A review of underwater acoustic metamaterials for underwater acoustic equipment
- 1School of Mechanical Engineering, Hangzhou Dianzi University, Hangzhou, China
- 2Marine Technology and Equipment Research Center, Hangzhou Dianzi University, Hangzhou, China
- 3Sanmen Sanyou Technology Inc, Taizhou, China
- 4School of Electronic Information, Hangzhou Dianzi University, Hangzhou, China
Researchers use underwater acoustic equipment to explore the unknown ocean environment, which is one of the important means to understand and utilize the ocean. For underwater acoustic equipment, the application of underwater acoustic metamaterials is the premise to ensure and improve the performance of underwater acoustic communication, acoustic stealth, and sonar detection. Due to the limitations of mass density law and high hydrostatic pressure, traditional underwater acoustic materials cannot effectively absorb low-frequency sound waves and have low efficiency of elastic energy conversion. The sound absorption effect is poor under low frequency and high hydrostatic pressure. In recent years, with the development of acoustic metamaterials technology, all kinds of underwater acoustic metamaterials have also been proposed. Compared with sound waves propagating in the air, underwater sound is more difficult to control than air sound with the same frequency, so the design of underwater acoustic metamaterials is more complicated. This paper reviews the basic characteristics, development history of sound absorption, sound insulation decoupling, and underwater acoustic guided metamaterials, then the existing problems and the future development direction of underwater acoustic metamaterials are discussed.
1 Introduction
For human beings, the ocean is a natural treasure house, which contains abundant natural resources. The marine ecosystem is closely related to human daily life [1, 2]. At present, human knowledge of the ocean is far less than that of the land. With the rapid development of underwater acoustic equipment (UAE) and underwater detection technology, higher requirements are put forward for acoustic performance of underwater target detection equipment, sea area information collection equipment and underwater military equipment (UME) [3]. Therefore, the application of underwater acoustic metamaterials is the key to improve the performance index of UAE.
The performance indexes of UAE mainly include underwater acoustic detection and acoustic stealth. As UAE is often used in various sea conditions, it will inevitably produce some subtle irregular vibrations, which will lead to near field self noise [4], resulting in the degradation of underwater acoustic detection performance of the equipment. In addition, more and more UME began to pursue “stealth” characteristics to reduce the probability of being discovered by enemy sonar. To solve these problems, in recent years, scholars have been studying underwater acoustic materials [5–8], aiming at reducing self-noise interference, improving their underwater acoustic communication and sonar detection performance, and countering active and passive sonar detection.
There are many classification methods of underwater acoustic materials, which can be divided into coating type and structural underwater acoustic materials according to the different molding process and bearing capacity. According to the frequency characteristics of the incident wave or its special structure, it can be divided into low, medium and high frequency underwater acoustic materials and porous and resonant underwater acoustic materials. According to the specific functions to be realized, underwater acoustic materials can be divided into sound absorption type, sound insulation type, decoupling type and reflect sound type.
Underwater acoustic materials include a wide range, which can be natural materials in nature, or metamaterials designed artificially according to task requirements or working frequency band. Among them, traditional underwater acoustic materials mainly include polymer materials, porous materials, filler materials, cavity resonant materials and impedance gradient materials [9, 10].
In 1990s, Sigalas and Economou proposed the concept of an artificial material/structure with elastic band gap mass density and periodic distribution of elastic constant, that is, phononic crystal. In the early stage when the concept of phononic crystal was put forward, scholars focused on the generation and modulation mechanism of band gap. The formation of phononic crystal band gap is mainly based on Bragg scattering mechanism. To control low-frequency waves, a large cell size is needed, which limits the engineering application of phononic crystals to a certain extent. In 2000, Liu et al. of Hong Kong University of Science and Technology found that the material had a low frequency band gap far lower than the Bragg band gap frequency by periodically arranging the local resonance unit composed of rubber coated shot in the elastic medium, and attributed the reason of this band gap to the resonance effect, so the concept of local resonance phononic crystal was put forward. At the same time, in the field of electromagnetism, researchers have designed “double negative materials” whose dielectric constant and permeability are negative at the same time, and called this kind of materials metamaterials. In 2004, Li and Chan of Hong Kong University of Science and Technology discovered that their designed composite artificial structure also showed “double negative” acoustic characteristics, so they proposed the concept of acoustic metamaterial by analogy with electromagnetic metamaterial. The development of acoustic metamaterials is shown in Figure 1.
Underwater acoustic metamaterial is also an artificial periodic structure [11] with microstructure as its basic building unit. This kind of structure can suppress waves in certain frequency ranges, which is called the so-called propagation “band gap” phenomenon. The frequency range of band gap can be adjusted by changing the configuration and spatial distribution of metamaterial microstructure units. This feature can be used to improve the signal to noise ratio of sonar system and reduce the near field self noise [12].
On the basis of acoustic metamaterials, underwater acoustic metamaterials transfer the research object from air to underwater, and the sound wave changes from air sound to underwater sound. Underwater acoustic metamaterials for underwater acoustic equipment are mainly divided into three categories according to different functions: (1) Sound absorption metamaterials (SAM): The absorption of sound waves is achieved by small surface reflection and high loss factor. It is mainly used to absorb near field self noise generated by the equipment body and active sonar detection sound waves. (2) Sound insulation decoupling metamaterials (SIDM): Through the impedance mismatch between the structure and the water, the self-noise caused by the vibration of the internal structure is blocked from transmitting into the water. (3) Underwater acoustic guided metamaterials (UAGM): Stealth is achieved by directing sound waves to travel along a specific path and thus changing their reflection path. The functional schematic diagram of three different types of underwater acoustic metamaterials is shown in Figure 2. In this paper, the basic characteristics, development history and future development direction of these three kinds of metamaterials are summarized, in order to point out the direction for the follow up researchers.

FIGURE 2. Schematic diagram of regulation function of three kinds of underwater acoustic metamaterials.
This paper is organized as follows. Introduction introduces the SAM. Introduction introduces the SIDM. Introduction introduces the UAGM. Introduction summarizes and discusses the future development direction of underwater sound absorption metamaterials.
2 Sound absorption metamaterials
Rubber polymer is usually used as the traditional acoustic absorbing material (TSAM), which is designed according to the two basic principles of impedance matching and damping dissipation [13, 14]. However, for the traditional sound absorbing materials, the reflection coefficient of the material surface is proportional to its loss factor, that is, there is a contradiction between the impedance matching requirement of the material and the high loss. Therefore, in order to promote acoustic damping dissipation, the internal acoustic structure of traditional materials needs to be changed. At present, the commonly used methods to change the acoustic structure of materials are the pore cavity resonance method, particle filling method, sandwich composite method, etc. At the same time, the TSAM also faces the two major problems of poor low frequency sound absorption capacity and poor sound absorption capacity under hydrostatic pressure.
Therefore, the development and design of low frequency (below 2 kHz), broadband, water pressure resistant SAM are the main challenges currently faced.
According to different sound absorption mechanisms, SAM can be divided into local resonance metamaterials (LRM) and non-resonance metamaterials (NRM).
In 2006, Zhao [15, 16] et al. introduced the local resonance theory into the design of sound-absorbing metamaterials for the first time, and systematically studied the absorption characteristics caused by local resonance. By filling the metal core covered by soft rubber layer in viscoelastic polymer as the local resonator, the underwater acoustic absorption effect at the local resonance frequency was verified. The cross section of metal steel ball array coated in viscoelastic polymer matrix is shown in Figure 3.
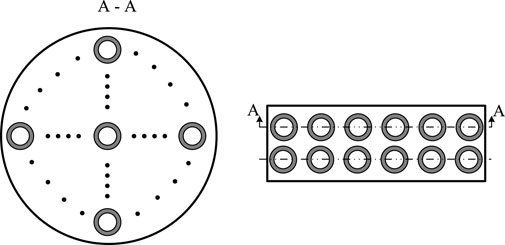
FIGURE 3. Cross sections of the array of coated steel spheres embedded in a viscoelastic polymer matrix.
The experimental results show that the designed local resonance metamaterial has low-frequency underwater sound absorption characteristics, and the underwater sound absorption effect at the local resonance frequency is obvious. Although the LRM has good sound absorption effect in low frequency band, the effective frequency range of sound absorption performance is relatively narrow. Therefore, Zhao, Wen et al. [17–20] further investigated the problem that the band gap width of LRM is too narrow by using finite element method and multiple scattering method. The results show that metamaterials with different local resonance frequencies can be superimposed to improve the sound absorption effect of metamaterials at low frequencies. Shi et al. [21] similarly designed a multilayer composite local resonance metamaterial, which widened or generated multiple band gaps by embedding multilayer local resonance couplings inside the matrix.
As shown in Figure 4, in order to explore the influence of coupling of multiple resonance units on sound absorption, Gu [22] et al. designed a sound-absorbing metamaterial with 12 resonance units in each basic unit. Under the action of this specific structure and multiple scattering, this material can absorb broadband low-frequency underwater sound in the frequency range of 600–2000 Hz and the high hydrostatic pressure of 0.5Mpa.
Zhong et al. [23] develops an underwater acoustic metamaterial plate with potential advantages of low-frequency broadband sound absorption and high hydrostatic pressure resistance. The proposed metamaterial is composed of particle-filled polyurethane damping materials and a square lattice of spiral resonators. The formation mechanisms of the locally resonant band gaps are investigated based on the modal analysis of plate modes and local resonances. Results show that In the frequency domain (0.8 kHz, 6 kHz), the average sound absorption coefficient of the proposed metamaterial plate under normal atmospheric pressure is 0.54. Furthermore, the sound absorption coefficient of the proposed structure is experimentally studied under different hydrostatic pressure conditions. Specifically, the proposed metamaterial structure achieves average sound absorption coefficient of 0.51 in the frequency range (1.5 kHz, 6 kHz) under 0.5 MPa.
At present, due to the limitation of the inherent characteristics of the local resonance theory, the sound absorption frequency band is narrow, and the sound absorption capacity needs to be improved in underwater use scenes. How to enhance the sound absorption band of metamaterials by using the multiple scattering effect generated by resonant coupling elements is a key issue in this field in the future.
NRM mainly includes porous foam metamaterials, gradient index metamaterials and other new sound absorption metamaterials.
A recent publication on broadband underwater metamaterial, Qu et al [24]. Present a novel metamaterial absorber with structured impedance-matched composite, which can realized an underwater acoustic absorber exhibiting high absorption from 4 to 20 kHz. And the structured composite represents a new type of acoustic metamaterials that has high acoustic energy density and promises broad underwater applications.
Xu et al. [25, 26] designed an open-cell SiC foam to solve the impedance mismatch problem of the existing underwater porous foam at low frequency. The experiment shows that the underwater sound absorption performance of the material can be improved by filling the holes with silicone oil, and the sound absorption coefficient is close to one in 750–4000 Hz. When the holes are filled with water, the sound absorption performance of the material can also be enhanced. However, it is worth noting that this material needs to rely on the rigid backing behind it. Wang et al. [27] developed a kind of gradient exponential metamaterial with acoustic black hole effect based on multiple scattering theory. The simulation results show that the metamaterial has an omnidirectional sound absorption effect in underwater broadband, which provides a new idea for the design of underwater acoustic coating.
In addition, Yuan et al. [14] proposed a kind of NRM based on the composite of graphene microchip and rubber material. The experimental results show that this material can enhance the absorption effect of 2–30 Hz underwater sound. Gao and Zhang et al. [28] put forward a kind of NRM with a spiral metal ring embedded in the viscoelastic layer. Results show that this metastructure can achieve the low frequency and broadband acoustic absorption below 1,000 Hz.
3 Sound insulation decoupling metamaterials
SIMD are mainly used to reduce the near-field self-noise caused by flow-induced vibration, so as to improve the sound detection and stealth performance of equipment.
At present, SIDM is mainly faced with two problems. First, if the soft rubber material is applied directly to the surface of the underwater acoustic equipment, the impedance mismatch can be formed. In this case, the structure of the device will become heavy and not conducive to use. Second, most of SIDMs are structural forms with embedded cavities, and the cavity volume is directly proportional to the impedance mismatch effect [3] and inversely proportional to the material pressure resistance [29]. The relationship between the two is difficult to balance. Therefore, how to improve the sound absorption performance while ensuring the pressure resistance of materials is the research focus of SIDM at present.
Previously, some researchers [30, 31] have studied the decoupling mechanism of traditional porous metamaterials and pointed out that its sound absorption mainly comes from the vibration isolation of the decoupling layer. Porous metamaterials has light weight and effective medium and high frequency decoupling ability, but its low frequency vibration suppression and isolation ability is still very weak, so it is difficult to play a decoupling role. Huang et al. [32] designed a complex axisymmetric pass based on the traditional cylindrical and conical pass and established an equivalent fluid model for simulation analysis. The simulation results show that the complex axisymmetric pass has better decoupling performance than the traditional pass.
Due to the design characteristics of a single local resonance unit, the sound insulation metamaterial also has the problem of the narrow sound insulation frequency band. However, the researchers found that the local resonators are also suitable for SIDM. In 2016, Huang et al. [33] introduced single-degree-of-freedom periodic local resonators into SIDM for the first time and established a computational model for local resonant scatterers based on effective medium theory and elastic dynamics theory. It is demonstrated that the effective density of the acoustic metamaterial decoupling layer has a great influence on the mechanical impedance of the system, and the low frequency noise reduction near the local resonance frequency is more obvious. Yang et al. [8] designed a kind of SIDM for UAE, which broadened the sound absorption band of metamaterials by coupling the local resonance and Bragg sound insulation band.
Zhong et al. [34] proposes a new type of composite underwater honeycomb-type acoustic metamaterial (AM) plate with the advantages of low-frequency broadband sound insulation and high hydrostatic pressure resistance. The underwater sound insulation performance and mechanisms of the new type of AM were investigated numerically and experimentally. The results show that the honeycomb-type AM has good underwater sound insulation performance at low frequencies and the proposed AM structure can still achieve an average sound transmission loss of 10 dB in the frequency range (2kHz, 10 kHz) under the hydrostatic pressure of 3 Mpa.
The chiral structure is a kind of porous structure with high shear stiffness, negative Poisson’s ratio and negative equivalent mass density. Wang et al. [35] studied the suppression effect of different frequency regions on acoustic radiation noise by adding a metal core local harmonic oscillator to chiral structural materials. The results show that not only chiral materials can also be used in SIDM, but also the coupling relationship between the local harmonic oscillator and chiral structure can inhibit the vibration of materials. Spadoni and Ruzzene et al. [36, 37] have analyzed the sound radiation characteristics of chiral beams, and the results show that chiral structures have an important influence on the vibration and sound isolation of beams. Zhu et al. [38] designed three groups of control tests (solid coating, chiral metamaterial coating and chiral coating filled with polystyrene foam) to verify the influence of different materials on the sound insulation effect of chiral metamaterials. The experimental results show that polystyrene foam is helpful to enhance the sound insulation effect of chiral metamaterials.
In addition, mechanical metamaterials with negative Poisson’s ratio are also widely used in the field of sound insulation and decoupling. The negative Poisson’s ratio property means that when a material is axially compressed, it will contract longitudinally, and when it is axially stretched, it will expand longitudinally. When the transverse strain of the material is equal to the longitudinal strain, the Poisson’s ratio is -1. Its basic unit body, tension and compression schematic are shown in Figure 5. Zhang et al. [39] designed a honeycomb vibration isolator for ships by using the characteristic of negative Poisson’s ratio. The metamaterial has a good vibration isolation effect below 50 Hz.
4 Underwater acoustic guided metamaterials
In recent years, with the increasingly wide application of UAE, not only the requirements for detection and communication accuracy are getting higher and higher, but also how to realize “stealth” detection has gradually become a research hotspot. Underwater acoustic control based on the principle of sound absorption and sound insulation will inevitably leave a “sound shadow area”, which is difficult to resist multi-directional stereo detection technologies such as multi-base sonar detection. Through the structural design of metamaterials, guiding sound waves to propagate along a specific path is the basic idea to realize stealth function.
In 2006, based on the theory of electromagnetic wave regulation, Pendry et al. [40] predicted the wave’s propagation trajectory to deduce the required material structure and designed an invisibility cloak that could control the wave to bypass the target without scattering, and provides a general theory for the design of uniform materials to guide sound waves to propagate along a specific curved path. As shown in Figure 6, the acoustic guidance control model and stealth effect diagram of stealth cloak can control acoustic waves to bypass the target object without scattering. Based on this idea, Cummer and Schuring et al. [41] developed the theory of transformation acoustics. In 2007, Chen et al. [42] demonstrated the possibility of transforming acoustic theory and designed a theoretical model of an invisibility cloak, which achieved the ideal invisibility effect. In 2018, Bi et al. [43] designed a three-dimensional underwater acoustic stealth cloak based on the theory of transformation acoustics. Its structural shape is an octahedral pyramid. When used, it is placed above the target, and the scattered wave is manipulated to imitate the plane scattered wave, so as to achieve the effect of hiding the target. However, these metamaterials are made up of anisotropic and heterogeneous media, and there are still great challenges in physical realization and engineering application.
In 2008, Norris et al. [12] Based on the transformation acoustic theory of anisotropic elastic modulus, the design idea of acoustic invisibility cloak using Pentamode metamaterials is proposed. Pentamode metamaterials have the characteristics of shear modulus far less than compression modulus, all-solid medium, and wide frequency band [44], so it has more application value in engineering. In 2013, On the basis of previous studies, Norris et al. [45] conducted experiments on the underwater acoustic refraction principle of pentamode metamaterials, and verified the feasibility of pentamode metamaterials in the field of underwater acoustic control. The variable model, solid characteristics, and anisotropic heterogeneous media make the pentamode metamaterials supplement the deficiency of UAGM, so it shows Pentamode metamaterials have gradually become the main way to realize underwater acoustic guided metamaterials.
Sun et al. [46] obtained the parameters needed for cloaks in impedance matching by inverse Laplace equation and designed cloaks based on pentamode metamaterials. The simulation results show that the cloak has a good broadband stealth effect. Chen et al. [47] designed a practical pentamode metamaterials cloak. The proposed cloak is assembled by pentamode lattice made of a single-phase solid material. During the simulation process, it was found that the pentamode metamaterials cloak could not achieve the ideal broadband characteristics because of its low shear modulus. Therefore, the pentamode metamaterials cloak could only hide the target within the segmented frequency range. Chen et al. found that the shear resonance can be improved by introducing material damping, and then the broadband performance can be improved.
Zhao et al. [48] prepared a two-dimensional pentamode metamaterials sample using a single metal material and verified its acoustic characteristics. The experimental results prove the correctness of the design idea. The above work shows that the pentamode metamaterials have gradually become the main way to realize UAGM.
Due to the limitation of material characteristics, at the beginning of the design of UAGM, its operating frequency band is set to a fixed value, which can only play the effect of diffraction regulation on the incident wave of a specific frequency. In the future, how to flexibly adjust the working frequency band of UAGM with the change of incident wave frequency and realize adaptive control is the main challenge before it is applied to underwater engineering.
5 Summary and prospect
This paper reviews the sound absorption mechanism and structural design process of SAM, SIDM, and UAGM from three perspectives: basic properties of metamaterials, development history, and future development directions. This paper also summarizes the sound absorption effect and underwater sound absorption performance of underwater acoustic metamaterials to provide a reference for subsequent researchers. The conclusions of this paper are as follows:
(1) Due to the limitation of the inherent characteristics of the local resonance theory, the sound absorption band of SAM is narrow and the sound absorption capacity for underwater use scenarios still needs to be improved. How to use the multiple scattering effects generated by the resonant coupling unit to enhance the sound absorption band of metamaterials is a key issue in this field in the future.
(2) In the environment of high hydrostatic pressure, SIDM is usually designed to be thicker and heavier. How to apply it in UAE under the premise of light weight is a major engineering problem for future sound insulation decoupling metamaterials.
(3) How to adjust the working frequency band of UAGM flexibly with the change of incident wave frequency and realize the active guidance of incident sound waves is a difficult problem to be solved in UAGM.
Generally speaking, compared with the traditional underwater acoustic materials, the performance of underwater acoustic metamaterials has great advantages, but the practical engineering application effect needs further demonstration. In the future, underwater acoustic metamaterials will develop in the direction of broadband, low frequency, and pressure resistance.
Author contributions
ZZ is the general manager of the article, responsible for the design and writing of this article. NH provided valuable suggestions for the design and writing of this article. JW provided opinions on the application of metamaterials in underwater equipment. WL is in charge of literature research. JZ provided valuable suggestions for the design and writing of this article. MW provided valuable suggestions for revision. FZ, HD, and YZ is responsible for coordinating the authors.
Funding
This research was supported by the Zhejiang Science and Technology Plan Project (No. 2022C01199), and Fundamental Research Funds for the Provincial Universities of Zhejiang (GK219909299001-021). The funders had no role in the design of the study; in the collection, analyses, or interpretation of data; in the writing of the manuscript, or in the decision to publish the results.
Conflict of interest
Author JW was employed by the Sanmen Sanyou Technology Inc.
The remaining authors declare that the research was conducted in the absence of any commercial or financial relationships that could be construed as a potential conflict of interest.
Publisher’s note
All claims expressed in this article are solely those of the authors and do not necessarily represent those of their affiliated organizations, or those of the publisher, the editors and the reviewers. Any product that may be evaluated in this article, or claim that may be made by its manufacturer, is not guaranteed or endorsed by the publisher.
References
1. Wang J, Sun J, Xie W, Chen H, Wang C, Yu Y, et al. Simulation and analysis of multiphase flow in a novel deepwater closed-cycle riserless drilling method with a subsea Pump+Gas combined lift. Front Phys (2022) 10:10. doi:10.3389/fphy.2022.946516
2. Hu Y, Xu D, Zhou Z, Zhao T, Shi Y, Tian Y, et al. Research on the influence of metamaterials on single photon lidar. Front Phys (2020) 8:8. doi:10.3389/fphy.2020.585881
3. Zhang Y, Chen K, Hao X, Cheng Y. A review of underwater acoustic metamaterials. Chin Sci Bull (2020) 65(15):1396–410. doi:10.1360/tb-2019-0690
4. Yin J, Cai L, Fang X, Xiao Y, Yang H, Zhang H, et al. Review on research progress of mechanical metamaterials and their applications on vibration and noise control. Adv Mechanics(in Chinese) (2022) 2022:1–64. doi:10.6052/1000-0992-12-345
5. Ko SH. Reduction of structure-borne noise using an air-voided elastomer. J Acoust Soc Am (1997) 101(6):3306–12. doi:10.1121/1.418292
6. Tao M, Tang W, Hua H. Noise reduction analysis of an underwater decoupling layer. J Vib Acoust (2010) 132:061006. doi:10.1115/1.4002126
7. Hao H. Gain influence for a reflection baffle. Appl Acoust(in Chinese) (2011) 20:187–92. doi:10.3969/.issn.1000-310X.2011.03.004
8. Yang H, Xiao Y, Zhao H, Zhong J, Wen J. On wave propagation and attenuation properties of underwater acoustic screens consisting of periodically perforated rubber layers with metal plates. J Sound Vibration (2019) 444:21–34. doi:10.1016/j.jsv.2018.12.031
9. Mei J, Ma G, Yang M, Yang Z, Wen W, Sheng P. Dark acoustic metamaterials as super absorbers for low-frequency sound. Nat Commun (2012) 3:756. Epub 2012/03/29. doi:10.1038/ncomms1758
10. Yang M, Ma G, Yang Z, Sheng P. Coupled membranes with doubly negative mass density and bulk modulus. Phys Rev Lett (2013) 110(13):134301. Epub 2013/04/16. doi:10.1103/PhysRevLett.110.134301
11. Kushwaha MS, Halevi P, Dobrzynski L, Djafari-Rouhani B. Acoustic band structure of periodic elastic composites. Phys Rev Lett (1993) 71(13):2022–5. Epub 1993/09/27. doi:10.1103/PhysRevLett.71.2022
12. Norris AN. Acoustic cloaking theory. Proc R Soc A (2008) 464(2097):2411–34. doi:10.1098/rspa.2008.0076
13. Mei J, Zhang X, Wu Y. Ultrathin metasurface with high absorptance for waterborne sound. J Appl Phys (2018) 123(9):091710. doi:10.1063/1.5009382
14. Yuan B, Jiang W, Jiang H, Chen M, Liu Y. Underwater acoustic properties of graphene nanoplatelet-modified rubber. J Reinforced Plastics Composites (2018) 37(9):609–16. doi:10.1177/0731684418754411
15. Zhao H, Wen J, Yu D, Wen X. Low-frequency acoustic absorption of localized resonances: Experiment and theory. J Appl Phys (2010) 107(2):023519. doi:10.1063/1.3284943
16. Zhao H, Liu Y, Wen J, Yu D, Wen X. Tri-component phononic crystals for underwater anechoic coatings. Phys Lett A (2007) 367(3):224–32. doi:10.1016/j.physleta.2007.02.048
17. Wen J, Zhao H, Lv L, Yuan B, Wang G, Wen X. Effects of locally resonant modes on underwater sound absorption in viscoelastic materials. J Acoust Soc Am (2011) 130(3):1201–8. Epub 2011/09/08. doi:10.1121/1.3621074
18. Zhong J, Wen J-H, Zhao H-G, Yin J-F, Yang H-B. Effects of core position of locally resonant scatterers on low-frequency acoustic absorption in viscoelastic panel. Chin Phys B (2015) 24(8):084301. doi:10.1088/1674-1056/24/8/084301
19. Zhao H, Wen J, Yang H, Lv L, Wen X. Backing effects on the underwater acoustic absorption of a viscoelastic slab with locally resonant scatterers. Appl Acoust (2014) 76:48–51. doi:10.1016/j.apacoust.2013.07.022
20. Meng H, Wen J, Zhao H, Wen X. Optimization of locally resonant acoustic metamaterials on underwater sound absorption characteristics. J Sound Vibration (2012) 331(20):4406–16. doi:10.1016/j.jsv.2012.05.027
21. Shi K, Jin G, Liu R, Ye T, Xue Y. Underwater sound absorption performance of acoustic metamaterials with multilayered locally resonant scatterers. Results Phys (2019) 12:132–42. doi:10.1016/j.rinp.2018.11.060
22. Gu Y, Zhong H, Bao B, Wang Q, Wu J. Experimental investigation of underwater locally multi-resonant metamaterials under high hydrostatic pressure for low frequency sound absorption. Appl Acoust (2021) 172:107605. doi:10.1016/j.apacoust.2020.107605
23. Zhong HB, Gu YH, Wu JH, Wang Q. 2D underwater acoustic metamaterials incorporating a combination of particle-filled polyurethane and spiral-based local resonance mechanisms. Compos Structures (2019) 220:1–10. doi:10.1016/j.compstruct.2019.03.091
24. Qu SC, Gao N, Tinel A, Morvan B, Romero-Garcia V, Groby JP, et al. Underwater metamaterial absorber withimpedance-matched composite. Sci Adv (2022) 8:eabm4206. doi:10.1126/sciadv.abm4206
25. Xu W, Jiang C, Zhang J. Underwater acoustic absorption of air-saturated open-celled silicon carbide foam. Colloids Surf A: Physicochemical Eng Aspects (2015) 471:153–8. doi:10.1016/j.colsurfa.2015.01.091
26. Xu W, Jiang C, Zhang J. Improvement in underwater acoustic absorption performance of open-celled sic foam. Colloids Surf A: Physicochemical Eng Aspects (2015) 482:568–74. doi:10.1016/j.colsurfa.2015.06.046
27. Wang C, Li S-D, Zheng W-G, Huang Q-B. Acoustic absorption characteristics of new underwater omnidirectional absorber. Chin Phys Lett (2019) 36(4):044301. doi:10.1088/0256-307x/36/4/044301
28. Gao N, Zhang Y. A low frequency underwater metastructure composed by helix metal and viscoelastic damping rubber. J Vibration Control (2018) 25(3):538–48. doi:10.1177/1077546318788446
29. Huang L, Xiao Y, Wen J, Yang H, Wen X. Analysis of decoupling mechanism of an acoustic coating layer with horizontal cylindrical cavities. Acta Phys Sin (2015) 64(15):154301. doi:10.7498/aps.64.154301
30. Ko S, Seong W, Pyo S. Structure-borne noise reduction for an infinite, elastic cylindrical shell. J Acoust Soc Am (2001) 109(4):1483–95. doi:10.1121/1.1349540
31. Laulagnet B, Guyader JL. Sound radiation from finite cylindrical coated shells, by means of asymptotic expansion of three-dimensional equations for coating. J Acoust Soc Am (1994) 96(1):277–86. doi:10.1121/1.410480
32. Huang L, Xiao Y, Wen J, Zhang H, Wen X. Optimization of decoupling performance of underwater acoustic coating with cavities via equivalent fluid model. J Sound Vibration (2018) 426:244–57. doi:10.1016/j.jsv.2018.04.024
33. Huang LZ, Xiao Y, Wen JH, Yang HB, Wen XS. Analysis of underwater decoupling properties of a locally resonant acoustic metamaterial coating. Chin Phys B (2016) 25(2):024302. doi:10.1088/1674-1056/25/2/024302
34. Wang D, Zhu D, Huang X. Influences of internal resonances on the vibration and sound reduction of chiral layer coating. Noise Vibr Contrl (in Chinese) (2015) 35:22–5. doi:10.3969/j.issn.1006-1335.2015.06.005
35. Zhong HB, Tian YJ, Gao NS, Lu K, Wu J. Ultra-thin composite underwater honeycomb-type acoustic metamaterial with broadband sound insulation and high hydrostatic pressure resistance. Compos Structures (2021) 277:114603. doi:10.1016/j.compstruct.2021.114603
36. Spadoni A, Ruzzene M. Structural and acoustic behavior of chiral truss-core beams. J Vib Acoust (2007) 5:616–26. doi:10.1115/1.2202161
37. Spadoni A, Ruzzene M, Gonella S, Scarpa F. Phononic properties of hexagonal chiral lattices. Wave Motion (2009) 46(7):435–50. doi:10.1016/j.wavemoti.2009.04.002
38. Zhu D, Huang X, wang Y, Xiao F, Hua H. Experimental and numerical research on the underwater sound radiation of floating structures with covering layers. Proc Inst Mech Eng C: J Mech Eng Sci (2014) 229(3):447–64. doi:10.1177/0954406214536719
39. Zhang G, Yang D, Zhu J. Performance analysis of a novel marine honeycomb vibration isolator. Chin J Ship Res (2013) 8:52–8. doi:10.3969/j.issn.1673-3185.2013.04.009
40. Pendry BJ, Schurig D, Smith DR. Controlling electromagnetic fields. science (2006) 312:5781–2. doi:10.1126/science.1125907
41. Cummer SA, Schurig D. One path to acoustic cloaking. New J Phys (2007) 9(3):45. doi:10.1088/1367-2630/9/3/045
42. Chen H, Chan CT. Acoustic cloaking in three dimensions using acoustic metamaterials. Appl Phys Lett (2007) 91(18):183518. doi:10.1063/1.2803315
43. Bi Y, Jia H, Sun Z, Yang Y, Zhao H, Yang J. Experimental demonstration of three-dimensional broadband underwater acoustic carpet cloak. Appl Phys Lett (2018) 112(22):223502. doi:10.1063/1.5026199
44. Su X, Norris AN, Cushing CW, Haberman MR, Wilson PS. Broadband focusing of underwater sound using a transparent pentamode lens. J Acoust Soc Am (2017) 141(6):4408–17. Epub 2017/06/18. doi:10.1121/1.4985195
45. Hladky-Hennion AC, Vasseur JO, Haw G, Croënne C, Haumesser L, Norris AN. Negative refraction of acoustic waves using a foam-like metallic structure. Appl Phys Lett (2013) 102(14):144103. doi:10.1063/1.4801642
46. Sun Z, Sun X, Jia H, Bi Y, Yang J. Quasi-isotropic underwater acoustic carpet cloak based on latticed pentamode metafluid. Appl Phys Lett (2019) 114(9):094101. doi:10.1063/1.5085568
47. Chen Y, Liu X, Hu G. Latticed pentamode acoustic cloak. Sci Rep (2015) 5:15745. Epub 2015/10/28. doi:10.1038/srep15745
Keywords: absorption materials, metamaterials, underwater acoustic equipment, sound absorption, sound isolation
Citation: Zhu Z, Hu N, Wu J, Li W, Zhao J, Wang M, Zeng F, Dai H and Zheng Y (2022) A review of underwater acoustic metamaterials for underwater acoustic equipment. Front. Phys. 10:1068833. doi: 10.3389/fphy.2022.1068833
Received: 13 October 2022; Accepted: 29 November 2022;
Published: 15 December 2022.
Edited by:
Han Zhang, Institute of Acoustics (CAS), ChinaReviewed by:
Chen Shen, Rowan University, United StatesCopyright © 2022 Zhu, Hu, Wu, Li, Zhao, Wang, Zeng, Dai and Zheng. This is an open-access article distributed under the terms of the Creative Commons Attribution License (CC BY). The use, distribution or reproduction in other forums is permitted, provided the original author(s) and the copyright owner(s) are credited and that the original publication in this journal is cited, in accordance with accepted academic practice. No use, distribution or reproduction is permitted which does not comply with these terms.
*Correspondence: Ning Hu, hooning@hdu.edu.cn; Jiabao Zhao, 212040208@hdu.edu.cn; Maofa Wang, wangmfl600@163.com; Fanzong Zeng, 202243010060@hdu.edu.cn; Huajie Dai, qinlongcrcc@foxmail.com; Yongju Zheng, joany0204@163.com