.
67. Bashirzadeh Y, Redford SA, Lorpaiboon C, Groaz A, Litschel T, Schwille P, et al. Actin crosslinker competition and sorting drive emergent GUV size-dependent actin network architecture. bioRxiv. (2020). doi: 10.1101/2020.10.03.322354
68. Liu AP, Fletcher DA. Actin polymerization serves as a membrane domain switch in model lipid bilayers. Biophys J. (2006) 91:4064–70. doi: 10.1529/biophysj.106.090852
69. Miyazaki M, Chiba M, Eguchi H, Ohki T, Ishiwata S. Cell-sized spherical confinement induces the spontaneous formation of contractile actomyosin rings in vitro. Nat Cell Biol. (2015) 17:480–9. doi: 10.1038/ncb3142
70. Korenbaum E, Rivero F. Calponin homology domains at a glance. J Cell Sci. (2002) 115:3543–5. doi: 10.1242/jcs.00003
71. Pinotsis N, Zielinska K, Babuta M, Arolas JL, Kostan J, Khan MB, et al. Calcium modulates the domain flexibility and function of an α-actinin similar to the ancestral α-actinin. Proc Natl Acad Sci USA. (2020) 117:22101–12. doi: 10.1073/pnas.1917269117
Keywords: actin bundles, macromolecular crowding, cation interactions, actin crosslinker, bending stiffness
Citation: Castaneda N, Park J and Kang EH (2021) Regulation of Actin Bundle Mechanics and Structure by Intracellular Environmental Factors. Front. Phys. 9:675885. doi: 10.3389/fphy.2021.675885
Received: 04 March 2021; Accepted: 13 April 2021;
Published: 27 May 2021.
Edited by:
Yuan Lin, The University of Hong Kong, Hong KongReviewed by:
Naomi Courtemanche, University of Minnesota Twin Cities, United StatesYashar Bashirzadeh, University of Michigan, United States
Copyright © 2021 Castaneda, Park and Kang. This is an open-access article distributed under the terms of the Creative Commons Attribution License (CC BY). The use, distribution or reproduction in other forums is permitted, provided the original author(s) and the copyright owner(s) are credited and that the original publication in this journal is cited, in accordance with accepted academic practice. No use, distribution or reproduction is permitted which does not comply with these terms.
*Correspondence: Ellen Hyeran Kang, ZWxsZW4ua2FuZ0B1Y2YuZWR1
†These authors have contributed equally to this work