- 1Department of Physics, Arizona State University, Tempe, AZ, United States
- 2Division of Display and Semiconductor Physics, Korea University, Sejong, South Korea
- 3Materials Science Division, Argonne National Laboratory, Lemont, IL, United States
- 4Departamento de Física Aplicada, Facultade de Física, Universidade de Santiago de Compostela, Santiago de Compostela, Spain
- 5Instituto de Materiais (iMATUS), Universidade de Santiago de Compostela, Santiago de Compostela, Spain
- 6Department of Physics and Astronomy, University of California, Davis, Davis, CA, United States
The discovery of superconductivity in thin films (∼10 nm) of infinite-layer hole-doped NdNiO2 has invigorated the field of high temperature superconductivity research, reviving the debate over contrasting views that nickelates that are isostructural with cuprates are either 1) sisters of the high temperature superconductors, or 2) that differences between nickel and copper at equal band filling should be the focus of attention. Each viewpoint has its merits, and each has its limitations, suggesting that such a simple picture must be superseded by a more holistic comparison of the two classes. Several recent studies have begun this generalization, raising a number of questions without suggesting any consensus. In this paper, we organize the findings of the electronic structures of n-layered NiO2 materials (n = 1 to ∞) to outline (ir)regularities and to make comparisons with cuprates, with the hope that important directions of future research will emerge.
1 Background
After much synthesis and characterization of low-valence layered nickelates over three decades [1–7], superconductivity was finally observed [8] in hole-doped
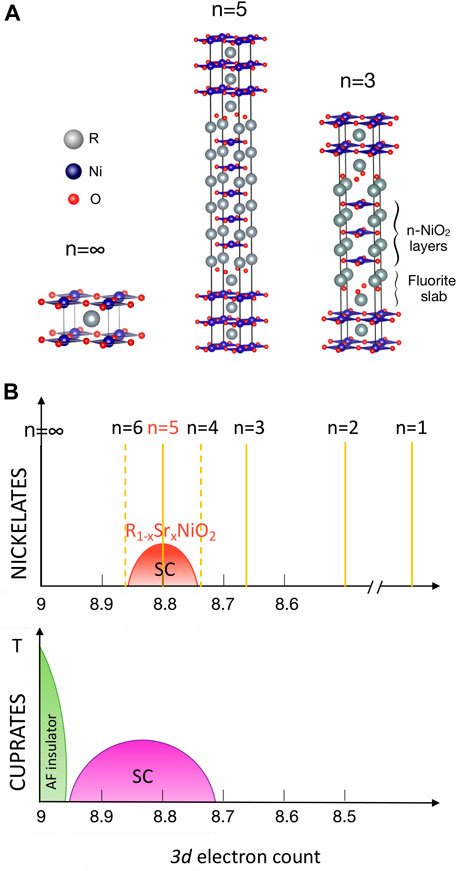
FIGURE 1. (A) Crystal structures of the n = ∞, n = 5, and n = 3 layered nickelates highlighting the presence of the n-NiO2 layers and the fluorite blocking slabs present in the n ≠ ∞ materials.
Some overviews on experimental and theoretical findings in this family of materials have been recently published [45–48]. In this paper, we focus on the electronic structure of layered nickelates, confining ourselves to materials with the basic infinite-layer structure: n square planar NiO2 layers each separated by an
2 From ∞ to One
2.1 “Infinite-Layer” n = ∞ Nickelate:
In parent
Noteworthy differences from cuprates were already reflected in early electronic structure calculations as well [51, 52]. For the parent material
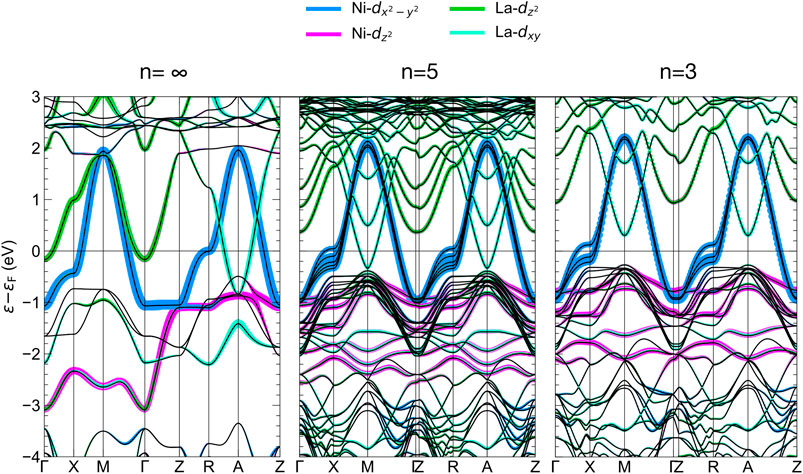
FIGURE 2. DFT band structures in the paramagnetic state for the n = ∞, n = 5, and n = 3 layered nickelates. The two types of Ni eg bands are highlighted, as well as the two relevant
Besides the presence of
The doped holes tend to be on the Ni sites, as opposed to cuprates where they tend to reside on the oxygen sites. Recent DFT + DMFT Ni 2p3/2 core-level XPS, XAS, and RIXS calculations (consistent with available core-level spectroscopies) indeed confirm that the Ni-O hybridization does not play an important role in connection with doping, implying that the physics of NdNiO2 is well described by a single-band Hubbard model [56]. This in turn brings up the issue of the nature of the doped holes on the Ni sites. That is, do they behave as effective d8 dopants, and if so, is d8 high-spin (S = 1) or low-spin (S = 0)? If the former, then these materials would fall in the category of Hund’s metals, and thus would deviate substantially from cuprates. DMFT calculations are consistent with this picture as they systematically favor high-spin d8 (S = 1) states [40, 57–60]. DFT calculations point instead towards a cuprate-like low-spin (S = 0) picture due to the large crystal-field splitting of the eg states in a square planar environment [53]. Along these lines, impurity calculations show that in the NiO2 layers a Zhang-Rice singlet (like in CuO2) is indeed favored upon hole-doping [35]. Further, cluster calculations find that hole doping distributes over the entire cluster, in contrast to local S = 1 states [61].
Because of their lower degree of p − d hybridization, the superexchange in
Discussing the origin of superconductivity in
2.2 The Superconducting n = 5 Material
Recently, a second superconducting member has been found in the
In terms of its electronic structure [73], the n = 5 material is intermediate between cuprate-like and n = ∞-like behavior. From DFT calculations, the charge-transfer energy of Nd6Ni5O12 is ∼ 4.0 eV. This reduced energy compared to the undoped infinite-layer material means that the Ni-3d states are not as close in energy to the Nd-5d states, consistent with the presence of a pre-peak in the oxygen K-edge (similar to what happens with Sr-doped NdNiO2 [53]). As a consequence, the electron pockets arising from the Nd-5d states are significantly smaller than those in the infinite-layer material (see Figure 2). This reduced pocket size along with the large hole-like contribution from the Ni-3d states is consistent with experiment in that the Hall coefficient remains positive at all temperatures, with a semiconductor-like temperature dependence reminiscent of under- and optimally-doped layered cuprates. Aside from the appearance of these small Nd-derived pockets at the zone corners, the Fermi surface of Nd6Ni5O12 is analogous to that of multilayer cuprates with one electron-like and four hole-like
2.3 The n = 3 Material, the Next Superconducting Member of the Series?
The materials discussed above can be put into the context of earlier studies of bulk reduced RP phases with n = 2, 3 NiO2 layers [5, 74–76], separated by fluorite
The n = 3 member of the series,
The difference between La and Pr trilayer materials could be due to the reduced volume associated with Pr (one of the motivations for the authors of Ref. [8] to study Sr-doped NdNiO2 rather than Sr-doped LaNiO2). The Ni spin state and metal versus insulator character have indeed been calculated to be sensitive to modest pressure [77]. Another factor is possible mixed valency of Pr as observed in cuprates (though Pr-M edge data on Pr4Ni3O8 did not indicate mixed valent behavior [75]). Because of its decreased charge-transfer energy relative to n = 5, the rare-earth derived pockets no longer occur [84] (see Figure 2). This lack of
If superconductivity were to occur, one might hope for a higher Tc as has indeed been predicted via t − J model calculations [87]. Recent RIXS measurements [81], though, and find a superexchange value for n = 3 nearly the same as that reported for the infinite-layer material. This suggests the possibility that Tc in the whole nickelate family may be confined to relatively low temperatures compared to the cuprates. The similar value of the superexchange for n = ∞ and n = 3 is somewhat of a puzzle. Though their tpd hoppings are very similar, the difference in the charge-transfer energy should have resulted in a larger superexchange for n = 3. The fact that it is not larger is one of the intriguing questions to be resolved in these low valence layered nickelates.
2.4 The n = 2 Material
The n = 2 member of the series, La3Ni2O6, has been synthesized and studied as well [5, 88]. In terms of filling, it lies further away from optimal d-filling, being nominally Ni1.5+: d8.5. Experimentally, it is a semiconductor with no trace of a transition occurring at any temperature, although NMR data suggest that the AFM correlations are similar to those of the n = 3 material. Electronic structure studies [80] have predicted its ground state to have a charge-ordered pattern with Ni2+ cations in a low-spin state and the Ni1+: d9 cations forming a S = 1/2 checkerboard pattern. This charge-ordering between S = 1/2 Ni1+: d9 and non-magnetic Ni2+: d8 cations is similar to the situation in the n = 3 material [80]. Calculations suggest that it is quite general in these layered nickelates that the Ni2+ cations in this square-planar environment are non-magnetic. This has been shown by ab initio calculations to be the case also with the Ni2+ dopants in the
2.5 The n = 1 Case
The long-known
Valence counting indicates Ni2+: d8, so a half-filled eg manifold. Conventional expectations are either 1) both 3d holes are in the
3 Outlook
While this new nickelate family seems to be emerging as its own class of superconductors, its connections to cuprates (crystal and electronic structures, formal d count in the superconducting region, and AFM correlations) retain a focus on similarities between the two classes. Apart from the obvious structural analogy, the cuprate-motivated prediction of optimal d8.8 filling has been realized in two nickelate materials, one achieved through chemical doping, and the other by layering dimensionality. In this context, the (so far) little studied n = 6 and n = 4 members of the series [73] may provide some prospect for superconductivity. Oxygen-reduced samples of these materials are so far lacking (though the n = 4 member of the RP series has been epitaxially grown [93]), and even if they are synthesized, they might require additional chemical tuning to achieve superconductivity. They share a similar electronic structure to the n = 5 material, but with slightly different nominal filling of the 3d bands [73]. Calculations show that as n decreases from n = ∞ to n = 3, the cuprate-like character increases, with the charge-transfer energy decreasing along with the self-doping effect from the rare earth 5d states. In contrast, the particular n = 1 member discussed above seems distinct from other nickelates, and provides a different set of questions in the context of quantum materials [91, 92].
Author Contributions
All authors listed have made a substantial, direct, and intellectual contribution to the work and approved it for publication.
Funding
AB was supported by the United States National Science Foundation, Grant No. DMR 2045826. K-WL was supported by the National Research Foundation of Korea, and Grant No. NRF2019R1A2C1009588. MN was supported by the Materials Sciences and Engineering Division, Basic Energy Sciences, Office of Science, United States Department of Energy. VP acknowledges support from the MINECO of Spain through the project PGC2018-101 334-BC21. WP acknowledges support from United States National Science Foundation, Grant No. DMR 1607139.
Conflict of Interest
The authors declare that the research was conducted in the absence of any commercial or financial relationships that could be construed as a potential conflict of interest.
Publisher’s Note
All claims expressed in this article are solely those of the authors and do not necessarily represent those of their affiliated organizations, or those of the publisher, the editors and the reviewers. Any product that may be evaluated in this article, or claim that may be made by its manufacturer, is not guaranteed or endorsed by the publisher.
References
1. Greenblatt M. Ruddlesden-Popper Lnn+1NinO3n+1 Nickelates: Structure and Properties. Curr Opin Solid State Mater Sci (1997) 2:174–83. doi:10.1016/s1359-0286(97)80062-9
2. Hayward MA, Green MA, Rosseinsky MJ, Sloan J. Sodium Hydride as a Powerful Reducing Agent for Topotactic Oxide Deintercalation: Synthesis and Characterization of the Nickel(I) Oxide LaNiO2. J Am Chem Soc (1999) 121:8843–54. doi:10.1021/ja991573i
3. Crespin M, Levitz P, Gatineau L. Reduced Forms of LaNiO3 perovskite. Part 1.-Evidence for New Phases: La2Ni2O5 and LaNiO2. J Chem Soc Faraday Trans 2 (1983) 79:1181–94. doi:10.1039/f29837901181
4. Crespin M, Isnard O, Dubois F, Choisnet J, Odier P. LaNiO2: Synthesis and Structural Characterization. J Solid State Chem (2005) 178:1326–34. doi:10.1016/j.jssc.2005.01.023
5. Poltavets VV, Lokshin KA, Dikmen S, Croft M, Egami T, Greenblatt M. La3Ni2O6: A New Double T'-type Nickelate with Infinite Ni1+/2+O2 Layers. J Am Chem Soc (2006) 128:9050–1. doi:10.1021/ja063031o
6. Kawai M, Matsumoto K, Ichikawa N, Mizumaki M, Sakata O, Kawamura N, et al. Orientation Change of an Infinite-Layer Structure LaNiO2 Epitaxial Thin Film by Annealing with CaH2. Cryst Growth Des (2010) 10:2044–6. doi:10.1021/cg100178y
7. Ikeda A, Manabe T, Naito M. Improved Conductivity of Infinite-Layer LaNiO2 Thin Films by Metal Organic Decomposition. Physica C: Superconductivity (2013) 495:134–40. doi:10.1016/j.physc.2013.09.007
8. Li D, Lee K, Wang BY, Osada M, Crossley S, Lee HR, et al. Superconductivity in an Infinite-Layer Nickelate. Nature (2019) 572:624–7. doi:10.1038/s41586-019-1496-5
9. Zeng SW, Li CJ, Chow LE, Cao Y, Zhang ZT, Tang CS, et al. Superconductivity In Infinite-Layer Lanthanide Nickelates. arXiv:2105.13492 (2021).
10. Osada M, Wang BY, Goodge BH, Harvey SP, Lee K, Li D, et al. Nickelate Superconductivity without Rare‐Earth Magnetism: (La,Sr)NiO2. Adv Mater (2021) 33:2104083. doi:10.1002/adma.202104083
11. Osada M, Wang BY, Goodge BH, Lee K, Yoon H, Sakuma K, et al. A Superconducting Praseodymium Nickelate with Infinite Layer Structure. Nano Lett (2020) 20:5735–40. doi:10.1021/acs.nanolett.0c01392
12. Li D, Wang BY, Lee K, Harvey SP, Osada M, Goodge BH, et al. Superconducting Dome in Nd1-xSrxNiO2 Infinite Layer Films. Phys Rev Lett (2020) 125:027001. doi:10.1103/physrevlett.125.027001
13. Zeng S, Tang CS, Yin X, Li C, Li M, Huang Z, et al. Phase Diagram and Superconducting Dome of Infinite-Layer Nd1−xSrxNiO2 Thin Films. Phys Rev Lett (2020) 125:147003. doi:10.1103/physrevlett.125.147003
14. Norman MR. Entering the Nickel Age of Superconductivity. Physics (2020) 13:85. doi:10.1103/physics.13.85
15. Pickett WE. The Dawn of the Nickel Age of Superconductivity. Nat Rev Phys (2021) 3:7–8. doi:10.1038/s42254-020-00257-3
16. Lee K, Goodge BH, Li D, Osada M, Wang BY, Cui Y, et al. Aspects of the Synthesis of Thin Film Superconducting Infinite-Layer Nickelates. APL Mater (2020) 8:041107. doi:10.1063/5.0005103
17. Goodge BH, Li D, Lee K, Osada M, Wang BY, Sawatzky GA, et al. Doping Evolution of the Mott-Hubbard Landscape in Infinite-Layer Nickelates. Proc Natl Acad Sci USA (2021) 118:e2007683118. doi:10.1073/pnas.2007683118
18. Hepting M, Li D, Jia CJ, Lu H, Paris E, Tseng Y, et al. Electronic Structure of the Parent Compound of Superconducting Infinite-Layer Nickelates. Nat Mater (2020) 19:381–5. doi:10.1038/s41563-019-0585-z
19. Fu Y, Wang L, Cheng H, Pei S, Zhou X, Chen J, et al. Core-Level X-Ray Photoemission And Raman Spectroscopy Studies On Electronic Structures In Mott-Hubbard Type Nickelate Oxide NdNiO2. arXiv:1911.03177 (2019).
20. Li Q, He C, Si J, Zhu X, Zhang Y, Wen H-H. Absence of Superconductivity in Bulk Nd1−xSrxNiO2. Commun Mater (2020) 1:16. doi:10.1038/s43246-020-0018-1
21. Wang B-X, Zheng H, Krivyakina E, Chmaissem O, Lopes PP, Lynn JW, et al. Synthesis and characterization of bulk Nd1-xSrxNiO2 and Nd1-xSrxNiO3. Phys Rev Mater (2020) 4:084409. doi:10.1103/physrevmaterials.4.084409
22. Gu Q, Li Y, Wan S, Li H, Guo W, Yang H, et al. Single Particle Tunneling Spectrum of Superconducting Nd1-xSrxNiO2 Thin Films. Nat Commun (2020) 11:6027. doi:10.1038/s41467-020-19908-1
23. Jiang P, Si L, Liao Z, Zhong Z. Electronic Structure of Rare-Earth Infinite-Layer RNiO2(R=La,Nd). Phys Rev B (2019) 100:201106. doi:10.1103/physrevb.100.201106
24. Liu Z, Ren Z, Zhu W, Wang Z, Yang J. Electronic and Magnetic Structure of Infinite-Layer NdNiO2: Trace of Antiferromagnetic Metal. Npj Quan Mater. (2020) 5:31. doi:10.1038/s41535-020-0229-1
25. Nomura Y, Hirayama M, Tadano T, Yoshimoto Y, Nakamura K, Arita R. Formation of a Two-Dimensional Single-Component Correlated Electron System and Band Engineering in the Nickelate Superconductor NdNiO2. Phys Rev B (2019) 100:205138. doi:10.1103/physrevb.100.205138
26. Wu X, Di Sante D, Schwemmer T, Hanke W, Hwang HY, Raghu S, et al. Robust dx2-y2-wave Superconductivity Of Infinite-Layer Nickelates. Phys Rev B (2020) 101:060504. doi:10.1103/physrevb.101.060504
27. Choi M-Y, Lee K-W, Pickett WE. Role of 4f States in Infinite-Layer NdNiO2. Phys Rev B (2020) 101:020503. doi:10.1103/physrevb.101.020503
28. Ryee S, Yoon H, Kim TJ, Jeong MY, Han MJ. Induced Magnetic Two-Dimensionality By Hole Doping In The Superconducting Infinite-Layer Nickelate Nd1-xSrxNiO2. Phys Rev B (2020) 101:064513. doi:10.1103/physrevb.101.064513
29. Gu Y, Zhu S, Wang X, Hu J, Chen H. A Substantial Hybridization between Correlated Ni-D Orbital and Itinerant Electrons in Infinite-Layer Nickelates. Commun Phys (2020) 3:84. doi:10.1038/s42005-020-0347-x
30. Leonov I, Skornyakov SL, Savrasov SY. Lifshitz Transition and Frustration of Magnetic Moments in Infinite-Layer NdNiO2 upon Hole Doping. Phys Rev B (2020) 101:241108. doi:10.1103/physrevb.101.241108
31. Lechermann F. Late Transition Metal Oxides with Infinite-Layer Structure: Nickelates versus Cuprates. Phys Rev B (2020) 101:081110. doi:10.1103/physrevb.101.081110
32. Lechermann F. Multiorbital Processes Rule the Nd1−xSrxNiO2 normal State. Phys Rev X (2020) 10:041002. doi:10.1103/physrevx.10.041002
33. Hu Y, Liu G, Hao W, Liu Y, Liu Z. Experimental Study on Regression Model of Ultraviolet Laser Processing Thermal Barrier Coating Based on Response Surface Method. J Phys Conf Ser (2019) 1187:032046. doi:10.1088/1742-6596/1187/3/032046
34. Sakakibara H, Usui H, Suzuki K, Kotani T, Aoki H, Kuroki K. Model Construction and a Possibility of Cupratelike Pairing in a New d9 Nickelate Superconductor (Nd, Sr)NiO2. Phys Rev Lett (2020) 125:077003. doi:10.1103/physrevlett.125.077003
35. Jiang M, Berciu M, Sawatzky GA. Critical Nature of the Ni Spin State in Doped NdNiO2. Phys Rev Lett (2020) 124:207004. doi:10.1103/physrevlett.124.207004
36. Werner P, Hoshino S. Nickelate Superconductors: Multiorbital Nature and Spin Freezing. Phys Rev B (2020) 101:041104. doi:10.1103/physrevb.101.041104
37. Zhang H, Jin L, Wang S, Xi B, Shi X, Ye F, et al. Effective Hamiltonian for Nickelate Oxides Nd1-xSrxNiO2. Phys Rev Res (2020) 2:013214. doi:10.1103/physrevresearch.2.013214
38. Karp J, Botana AS, Norman MR, Park H, Zingl M, Millis A. Many-Body Electronic Structure of NdNiO2 and CaCuO2. Phys Rev X (2020) 10:021061. doi:10.1103/physrevx.10.021061
39. Botana AS, Norman MR. Similarities and Differences between LaNiO2 and CaCuO2 and Implications for Superconductivity. Phys Rev X (2020) 10:011024. doi:10.1103/physrevx.10.011024
40. Wang Y, Kang C-J, Miao H, Kotliar G. Hund's Metal Physics: From SrNiO2 to LaNiO2. Phys Rev B (2020) 102:161118. doi:10.1103/physrevb.102.161118
41. Olevano V, Bernardini F, Blase X, Cano A. Ab Initio many-body GW Correlations in the Electronic Structure of LaNiO2. Phys Rev B (2020) 101:161102(R). doi:10.1103/physrevb.101.161102
42. Zhang Y-H, Vishwanath A. Type-II t-J model in Superconducting Nickelate Nd1-xSrxNiO2. Phys Rev Res (2020) 2:023112. doi:10.1103/physrevresearch.2.023112
43. Petocchi F, Christiansson V, Nilsson F, Aryasetiawan F, Werner P. Normal State of Nd1-xSrxNiO2 from Self-Consistent GW+EDMFT. Phys Rev X (2020) 10:041047. doi:10.1103/physrevx.10.041047
44. Pan GA, Segedin DF, LaBollita H, Song Q, Nica EM, Goodge BH, et al. Superconductivity in a Quintuple-layer Square-planar Nickelate. Nat Mater (published online) (2021). doi:10.1038/s41563-021-01142-9
45. Zhang J, Tao X. Review on quasi-2D Square Planar Nickelates. CrystEngComm (2021) 23:3249–64. doi:10.1039/d0ce01880e
48. Botana AS, Bernardini F, Cano A. Nickelate Superconductors: An Ongoing Dialog between Theory and Experiments. J Exp Theor Phys (2021) 132:618–27. doi:10.1134/s1063776121040026
49. Osada M, Wang BY, Lee K, Li D, Hwang HY. Phase Diagram of Infinite Layer Praseodymium Nickelate Pr1−xSrxNiO2 Thin Films. Phys Rev Mater (2020) 4:121801. doi:10.1103/physrevmaterials.4.121801
50. Lu H, Rossi M, Nag A, Osada M, Li DF, Lee K, et al. Magnetic Excitations in Infinite-Layer Nickelates. Science (2021) 373:213–6. doi:10.1126/science.abd7726
51. Lee K-W, Pickett WE. Infinite-layer LaNiO2: Ni1+ is not Cu2+. Phys Rev B (2004) 70:165109. doi:10.1103/physrevb.70.165109
52. Anisimov VI, Bukhvalov D, Rice TM. Electronic Structure of Possible Nickelate Analogs to the Cuprates. Phys Rev B (1999) 59:7901–6. doi:10.1103/physrevb.59.7901
53. Krishna J, LaBollita H, Fumega AO, Pardo V, Botana AS. Effects of Sr Doping on the Electronic and Spin-State Properties of Infinite-Layer Nickelates: Nature of Holes. Phys Rev B (2020) 102:224506. doi:10.1103/physrevb.102.224506
54. Weber C, Yee C, Haule K, Kotliar G. Scaling of the Transition Temperature of Hole-Doped Cuprate Superconductors with the Charge-Transfer Energy. Epl (2012) 100:37001. doi:10.1209/0295-5075/100/37001
55. Been E, Lee WS, Hwang HY, Cui Y, Zaanen J, Deveraux T, et al. Electronic Structure Trends Across the Rare-Earth Series in Superconducting Infinite-Layer Nickelates. Phys Rev X (2021) 11:011050. doi:10.1103/physrevx.11.011050
56. Higashi K, Winder M, Kunes J, Hariki A. Core-Level X-Ray Spectroscopy of Infinite-Layer Nickelate: LDA + DMFT Study. Phys Rev X (2021) 11:041009. doi:10.1103/physrevx.11.041009
57. Kang B, Melnick C, Semon P, Ryee S, Han MJ, Kotliar G, et al. Infinite-Layer Nickelates As Ni-eg Hund’s Metals arXiv:2007.14610 (2021).
58. Ryee S, Han MJ, Choi S. Hund Physics Landscape of Two-Orbital Systems. Phys Rev Lett (2021) 126:206401. doi:10.1103/physrevlett.126.206401
59. Liu Z, Xu C, Cao C, Zhu W, Wang ZF, Yang J. Doping Dependence of Electronic Structure of Infinite-layer NdNiO2. Phys Rev B (2021) 103:045103. doi:10.1103/physrevb.103.045103
60. Kang C-J, Kotliar G. Optical Properties of the Infinite-Layer La1−xSrxNiO2 and Hidden Hund's Physics. Phys Rev Lett (2021) 126:127401. doi:10.1103/physrevlett.126.127401
61. Plienbumrung T, Daghofer M, Oleś AM. Interplay between Zhang-Rice Singlets and High-Spin States in a Model for Doped NiO2 Planes. Phys Rev B (2021) 103:104513. doi:10.1103/physrevb.103.104513
62. Zhao D, Zhou YB, Fu Y, Wang L, Zhou XF, Cheng H, et al. Intrinsic Spin Susceptibility and Pseudogaplike Behavior in Infinite-Layer LaNiO2. Phys Rev Lett (2021) 126:197001. doi:10.1103/physrevlett.126.197001
63. Lin H, Gawryluk DJ, Klein YM, Huangfu S, Pomjakushina E, von Rohr F, et al. Universal Spin-Glass Behaviour In Bulk LaNiO2, PrNiO2, and NdNiO2. arXiv:2104.14324 (2021).
64. Zhang R, Lane C, Singh B, Nokelainen J, Barbiellini B, Markiewicz RS, et al. Magnetic and F-Electron Effects in LaNiO2 and NdNiO2 Nickelates with Cuprate-like 3dx2-y2 Band. Commun Phys (2021) 4:118. doi:10.1038/s42005-021-00621-4
65. Choi M-Y, Pickett WE, Lee K-W. Fluctuation-frustrated Flat Band Instabilities in NdNiO2. Phys Rev Res (2020) 2:033445. doi:10.1103/physrevresearch.2.033445
66. Wu X, Di Sante D, Schwemmer T, Hanke W, Hwang HY, Raghu S, et al. Robust dx2-y2 -wave Superconductivity of Infinite-layer Nickelates. Phys Rev B (2020) 101:060504. doi:10.1103/physrevb.101.060504
67. Gu Q, Li Y, Wan S, Li H, Guo W, Yang H, et al. Single Particle Tunneling Spectrum of Superconducting Nd1-xSrxNiO2 Thin Films. Nat Commun (2020) 11:6027. doi:10.1038/s41467-020-19908-1
68. He R, Jiang P, Lu Y, Song Y, Chen M, Jin M, et al. Polarity-Induced Electronic and Atomic Reconstruction at NdNiO2/SrTiO3 Interfaces. Phys Rev B (2020) 102:035118. doi:10.1103/physrevb.102.035118
69. Bernardini F, Cano A. Stability and Electronic Properties of LaNiO2/SrTiO3 Heterostructures. J Phys Mater (2020) 3:03LT01. doi:10.1088/2515-7639/ab9d0f
70. Geisler B, Pentcheva R. Correlated Interface Electron gas in Infinite-Layer Nickelate Versus Cuprate Films on SrTiO3(001). Phys Rev Res (2021) 3:013261. doi:10.1103/physrevresearch.3.013261
71. Ortiz RA, Menke H, Misják F, Mantadakis DT, Fürsich K, Schierle E, et al. Superlattice Approach to Doping Infinite-Layer Nickelates. Phys Rev B (2021) 104:165137. doi:10.1103/physrevb.104.165137
72. Ren X, Gao Q, Zhao Y, Hailan Luo XZ, Zhu Z. Superconductivity in Infinite-Layer Pr{0.8}Sr{0.2}NiO2 Films on Different Substrates. arXiv:2109.05761 (2021).
73. LaBollita H, Botana AS. Tuning the Van Hove Singularities in AV3Sb5 (A=K, Rb, Cs) via Pressure and Doping. Phys Rev B (2021) 104:035148. doi:10.1103/physrevb.104.205129
74. Poltavets VV, Lokshin KA, Croft M, Mandal TK, Egami T, Greenblatt M. Crystal Structures of Ln4Ni3O8 (Ln = La, Nd) Triple Layer T'-type Nickelates. Inorg Chem (2007) 46:10887–91. doi:10.1021/ic701480v
75. Zhang J, Botana AS, Freeland JW, Phelan D, Zheng H, Pardo V, et al. Large Orbital Polarization in a Metallic Square-Planar Nickelate. Nat Phys (2017) 13:864–9. doi:10.1038/nphys4149
76. Zhang J, Chen Y-S, Phelan D, Zheng H, Norman MR, Mitchell JF. Stacked Charge Stripes in the quasi-2D Trilayer Nickelate La4Ni3O8. Proc Natl Acad Sci USA (2016) 113:8945–50. doi:10.1073/pnas.1606637113
77. Pardo V, Pickett WE. Pressure-Induced Metal-Insulator and Spin-State Transition in Low-Valence Layered Nickelates. Phys Rev B (2012) 85:045111. doi:10.1103/physrevb.85.045111
78. Karp J, Hampel A, Zingl M, Botana AS, Park H, Norman MR, et al. Comparative many-body Study of Pr4Ni3O8 and NdNiO2. Phys Rev B (2020) 102:245130. doi:10.1103/physrevb.102.245130
79. Zhang J, Pajerowski DM, Botana AS, Zheng H, Harriger L, Rodriguez-Rivera J, et al. Spin Stripe Order in a Square Planar Trilayer Nickelate. Phys Rev Lett (2019) 122:247201. doi:10.1103/physrevlett.122.247201
80. Botana AS, Pardo V, Pickett WE, Norman MR. Charge Ordering in Ni1+/Ni2+ Nickelates: La4Ni3O8 and La3Ni2O6. Phys Rev B (2016) 94:081105. doi:10.1103/physrevb.94.081105
81. Lin JQ, Villar Arribi P, Fabbris G, Botana AS, Meyers D, Miao H, et al. Strong Superexchange in a d9-δ Nickelate Revealed by Resonant Inelastic X-Ray Scattering. Phys Rev Lett (2021) 126:087001. doi:10.1103/physrevlett.126.087001
82. Cheng J-G, Zhou J-S, Goodenough JB, Zhou HD, Matsubayashi K, Uwatoko Y, et al. Pressure Effect on the Structural Transition and Suppression of the High-Spin State in the Triple-LayerT′−La4Ni3O8. Phys Rev Lett (2012) 108:236403. doi:10.1103/physrevlett.108.236403
83. Retoux R, Rodriguez-Carvajal J, Lacorre P. Neutron Diffraction and TEM Studies of the Crystal Structure and Defects of Nd4Ni3O8. J Solid State Chem (1998) 140:307–15. doi:10.1006/jssc.1998.7892
84. Pardo V, Pickett WE. Quantum Confinement Induced Molecular Correlated Insulating State in La4Ni3O8. Phys Rev Lett (2010) 105:266402. doi:10.1103/physrevlett.105.266402
85. Shen Y, Sears J, Fabbris G, Li J, Pelliciari J, Jarrige I, et al. Role of Oxygen States in Square Planar d9-Delta Nickelates. arXiv:2110.08937 (2021).
86. Botana AS, Pardo V, Norman MR. Electron Doped Layered Nickelates: Spanning the Phase Diagram of the Cuprates. Phys Rev Mater (2017) 1:021801. doi:10.1103/physrevmaterials.1.021801
87. Nica EM, Krishna J, Yu R, Si Q, Botana AS, Erten O. Theoretical Investigation of Superconductivity in Trilayer Square-Planar Nickelates. Phys Rev B (2020) 102:020504. doi:10.1103/physrevb.102.020504
88. Crocker J, Dioguardi A, Shirer K, Poltavets V, Greenblatt M, Klavins P, et al. NMR Evidence for Spin Fluctuations in the Bilayer Nickelate La3Ni2O6. Phys Rev B (2013) 88:075124.
89. Matsumoto Y, Yamamoto T, Nakano K, Takatsu H, Murakami T, Hongo K, et al. High‐Pressure Synthesis of A2 NiO2 Ag2 Se2 (A=Sr, Ba) with a High‐Spin Ni2+ in Square‐Planar Coordination. Angew Chem Int Ed (2019) 58:756–9. doi:10.1002/anie.201810161
90. Jin H-S, Pickett WE, Lee K-W. Proposed Ordering of Textured Spin Singlets in a Bulk Infinite-Layer Nickelate. Phys Rev Res (2020) 2:033197. doi:10.1103/physrevresearch.2.033197
91. Tu W-L, Moon E-G, Lee K-W, Pickett WE, Lee H-Y Field-Induced Bose-Einstein Condensation and Supersolid in the Two-Dimensional Kondo Necklace. arXiv:2107.11936 (2021).
92. Singh RRP Magnetism of Competing High-Spin/Low-Spin States in Ba2NiO2(AgSe)2 and Related Two-Orbital Two-Electron Systems. arXiv:2108.09706 (2021).
Keywords: superconductivity, electronic structure ab-initio calculations, nickelates, magnetism, cuprates electronic structure
Citation: Botana AS, Lee K-W, Norman MR, Pardo V and Pickett WE (2022) Low Valence Nickelates: Launching the Nickel Age of Superconductivity. Front. Phys. 9:813532. doi: 10.3389/fphy.2021.813532
Received: 11 November 2021; Accepted: 14 December 2021;
Published: 02 February 2022.
Edited by:
Matthias Eschrig, University of Greifswald, GermanyReviewed by:
Andrzej M. Oles, Jagiellonian University, PolandCopyright © 2022 Botana, Lee, Norman, Pardo and Pickett. This is an open-access article distributed under the terms of the Creative Commons Attribution License (CC BY). The use, distribution or reproduction in other forums is permitted, provided the original author(s) and the copyright owner(s) are credited and that the original publication in this journal is cited, in accordance with accepted academic practice. No use, distribution or reproduction is permitted which does not comply with these terms.
*Correspondence: Antia S. Botana, YW50aWEuYm90YW5hQGFzdS5lZHU=