- Department of Chemistry, Saint Mary’s University, Halifax, NS, Canada
The advancement of non-invasive quantitative optical diagnosis techniques such as polarization-sensitive second harmonic generation microscopy (PSHG) for diseases such as cancer presents opportunities for improving disease understanding and survival rates. Here, novel and developing techniques in PSHG microscopy applied for the differentiation of cancerous or diseased tissues are presented, including circular dichroism, modulation of laser linear polarization, detection of outgoing linear laser polarization, and double-Stokes Mueller. Typically, initial cancer diagnosis is performed by visual inspection of stained biopsy or surgical resection tissue sections under bright-field microscopy, however, early diagnosis is challenging due to variability in morphological interpretation of the tissues, and because cancer initiation regions can be small and easy to miss. Therefore, pathologists could benefit in identifying cancer on biopsy or surgical resection sections by using unbiased quantitative automated technologies with high spatial resolution and improved disease specificity that can check the entire slide pixel-by-pixel. Second harmonic generation microscopy offers the opportunity to measure ultrastructural alterations in collagenous scaffolds of organ tissues virtually background free on submicron-sized tissue regions. The approach is particularly interesting for cancer diagnosis applications, because during cancer initiation and progression, the collagen in the affected tissue extracellular matrix is often deregulated and becomes disorganized. This mini-review contains a thorough summary of PSHG techniques that have interrogated diseased tissues, and discusses their technical variations and successes in disease discrimination.
Introduction
Second harmonic generation (SHG) or frequency doubling is a nonlinear optical process which occurs efficiently in a microscope when two laser photons of wavelength λ interact with matter to produce light at λ/2 (Figure 1B). Only non-central symmetric materials at both molecular and macromolecular scales can produce SHG and therefore, microcrystalline structures are required for SHG. In animals, most SHG occurs from fibrous collagenous connective tissues or myosin in muscle tissues, both having a non-central microcrystalline structure. Since the SHG is emitted due to the presence and ultrastructure of the muscle or collagen interacting with the laser, no dyes or sample modification procedures are needed, and the signal can be interpreted as a direct indicator of structural sample changes.
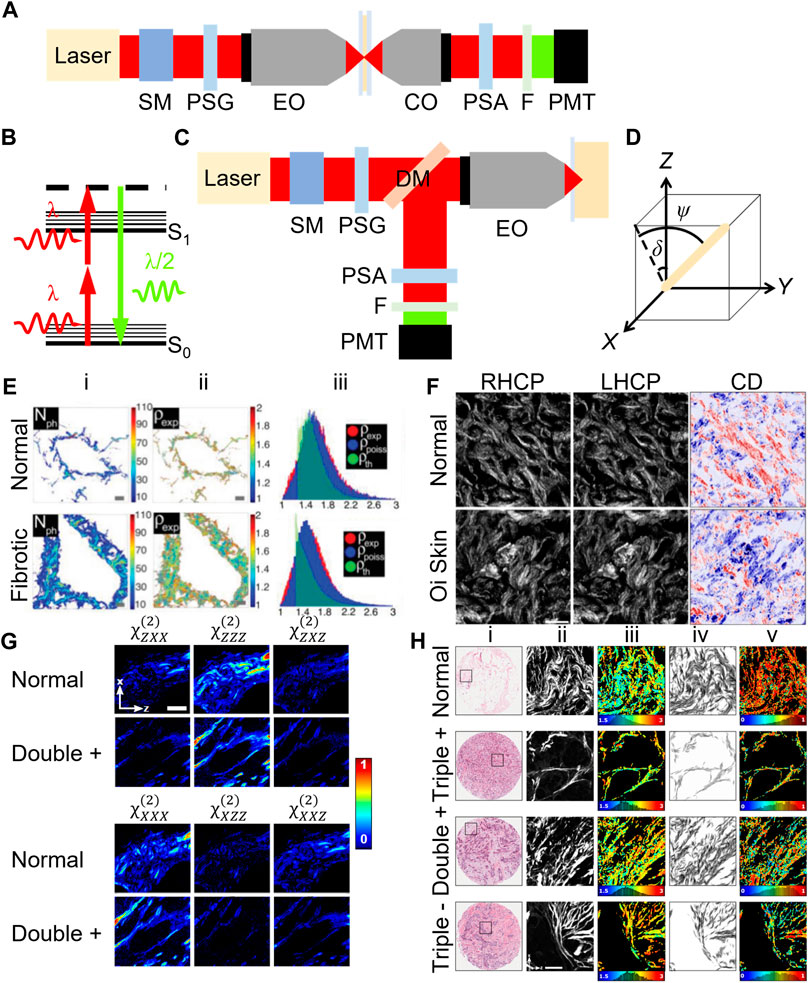
FIGURE 1. Typical microscope schematics for forward- (A) and epi-detection (C) configurations for polarization-sensitive second harmonic generation (SHG) microscopy. The energy state diagram of SHG (B) along with the coordinate system, where Z-X is the image plane and the laser propagates along Y, for an arbitrarily oriented fiber (D) is shown. Abbreviations: SM-scanning mirrors, PSG-polarization state generator, EO-excitation microscope objective, CO-collection microscope objective, PSA-polarization state analyzer, F-filter, PMT-photomultiplier tube detector and DM-dichroic mirror. Example images obtained using PI-SHG (E), CD-SHG (F), DSMP-SHG (G) and PIPO-SHG (H). SHG intensity (E-i), fitted
SHG is energy conserving and consequently does not photobleach, differing significantly from non-parametric processes, such as fluorescence, where absorbed Stokes energy often leads to sample damage and photobleaching. For biological imaging, lasers with wavelength outside of the tissue absorption spectrum are typically chosen, therefore reduced heat is deposited into the sample allowing for long duration functional in vivo studies.
The SHG intensity and polarization can be written:
Historical Interpretation of the PSHG Parameters Pertaining to Collagen
Researchers, Freund et al. [3–6], pioneered the use of SHG to investigate the structure of collagenous biological tissue by utilizing different laser polarizations. They focused a Q-switched Nd:YAG laser onto rat tail tendon, collecting SHG in the forward direction, similar to Figure 1A, but without scan mirrors and at different scattering angles. They found an SHG peak at the 0° scattering angle, indicative of the macroscopic ordered polar structure of collagen, and found that χ(2) of tendon exhibited cylindrical symmetry, since rotation about the tendon axis did not appreciably change SHG parameters. They attributed the signal predominantly to C-N bonds in the amino acids, arguing these are the likely dominant polarizable, noncentrosymmetric and non-mobile candidates. They used a polarization state analyzer (PSA) consisting of a polarizer to directly measure
Noting the important SHG theoretical work of Dick [7], two groups, Plotnikov et al. [8] and Tiaho et al. [9], attributed
Applications of SHG Microscopy for Determining the Ultrastructure of Diseased Collagenous Tissues
Polarization-In SHG Microscopy
Pioneered in 1979, polarization-in SHG (PI-SHG) microscopy utilizes a polarization state generator (PSG) to rotate the laser linear polarization state. One method is to use a half-wave plate (HWP) in a motorized rotating mount, located before the excitation objective lens (Figures 1A,C). An SHG intensity image is recorded at each HWP angle, typically with 10–30 steps in the range 0°–90°. Since SHG is predominantly forward-directed, most epi-detected photons require backscattering, which is less efficient than forward-detection [11], and results in depolarization of a fraction of the SHG. Parameters
PI-SHG microscopy was used to distinguish normal and diseased tissue regions in several tissues (Table 1) with statistical significance, via the χ(2)zzz values of osteosarcoma, breast cancer and melanoma tissues [12], as well as the
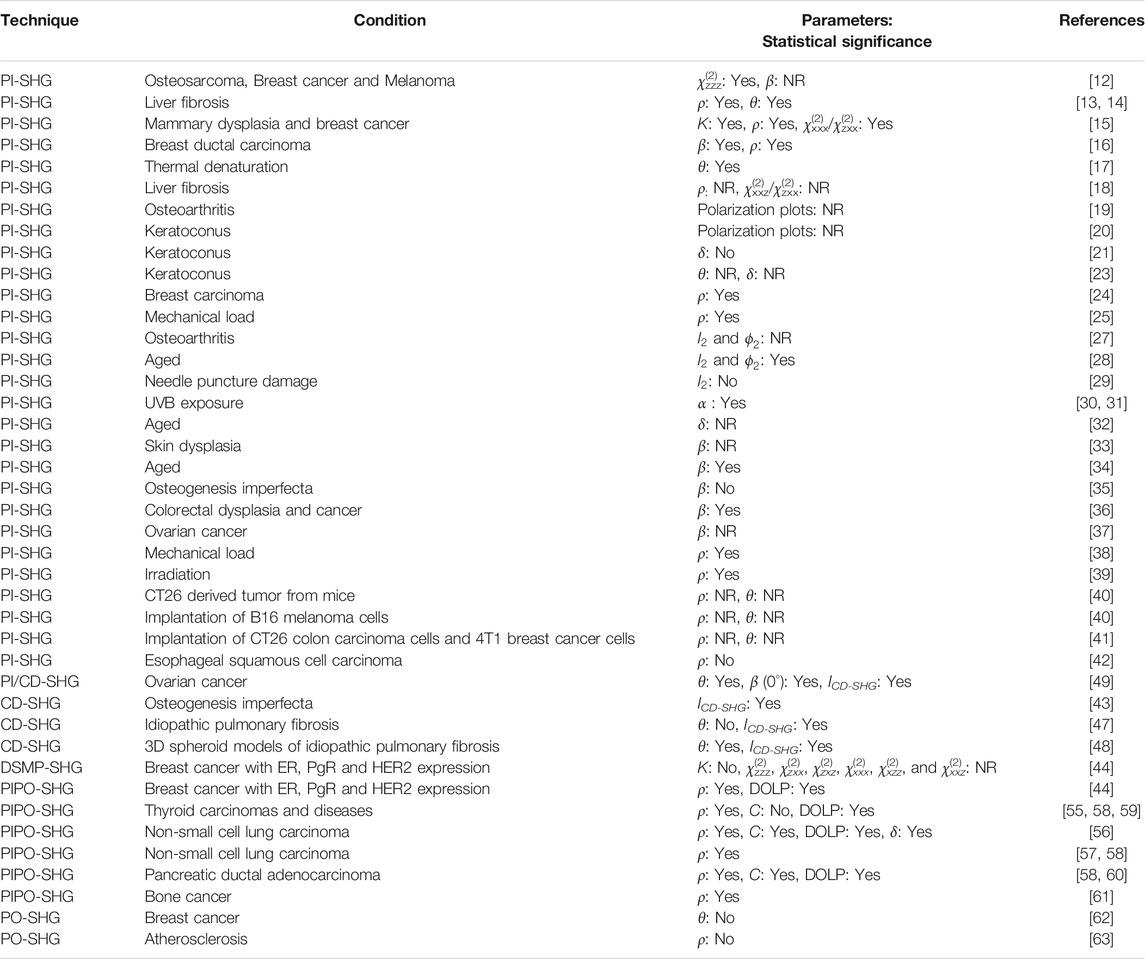
TABLE 1. A summary of the PSHG microscopy techniques used for quantifying the differences in collagen structure in diseased tissue and the corresponding parameters measured with those techniques. The following abbreviation is used NR: Not reported.
To avoid delays due to nonlinear data fitting, a fast Fourier transform (FFT) algorithm has been developed to extract
Several groups have also investigated the excitation anisotropy
Polarization analysis of the outgoing SHG has also been incorporated using a PSA typically located after the collection objective in a forward-detection geometry (Figure 1A), or after a dichroic mirror that separates SHG in epi-detection (Figure 1C). While variable PSAs are reviewed in Double Stokes Mueller Polarimetric SHG (DSMP-SHG) Microscopy and Polarization-In, Polarization-Out SHG (PIPO-SHG) Microscopy sections, stationary PSAs are also used. In one implementation, SHG intensity through a polarizer parallel (
Interestingly, analysis of the PSHG data has also been achieved through Fourier projection of the PSHG image stacks onto two phasor plots referred to as microscopic multiparametric analysis by phasor projection of PSHG (µMAPPS). This technique has been used to compare the
PI-SHG microscopy was also used to image submucosa of esophageal squamous cell carcinoma (ESCC). The
Circular Dichroism Second Harmonic Generation Microscopy
In circular dichroism SHG (CD-SHG) microscopy, two SHG images are obtained using left-handed (IL) and right-handed (IR) circularly polarized laser light, with no PSA in the SHG detection path (Figures 1A,C). The CD-SHG intensity (ICD-SHG) is the normalized difference of the two quantities,
CD-SHG microscopy was applied to determine differences between normal and osteogenesis imperfecta skin tissues as well as idiopathic pulmonary fibrosis human lung tissue where variations in ICD-SHG were statistically significant [43, 47, 48]. Another study used CD-SHG microscopy to find differences between 4 ovarian tissue classifications. The mean ICD-SHG value for normal ovarian tissue was significantly higher than the other tissues [49].
Double Stokes Mueller Polarimetric SHG Microscopy
Double Stokes Mueller polarimetric SHG (DSMP-SHG) microscopy, introduced in 2015 [50, 51], is an alternative method to performing PSHG microscopy. DSMP-SHG microscopy aims to obtain all possible polarization information in the smallest amount of measurements. Stokes vectors are used to describe the laser polarization
DSMP-SHG has the advantage that 6 laboratory frame susceptibility components can be extracted from double Mueller matrix components:
Polarization-In, Polarization-Out SHG Microscopy
Polarization-in, polarization-out SHG (PIPO-SHG) uses a simplified PSA compared to DSMP-SHG as well as the same PSG as in the PI-SHG technique, and hence is typically implemented using a forward-detection geometry (Figure 1A). The PSA measures the linear polarization state of the SHG signal at different angles of the linear polarization of the laser. The simplest PSA consists of a linear polarizer in a motorized rotation mount. SHG images are typically captured with at least 8 PSG angles and 8 PSA angles, hence at least 64 images are recorded for the technique, resulting in ∼20 min acquisition times in comparison to PI-SHG which takes ∼1.5 min [53]. The additional dimensionality of the data is thought to produce higher accuracy fitting, although at the time of writing this manuscript no study has performed the comparison with the other techniques. Fitting of the SHG intensity versus PSA and PSG angles yields δ and
The PIPO-SHG measurements also allow the DOLP of the SHG signal to be obtained, where the SHG light is fully linearly polarized when
A PIPO-SHG microscopy study of pathology slides of lung samples from patients with non-small cell lung carcinoma revealed significant differences in the mean
PIPO-SHG microscopy was also applied to study a microarray slide containing 3 pathological human breast cancer types (Figure 1H) with the overexpression/absence of estrogen receptor, progesterone receptor, and human epidermal growth factor receptor 2: triple +, double +, and triple -. The mean and median
Discussion
Automated digital histopathology using PSHG microscopy is a promising technology for diagnosis of disease in histopathological samples, however, implementation requires fast imaging and data analysis balanced with maintaining high measurement accuracy to obtain diagnosis based on the fewest amount of analyzed pixels. To reduce imaging time due to the different laser polarizations needed, liquid crystal or electro-optic modulators can be used [64–66], or beams with different polarizations can be interleaved. The clever use of fewer input polarizations could also be used, such as in Stokes-Mueller SHG [67] which uses four polarizations in a PI-SHG setup, or advanced polarimeters with multiple detectors can be used for simultaneous detection of the SHG polarization states [68]. In another technique, polarimetry analysis can also be performed using circularly polarized laser excitation, only requiring a single scan [53, 69]. Fourier techniques could be used for faster data analysis [16, 23, 24], while imaging rates can be increased using higher repetition rate pulsed lasers combined with faster scanners such as spinning mirrors, or by increasing the field of view using a wide-field imaging approach [70]. With these developments, it is reasonable that PSHG microscopy may be implemented in a modified hospital pathology slide scanner. Furthermore, since SHG requires no dyes, it can be implemented as an epi-detection setup in an endoscope for in vivo quantitative imaging as a biopsy tool [71–73].
It is evident that PSHG imaging can quantitatively differentiate certain diseased tissues based on their ultrastructure in pathological sample slides however, care must be given to PSHG image quality and the image analysis methods used. For instance, when assessing the quality of PSHG images, it has been found that using SHG intensity as a criterion is only suitable in specific instances [74]. Furthermore, while statistical discrimination based on many samples has been validated in different tissues, the efficacy of diagnosis in individual regions remains unclear and must be addressed. Improved differentiation could be obtained via additional implementation of complementary SHG intensity analysis techniques, such as texture analysis [56, 75–77], the Hough transform [78] and the structure tensor [79, 80]. These analysis techniques could be extended to PSHG
Author Contributions
The authors contributed to manuscript writing (RC, DT) and editing (RC, AJ, MH, DT).
Funding
The research was supported by the Natural Sciences and Engineering Research Council of Canada Discovery Grants Program (RGPIN-2018-05444), Canada’s Research Support Fund, and Saint Mary’s University.
Conflict of Interest
The authors declare that the research was conducted in the absence of any commercial or financial relationships that could be construed as a potential conflict of interest.
Publisher’s Note
All claims expressed in this article are solely those of the authors and do not necessarily represent those of their affiliated organizations, or those of the publisher, the editors and the reviewers. Any product that may be evaluated in this article, or claim that may be made by its manufacturer, is not guaranteed or endorsed by the publisher.
References
1. Kleinman DA. Nonlinear Dielectric Polarization in Optical media. Phys Rev (1962) 126:1977–9. doi:10.1103/PhysRev.126.1977
2. Dailey CA, Burke BJ, Simpson GJ. The General Failure of Kleinman Symmetry in Practical Nonlinear Optical Applications. Chem Phys Lett (2004) 390:8–13. doi:10.1016/j.cplett.2004.03.109
3. Roth S, Freund I. Second Harmonic Generation in Collagen. J Chem Phys (1979) 70:1637–43. doi:10.1063/1.437677
4. Roth S, Freund I. Optical Second-Harmonic Scattering in Rat-Tail Tendon. Biopolymers (1981) 20:1271–90. doi:10.1002/bip.1981.360200613
5. Freund I, Deutsch M. Second-harmonic Microscopy of Biological Tissue. Opt Lett (1986) 11:94–6. doi:10.1364/OL.11.000094
6. Freund I, Deutsch M, Sprecher A. Connective Tissue Polarity. Optical Second-Harmonic Microscopy, Crossed-Beam Summation, and Small-Angle Scattering in Rat-Tail Tendon. Biophysical J (1986) 50:693–712. doi:10.1016/S0006-3495(86)83510-X
7. Dick B. Irreducible Tensor Analysis of Sum- and Difference-Frequency Generation in Partially Oriented Samples. Chem Phys (1985) 96:199–215. doi:10.1016/0301-0104(85)85085-0
8. Plotnikov SV, Millard AC, Campagnola PJ, Mohler WA. Characterization of the Myosin-Based Source for Second-Harmonic Generation from Muscle Sarcomeres. Biophysical J (2006) 90:693–703. doi:10.1529/biophysj.105.071555
9. Tiaho F, Recher G, Rouède D. Estimation of Helical Angles of Myosin and Collagen by Second Harmonic Generation Imaging Microscopy. Opt Express (2007) 15:12286–95. doi:10.1364/OE.15.012286
10. Tuer AE, Akens MK, Krouglov S, Sandkuijl D, Wilson BC, Whyne CM, et al. Hierarchical Model of Fibrillar Collagen Organization for Interpreting the Second-Order Susceptibility Tensors in Biological Tissue. Biophysical J (2012) 103:2093–105. doi:10.1016/j.bpj.2012.10.019
11. Williams RM, Zipfel WR, Webb WW. Interpreting Second-Harmonic Generation Images of Collagen I Fibrils. Biophysical J (2005) 88:1377–86. doi:10.1529/biophysj.104.047308
12. Hompland T, Erikson A, Lindgren M, Lindmo T, de Lange Davies C. Second-harmonic Generation in Collagen as a Potential Cancer Diagnostic Parameter. J Biomed Opt (2008) 13:054050. doi:10.1117/1.2983664
13. Rouède D, Schaub E, Bellanger J-J, Ezan F, Scimeca J-C, Baffet G, et al. Determination of Extracellular Matrix Collagen Fibril Architectures and Pathological Remodeling by Polarization Dependent Second Harmonic Microscopy. Sci Rep (2017) 7:12197. doi:10.1038/s41598-017-12398-0
14. Rouède D, Schaub E, Bellanger J-J, Ezan F, Tiaho F. Wavy Nature of Collagen Fibrils Deduced from the Dispersion of Their Second-Order Nonlinear Optical Anisotropy Parameters ρ. Opt Express (2020) 28:4845–58. doi:10.1364/OE.380089
15. Ambekar R, Lau T-Y, Walsh M, Bhargava R, Toussaint KC. Quantifying Collagen Structure in Breast Biopsies Using Second-Harmonic Generation Imaging. Biomed Opt Express (2012) 3:2021–35. doi:10.1364/BOE.3.002021
16. Tsafas V, Gavgiotaki E, Tzardi M, Tsafa E, Fotakis C, Athanassakis I, et al. Polarization‐dependent Second‐harmonic Generation for Collagen‐based Differentiation of Breast Cancer Samples. J Biophotonics (2020) 13:e202000180. doi:10.1002/jbio.202000180
17. Matteini P, Cicchi R, Ratto F, Kapsokalyvas D, Rossi F, de Angelis M, et al. Thermal Transitions of Fibrillar Collagen Unveiled by Second-Harmonic Generation Microscopy of Corneal Stroma. Biophysical J (2012) 103:1179–87. doi:10.1016/j.bpj.2012.07.055
18. Lin J, Pan S, Zheng W, Huang Z. Polarization-resolved Second-Harmonic Generation Imaging for Liver Fibrosis Assessment without Labeling. Appl Phys Lett (2013) 103:173701. doi:10.1063/1.4826516
19. Brown CP, Houle M-A, Popov K, Nicklaus M, Couture C-A, Laliberté M, et al. Imaging and Modeling Collagen Architecture from the Nano to Micro Scale. Biomed Opt Express (2014) 5:233–43. doi:10.1364/boe.5.000233
20. Ávila FJ, Del Barco O, Bueno JM. Polarimetric Multiphoton Microscopy for the Analysis of Ocular Structures. Opt Pura Apl (2019) 52:1–9. doi:10.7149/OPA.52.1.51013
21. Raoux C, Schmeltz M, Bied M, Alnawaiseh M, Hansen U, Latour G, et al. Quantitative Structural Imaging of Keratoconic Corneas Using Polarization-Resolved SHG Microscopy. Biomed Opt Express (2021) 12:4163–78. doi:10.1364/boe.426145
22. Amat-Roldan I, Psilodimitrakopoulos S, Loza-Alvarez P, Artigas D. Fast Image Analysis in Polarization SHG Microscopy. Opt Express (2010) 18:17209–19. doi:10.1364/OE.18.017209
23. Alizadeh M, Merino D, Lombardo G, Lombardo M, Mencucci R, Ghotbi M, et al. Identifying Crossing Collagen Fibers in Human Corneal Tissues Using pSHG Images. Biomed Opt Express (2019) 10:3875–88. doi:10.1364/boe.10.003875
24. Mercatelli R, Triulzi T, Pavone FS, Orlandi R, Cicchi R. Collagen Ultrastructural Symmetry and its Malignant Alterations in Human Breast Cancer Revealed by Polarization‐resolved Second‐harmonic Generation Microscopy. J Biophotonics (2020) 13:e202000159. doi:10.1002/jbio.202000159
25. Quigley AS, Bancelin S, Deska-Gauthier D, Légaré F, Kreplak L, Veres SP. In Tendons, Differing Physiological Requirements lead to Functionally Distinct Nanostructures. Sci Rep (2018) 8:4409. doi:10.1038/s41598-018-22741-8
26. Duboisset J, Aït-Belkacem D, Roche M, Rigneault H, Brasselet S. Generic Model of the Molecular Orientational Distribution Probed by Polarization-Resolved Second-Harmonic Generation. Phys Rev A (2012) 85:043829. doi:10.1103/PhysRevA.85.043829
27. Mansfield JC, Mandalia V, Toms A, Winlove CP, Brasselet S. Collagen Reorganization in Cartilage under Strain Probed by Polarization Sensitive Second Harmonic Generation Microscopy. J R Soc Interface (2019) 16:20180611. doi:10.1098/rsif.2018.0611
28. Aït-Belkacem D, Guilbert M, Roche M, Duboisset J, Ferrand P, Sockalingum G, et al. Microscopic Structural Study of Collagen Aging in Isolated Fibrils Using Polarized Second Harmonic Generation. J Biomed Opt (2012) 17:080506. doi:10.1117/1.JBO.17.8.080506
29. Wang J-Y, Mansfield JC, Brasselet S, Vergari C, Meakin JR, Winlove CP. Micro-mechanical Damage of Needle Puncture on Bovine Annulus Fibrosus Fibrils Studied Using Polarization-Resolved Second Harmonic Generation(P-SHG) Microscopy. J Mech Behav Biomed Mater (2021) 118:104458. doi:10.1016/j.jmbbm.2021.104458
30. Yasui T, Takahashi Y, Fukushima S, Ogura Y, Yamashita T, Kuwahara T, et al. Observation of Dermal Collagen Fiber in Wrinkled Skin Using Polarization-Resolved Second-Harmonic-Generation Microscopy. Opt Express (2009) 17:912–23. doi:10.1364/oe.17.000912
31. Fukushima S-i., Yonetsu M, Yasui T. Polarization-resolved Second-Harmonic-Generation Imaging of Dermal Collagen Fiber in Prewrinkled and Wrinkled Skins of Ultraviolet-B-Exposed Mouse. J Biomed Opt (2018) 24:031006. doi:10.1117/1.JBO.24.3.031006
32. Tanaka Y, Hase E, Fukushima S, Ogura Y, Yamashita T, Hirao T, et al. Motion-artifact-robust, Polarization-Resolved Second-Harmonic-Generation Microscopy Based on Rapid Polarization Switching with Electro-Optic Pockells Cell and its Application to In Vivo Visualization of Collagen Fiber Orientation in Human Facial Skin. Biomed Opt Express (2014) 5:1099–113. doi:10.1364/boe.5.001099
33. Hristu R, Stanciu SG, Tranca DE, Stanciu GA. Improved Quantification of Collagen Anisotropy with Polarization-Resolved Second Harmonic Generation Microscopy. J Biophoton (2017) 10:1171–9. doi:10.1002/jbio.201600197
34. Miler I, Rabasovic MD, Aleksic M, Krmpot AJ, Kalezic A, Jankovic A, et al. Polarization‐resolved SHG Imaging as a Fast Screening Method for Collagen Alterations during Aging: Comparison with Light and Electron Microscopy. J Biophotonics (2021) 14:e202000362. doi:10.1002/jbio.202000362
35. Nadiarnykh O, Plotnikov S, Mohler WA, Kalajzic I, Redford-Badwal D, Campagnola PJ. Second Harmonic Generation Imaging Microscopy Studies of Osteogenesis Imperfecta. J Biomed Opt (2007) 12:051805. doi:10.1117/1.2799538
36. Birk JW, Tadros M, Moezardalan K, Nadyarnykh O, Forouhar F, Anderson J, et al. Second Harmonic Generation Imaging Distinguishes Both High-Grade Dysplasia and Cancer from normal Colonic Mucosa. Dig Dis Sci (2014) 59:1529–34. doi:10.1007/s10620-014-3121-7
37. Nadiarnykh O, LaComb RB, Brewer MA, Campagnola PJ. Alterations of the Extracellular Matrix in Ovarian Cancer Studied by Second Harmonic Generation Imaging Microscopy. BMC Cancer (2010) 10:1471–2407. doi:10.1186/1471-2407-10-94
38. Gusachenko I, Tran V, Houssen YG, Allain J-M, Schanne-Klein M-C. Polarization-resolved Second-Harmonic Generation in Tendon upon Mechanical Stretching. Biophysical J (2012) 102:2220–9. doi:10.1016/j.bpj.2012.03.068
39. Silva DFT, Gomes ASL, De Campos Vidal B, Ribeiro MS. Birefringence and Second Harmonic Generation on Tendon Collagen Following Red Linearly Polarized Laser Irradiation. Ann Biomed Eng (2013) 41:752–62. doi:10.1007/s10439-012-0720-3
40. Radaelli F, D’Alfonso L, Collini M, Mingozzi F, Marongiu L, Granucci F, et al. μMAPPS: a Novel Phasor Approach to Second Harmonic Analysis for In Vitro-In Vivo Investigation of Collagen Microstructure. Sci Rep (2017) 7:17468. doi:10.1038/s41598-017-17726-y
41. Scodellaro R, Bouzin M, Mingozzi F, D'Alfonso L, Granucci F, Collini M, et al. Whole-Section Tumor Micro-architecture Analysis by a Two-Dimensional Phasor-Based Approach Applied to Polarization-dependent Second Harmonic Imaging. Front Oncol (2019) 9:527. doi:10.3389/fonc.2019.00527
42. Chen W-C, Chen Y-J, Lin S-T, Hung W-H, Chan M-C, Wu I-C, et al. Label-free Characterization of Collagen Fibers in Cancerous Esophagus Tissues Using Ratiometric Nonlinear Optical Microscopy. Exp Biol Med (Maywood) (2020) 245:1213–21. doi:10.1177/1535370220934039
43. Chen X, Raggio C, Campagnola PJ. Second-harmonic Generation Circular Dichroism Studies of Osteogenesis Imperfecta. Opt Lett (2012) 37:3837–9. doi:10.1364/OL.37.003837
44. Golaraei A, Kontenis L, Cisek R, Tokarz D, Done SJ, Wilson BC, et al. Changes of Collagen Ultrastructure in Breast Cancer Tissue Determined by Second-Harmonic Generation Double Stokes-Mueller Polarimetric Microscopy. Biomed Opt Express (2016) 7:4054–68. doi:10.1364/BOE.7.004054
45. Campbell KR, Campagnola PJ. Wavelength-Dependent Second Harmonic Generation Circular Dichroism for Differentiation of Col I and Col III Isoforms in Stromal Models of Ovarian Cancer Based on Intrinsic Chirality Differences. J Phys Chem B (2017) 121:1749–57. doi:10.1021/acs.jpcb.6b06822
46. Golaraei A, Kontenis L, Mirsanaye K, Krouglov S, Akens MK, Wilson BC, et al. Complex Susceptibilities and Chiroptical Effects of Collagen Measured with Polarimetric Second-Harmonic Generation Microscopy. Sci Rep (2019) 9:12488. doi:10.1038/s41598-019-48636-w
47. James DS, Jambor AN, Chang H-Y, Alden Z, Tilbury KB, Sandbo NK, et al. Probing ECM Remodeling in Idiopathic Pulmonary Fibrosis via Second Harmonic Generation Microscopy Analysis of Macro/supramolecular Collagen Structure. J Biomed Opt (2019) 25:014505. doi:10.1117/1.jbo.25.1.014505
48. James DS, Brereton CJ, Davies DE, Jones MG, Campagnola PJ. Examining Lysyl Oxidase-like Modulation of Collagen Architecture in 3D Spheroid Models of Idiopathic Pulmonary Fibrosis via Second-Harmonic Generation Microscopy. J Biomed Opt (2021) 26:066501. doi:10.1117/1.jbo.26.6.066501
49. Campbell KR, Chaudhary R, Handel JM, Patankar MS, Campagnola PJ. Polarization-resolved Second Harmonic Generation Imaging of Human Ovarian Cancer. J Biomed Opt (2018) 23:066501. doi:10.1117/1.JBO.23.6.066501
50. Samim M, Krouglov S, Barzda V. Double Stokes Mueller Polarimetry of Second-Harmonic Generation in Ordered Molecular Structures. J Opt Soc Am B (2015) 32:451–60. doi:10.1364/JOSAB.32.000451
51. Samim M, Krouglov S, Barzda V. Nonlinear Stokes-Mueller Polarimetry. Phys Rev A (2016) 93:013847. doi:10.1103/PhysRevA.93.013847
52. Tuer AE, Krouglov S, Prent N, Cisek R, Sandkuijl D, Yasufuku K, et al. Nonlinear Optical Properties of Type I Collagen Fibers Studied by Polarization Dependent Second Harmonic Generation Microscopy. J Phys Chem B (2011) 115:12759–69. doi:10.1021/jp206308k
53. Alizadeh M, Ghotbi M, Loza-Alvarez P, Merino D. Comparison of Different Polarization Sensitive Second Harmonic Generation Imaging Techniques. Methods Protoc (2019) 2:49. doi:10.3390/mps2020049
54. Golaraei A, Mirsanaye K, Ro Y, Krouglov S, Akens MK, Wilson BC, et al. Collagen Chirality and Three-Dimensional Orientation Studied with Polarimetric Second-Harmonic Generation Microscopy. J Biophotonics (2019) 12:e201800241. doi:10.1002/jbio.201800241
55. Tokarz D, Cisek R, Golaraei A, Asa SL, Barzda V, Wilson BC. Ultrastructural Features of Collagen in Thyroid Carcinoma Tissue Observed by Polarization Second Harmonic Generation Microscopy. Biomed Opt Express (2015) 6:3475–81. doi:10.1364/BOE.6.003475
56. Golaraei A, Mostaço-Guidolin LB, Raja V, Navab R, Wang T, Sakashita S, et al. Polarimetric Second-Harmonic Generation Microscopy of the Hierarchical Structure of Collagen in Stage I-III Non-small Cell Lung Carcinoma. Biomed Opt Express (2020) 11:1851–63. doi:10.1364/boe.387744
57. Golaraei A, Cisek R, Krouglov S, Navab R, Niu C, Sakashita S, et al. Characterization of Collagen in Non-small Cell Lung Carcinoma with Second Harmonic Polarization Microscopy. Biomed Opt Express (2014) 5:3562–7. doi:10.1364/BOE.5.003562
58. Tokarz D, Cisek R, Golaraei A, Krouglov S, Navab R, Niu C, et al. Tumor Tissue Characterization Using Polarization-Sensitive Second Harmonic Generation Microscopy. Proceed SPIE (2015) 9531:95310C. doi:10.1117/12.2180969
59. Tokarz D, Cisek R, Joseph A, Asa SL, Wilson BC, Barzda V. Characterization of Pathological Thyroid Tissue Using Polarization-Sensitive Second Harmonic Generation Microscopy. Lab Invest (2020) 100:1280–7. doi:10.1038/s41374-020-0475-7
60. Tokarz D, Cisek R, Joseph A, Golaraei A, Mirsanaye K, Krouglov S, et al. Characterization of Pancreatic Cancer Tissue Using Multiphoton Excitation Fluorescence and Polarization-Sensitive Harmonic Generation Microscopy. Front Oncol (2019) 9:272. doi:10.3389/fonc.2019.00272
61. Burke M, Golaraei A, Atkins A, Akens M, Barzda V, Whyne C. Collagen Fibril Organization within Rat Vertebral Bone Modified with Metastatic Involvement. J Struct Biol (2017) 199:153–64. doi:10.1016/j.jsb.2017.06.008
62. Han X, Burke RM, Zettel ML, Tang P, Brown EB. Second Harmonic Properties of Tumor Collagen: Determining the Structural Relationship between Reactive Stroma and Healthy Stroma. Opt Express (2008) 16:1846–59. doi:10.1364/oe.16.001846
63. Doras C, Taupier G, Barsella A, Mager L, Boeglin A, Bulou H, et al. Polarization State Studies in Second Harmonic Generation Signals to Trace Atherosclerosis Lesions. Opt Express (2011) 19:15062–8. doi:10.1364/oe.19.015062
64. Dewalt EL, Sullivan SZ, Schmitt PD, Muir RD, Simpson GJ. Polarization-modulated Second Harmonic Generation Ellipsometric Microscopy at Video Rate. Anal Chem (2014) 86:8448–56. doi:10.1021/ac502124v
65. Dow XY, DeWalt EL, Sullivan SZ, Schmitt PD, Ulcickas JRW, Simpson GJ. Imaging the Nonlinear Susceptibility Tensor of Collagen by Nonlinear Optical Stokes Ellipsometry. Biophysical J (2016) 111:1361–74. doi:10.1016/j.bpj.2016.05.055
66. Reiser K, Stoller P, Knoesen A. Three-Dimensional Geometry of Collagenous Tissues by Second Harmonic Polarimetry. Sci Rep (2017) 7:2642. doi:10.1038/s41598-017-02326-7
67. Ávila FJ, Del Barco O, Bueno JM. Quantifying External and Internal Collagen Organization from Stokes-Vector-Based Second Harmonic Generation Imaging Polarimetry. J Opt (2017) 19:105301. doi:10.1088/2040-8986/aa825d
68. Mazumder N, Qiu J, Foreman MR, Romero CM, Hu C-W, Tsai H-R, et al. Polarization-resolved Second Harmonic Generation Microscopy with a Four-Channel Stokes-Polarimeter. Opt Express (2012) 20:14090–9. doi:10.1364/OE.20.014090
69. Psilodimitrakopoulos S, Loza-Alvarez P, Artigas D. Fast Monitoring of Iin-Vvivo Conformational Changes in Myosin Using Single Scan Polarization-SHG Microscopy. Biomed Opt Express (2014) 5:4362–73. doi:10.1364/BOE.5.004362
70. Zhao H, Cisek R, Karunendiran A, Tokarz D, Stewart BA, Barzda V. Live Imaging of Contracting Muscles with Wide-Field Second Harmonic Generation Microscopy Using a High Power Laser. Biomed Opt Express (2019) 10:5130–5. doi:10.1364/boe.10.005130
71. Fu L, Gu M. Polarization Anisotropy in Fiber-Optic Second Harmonic Generation Microscopy. Opt Express (2008) 16:5000–6. doi:10.1364/oe.16.005000
72. Bao H, Boussioutas A, Jeremy R, Russell S, Gu M. Second Harmonic Generation Imaging via Nonlinear Endomicroscopy. Opt Express (2010) 18:1255–60. doi:10.1364/oe.18.001255
73. Ducourthial G, Leclerc P, Mansuryan T, Fabert M, Brevier J, Habert R, et al. Development of a Real-Time Flexible Multiphoton Microendoscope for Label-free Imaging in a Live Animal. Sci Rep (2015) 5:18303. doi:10.1038/srep18303
74. Stanciu SG, Ávila FJ, Hristu R, Bueno JM. A Study on Image Quality in Polarization-Resolved Second Harmonic Generation Microscopy. Sci Rep (2017) 7:15476. doi:10.1038/s41598-017-15257-0
75. Pouli D, Genega EM, Sullivan TB, Rieger-Christ KM, Wright V, Georgakoudi I, et al. Two-photon Images Reveal Unique Texture Features for Label-free Identification of Ovarian Cancer Peritoneal Metastases. Biomed Opt Express (2019) 10:4479–88. doi:10.1364/boe.10.004479
76. Mostaço-Guidolin LB, Osei ET, Ullah J, Hajimohammadi S, Fouadi M, Li X, et al. Defective Fibrillar Collagen Organization by Fibroblasts Contributes to Airway Remodeling in Asthma. Am J Respir Crit Care Med (2019) 200:431–43. doi:10.1164/rccm.201810-1855OC
77. Gant KL, Jambor AN, Li Z, Rentchler EC, Weisman P, Li L, et al. Evaluation of Collagen Alterations in Early Precursor Lesions of High Grade Serous Ovarian Cancer by Second Harmonic Generation Microscopy and Mass Spectrometry. Cancers (2021) 13:2794. doi:10.3390/cancers13112794
78. Bueno JM, Ávila FJ, Hristu R, Stanciu SG, Eftimie L, Stanciu GA. Objective Analysis of Collagen Organization in Thyroid Nodule Capsules Using Second Harmonic Generation Microscopy Images and the Hough Transform. Appl Opt (2020) 59:6925–31. doi:10.1364/ao.393721
79. Ávila FJ, Bueno JM. Analysis and Quantification of Collagen Organization with the Structure Tensor in Second Harmonic Microscopy Images of Ocular Tissues. Appl Opt (2015) 54:9848–54. doi:10.1364/AO.54.009848
80. Bueno JM, Ávila FJ, Martínez-García MC. Quantitative Analysis of the Corneal Collagen Distribution after In Vivo Cross-Linking with Second Harmonic Microscopy. Biomed Res Int (2019) 2019:3860498. doi:10.1155/2019/3860498
81. Artigas D, Merino D, Polzer C, Loza-Alvarez P. Sub-diffraction Discrimination with Polarization-Resolved Two-Photon Excited Fluorescence Microscopy. Optica (2017) 4:911–8. doi:10.1364/optica.4.000911
Keywords: cancer, optical pathology, medical imaging, nonlinear optical polarimetry, nonlinear optical microscopy
Citation: Cisek R, Joseph A, Harvey M and Tokarz D (2021) Polarization-Sensitive Second Harmonic Generation Microscopy for Investigations of Diseased Collagenous Tissues. Front. Phys. 9:726996. doi: 10.3389/fphy.2021.726996
Received: 17 June 2021; Accepted: 10 August 2021;
Published: 30 August 2021.
Edited by:
Nirmal Mazumder, Manipal Academy of Higher Education, IndiaReviewed by:
Stefan G. Stanciu, Politehnica University of Bucharest, RomaniaMehdi Alizadeh, Vilnius University, Lithuania
Francisco Avila, Universidad de Zaragoza de, Spain
Copyright © 2021 Cisek, Joseph, Harvey and Tokarz. This is an open-access article distributed under the terms of the Creative Commons Attribution License (CC BY). The use, distribution or reproduction in other forums is permitted, provided the original author(s) and the copyright owner(s) are credited and that the original publication in this journal is cited, in accordance with accepted academic practice. No use, distribution or reproduction is permitted which does not comply with these terms.
*Correspondence: Danielle Tokarz, ZGFuaWVsbGUudG9rYXJ6QHNtdS5jYQ==