- 1School of Mechanical Engineering, Anhui Technical College of Mechanical and Electrical Engineering, Wuhu, China
- 2School of Mechanical and Electronic Engineering, Nanjing Forestry University, Nanjing, Jiangsu, China
- 3School of Mechanical Engineering, Southeast University, Nanjing, Jiangsu, China
- 4School of Automobile and Aviation, Wuhu Institute of Technology, Wuhu, China
- 5School of Automotive and Traffic Engineering, Hefei University of Technology, Hefei, China
- 6School of Automotive and Transportation Engineering, Shenzhen Polytechnic, Shenzhen, Guangdong, China
Recently, biphenylene was successfully synthesized as a novel allotrope of carbon. In this investigation, non-equilibrium molecular dynamics calculations are conducted to explore the intrinsic thermal properties of biphenylene. The isotropic thermal conductivity of biphenylene is obtained, which is also sensitive to size and temperature. Furthermore, the graphene/biphenylene lateral heterostructure is constructed to possess an interfacial thermal conductance of about 2.84 × 109 W K−1 m−2. The external tensile strain can induce a redshift of the vibrational density of states of pristine graphene and biphenylene, and the improved overlap also results in an enhanced heat flux in the biphenylene/graphene heterostructure. Our approach can provide theoretical guidance to design a thermal management device based on graphene and biphenylene.
Introduction
After the graphene was prepared by the Geim using a mechanical stripping method [1]; two-dimensional (2D) materials attract extensive attention because of their novel performance and promising applications [2–8]. Specifically, transition metal dichalcogenides (TMDs) and transition metal carbides or carbonitrides (MXene) have also been proposed to rise rapidly [9–12]. Their unique layered structure endows unusual physical and chemical properties, such as high mechanical strength [13] and excellent thermal [14], magnetic [4], and optical performance [15]. For example, when GeC changes from an indirect band gap to a direct band gap, it can maintain structural stability under high pressure [16]. Interestingly, the band gap of arsenene can be changed under the effect of stress. Research studies found a novel phenomenon, especially for folded arsenene: when 6% stress is applied, the gap of arsenene will be closed [17].
Thermal behaviors of 2D materials act as critical factors in heat dissipation when used as nano-devices, and the heat transport will directly determine the efficiency [18, 19]. Thus, research on the thermal properties of 2D materials has become a hotspot. For example, Balandin et al. measured the thermal conductivity of a suspended graphene monolayer in a room-temperature environment for the first time using the Raman method [20]. The measurement results show that its thermal conductivity is much higher than that of diamond and graphite block, reaching about 4,840–5,300 W m−1 K−1. Cai et al. obtained the thermal conductivity of the suspended graphene monolayer using the same method and simultaneously measured the Raman laser absorption of graphene using a laser power meter [21]. The results demonstrate that the thermal conductivity of the graphene monolayer grown by chemical vapor deposition (CVD) was about 2,500–3,100 W m−1 K−1 and 1,200–1,400 W m−1 K−1, at the temperatures 350 K and 500 K, respectively. Interestingly, when two different layered materials form a lateral heterostructure, the phonons can scatter when passing through the interface, resulting in interfacial thermal conductance [22]. For example, topological defects can improve the interfacial thermal conductance of graphene/h-BN from 6.42 × 109 W K−1 m−2 to 7.09 × 109 W K−1 m−2 [23]. The interfacial thermal conductance of the black phosphorene heterostructure can be reduced using a nanophononic structure [24]. In addition, the covalent interface of the lateral heterostructure can evidently induce a temperature drop, which is a critical factor for interfacial thermal conductance [25]. Recently, a novel non-benzenoid carbon allotrope was prepared [26], named biphenylene, which shows metallic characteristics [26]. It is an anti-aromatic compound and possesses tunable magnetic properties [27]. In addition, the maximum Young’s modulus of biphenylene is obtained as 259.7 N/m, and its ultra-high melting point is up to 4,500 K, showing strong mechanical and high stability performance [28]. In addition, biphenylene has been reported to be a candidate material for fuel cells [29].
In this work, the thermal conductivity of biphenylene is addressed using molecular dynamics calculations. Then, the size and temperature dependence of the thermal conductivity of biphenylene are addressed. Importantly, the biphenylene/graphene heterostructure is constructed, and interfacial thermal conductance is investigated. In addition, the stain-tunable interfacial thermal conductance of the biphenylene/graphene heterostructure is studied, and the vibrational density of states is further explored.
Computational methods
In our simulations, the LAMMPS package was used for all the molecular dynamics (MD) calculations [30]. The interaction between the C atoms was described by the Tersoff potential [3]. The time step was set as 0.5 fs and NPT (isothermal and isobaric), NVT, and NVE (isovolumetric and isoenergetic) ensembles were conducted for 500 ps in the MD simulations to obtain an equilibrium state for the system. Moreover, Newton’s equations were reflected in the integrated velocity. The Verlet algorithm was used to show the atomic motion. The temperature and energy of the studied system were monitored, and they also present a convergence, further showing a steady state. In the calculations for the phonon spectra of the system, the density functional perturbation theory (DFPT) within the PHONOPY code was used [31, 32].
Results and discussion
In order to investigate the heat transfer characteristics along the x direction, the nanoribbon of the biphenylene monolayer is constructed along x, as shown in Figure 1. Two ends of biphenylene are fixed and hot and cold baths are used by Nosé–Hoover reservoirs next to the two ends. Thus, the heat current induced by such a temperature gradient is obtained along the x direction. The size dependence of thermal conductivity was considered by setting the width (in the y direction) of the biphenylene nanoribbon to about 50 Å, while the length (L, in the x direction) is variable by 411.1, 616.5, 822.8, 1,027.8, and 1,233.4 Å. Similarly, the thermal conductivity of the biphenylene monolayer along the y direction is also calculated using the nanoribbon with the width (in the x direction) of the biphenylene nanoribbon about 50 Å, while the length (in the y direction) is variable by 409.1, 614.0, 818.3, 1,022.8, and 1,227.0 Å.
The thermal conductivity
where J is the heat flux and T and S are the temperature and the cross-sectional area of the heat flux, respectively [33]. Importantly, dT/dL is obtained by the fitting of the linear region. In addition, we calculate the heat flux (J) using the non-equilibrium molecular dynamics (NEMD) method [30]:
where εi is the energy of the atoms I, while vi represents the velocity of the atom. rij is used to decide the distance between i and j. Fij and Fijk are the two-body and three-body forces, respectively. V is the volume of the studied system. The thermal conductivity of the biphenylene monolayer is shown in Figure 2A with the length ranging from 40 nm to 120 nm at 300 K in the x and y directions. One can see that the biphenylene monolayer reveals isotropic thermal conductivity, which is enhanced as the length increases, but it will not increase at a certain length of about 100 nm. In addition, we obtain the maximum thermal conductivity of about 52.82 W m−1 K−1 and 50.97 W m−1 K−1 in the x and y directions, respectively. In contrast, the thermal conductivity of the biphenylene monolayer can be decreased by increasing the temperature, as shown in Figure 2B, in both x and y directions. In addition, there is a decent linear relationship between temperature and thermal conductivity. It is worth noting that the thermal conductivity of biphenylene in the x direction is higher than that in the y direction, which is contributed by the higher group velocity in the x direction because the acoustic branches in the x direction are steeper than those in the y direction, as shown in Figure 2C.
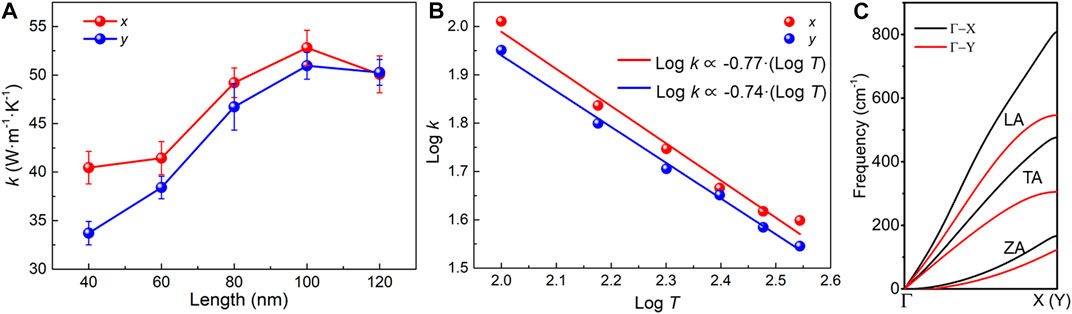
FIGURE 2. Thermal conductivity of the biphenylene monolayer by different (A) lengths and (B) temperatures; (C) phonon scattering spectrum of the biphenylene monolayer along the x and y directions.
Then, we construct the lateral heterostructure by biphenylene and graphene with the length and width as 613.7 and 47.5 Å, respectively, as shown in Figure 3A, where the interface is denoted by the blue dashed line. To investigate the interface properties of biphenylene and graphene, the potential drop (ΔV) is calculated as shown in Figure 3B. The obtained potential drop of the biphenylene/graphene interface is 0.326 eV, which is important to induce a built-in electric field enhancing the migration of the charges [10]. Then, the stability of the biphenylene/graphene lateral heterostructure is calculated by the phonon spectrum, as shown in Figure 3C. One can see that an imaginary frequency exists in the phonon spectrum of the biphenylene/graphene lateral heterostructure, suggesting the stability of the structure. To explore the thermal performance of the biphenylene/graphene lateral heterostructure, the NEMD method is also used to obtain the heat flux of the calculated system. Similarly, hot and cold baths are fixed on biphenylene and graphene, respectively, by Nosé–Hoover reservoirs as shown in Figure 3A. Thus, the heat flux is transferred from graphene to biphenylene. After crossing the interface, the interfacial thermal conductance of the biphenylene/graphene lateral heterostructure is further explored by
where ΔT is the temperature difference across the biphenylene/graphene interface. In addition, a free boundary condition was set in the y-direction and z-direction to build such a nanoribbon structure for the biphenylene/graphene lateral heterostructure. The system temperature is decided by the average temperature of the hot and cold baths.
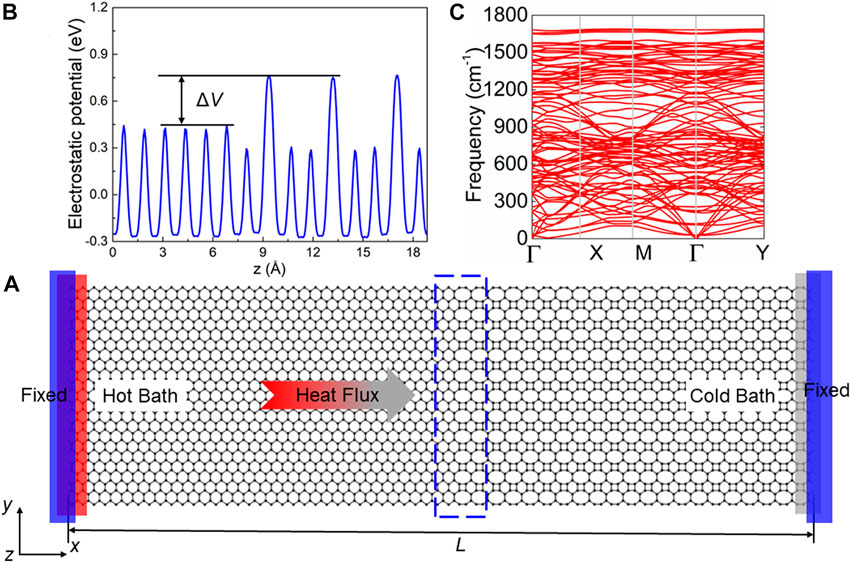
FIGURE 3. (A) Schematic of heat transfer in the biphenylene/graphene lateral heterostructure; (B) potential drop and (C) phonon scattering spectrum of the interface.
The temperature difference of the biphenylene/graphene lateral heterostructure under different temperatures is shown in Figure 4. Evidently, a large temperature difference is induced across the interface. The obtained temperature difference of the biphenylene/graphene lateral heterostructure at 300 K is about 11.02 K. In addition, the heat flux is calculated as 3.13 × 1010 W m−2; thus, the interfacial thermal conductance of the biphenylene/graphene lateral heterostructure is about 2.84 × 109 W K−1 m−2. Such a pronounced temperature drop resulted in phonon scattering across the interface. In addition, the calculated interfacial thermal conductance of the biphenylene/graphene heterostructure is higher than that of other graphene-based lateral heterostructures such as graphene/BN (about 1.2 × 109 W K−1 m−2) [34], graphene/metal (about 2.50 × 108 W K−1 m−2) [35], graphene/phosphorene (about 2.49 × 108 W K−1 m−2) [36], and graphene/MoS2 (about 2.49 × 108 W K−1 m−2) [37].
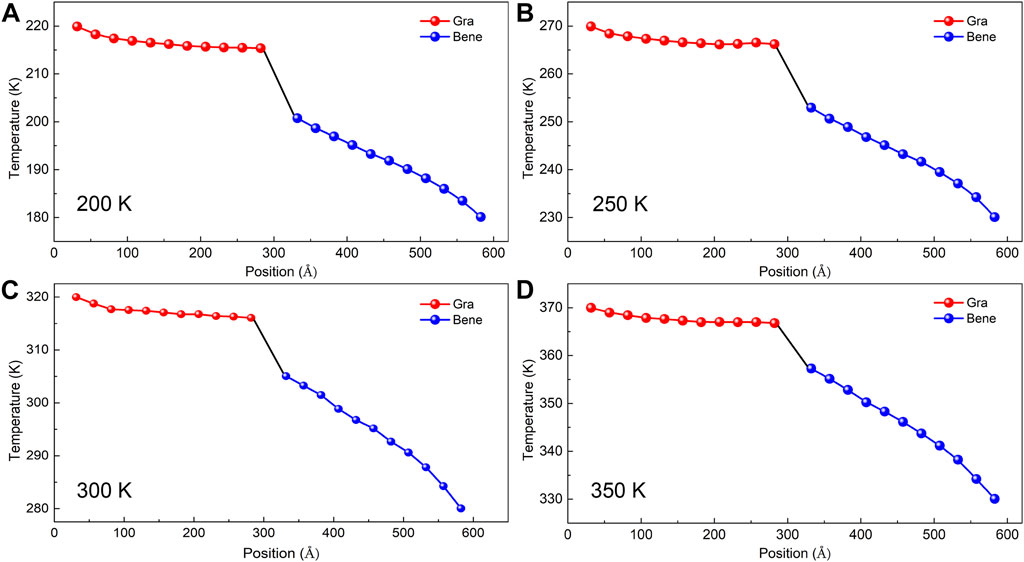
FIGURE 4. Temperature distribution of the biphenylene/graphene lateral heterostructure at (A) 200 K, (B) 250 K, (C) 300 K, and (D) 350 K.
Strain engineering is a popular method to tune the properties of the 2D materials, such as electronic [38], thermal [39], and thermoelectric [40] performances. In general, the thermal conductivity of 2D materials can be reduced because of the suppressed phonon group velocity and the improved anharmonicity under external tensile strain, which is reported by graphene [41, 42]. Thus, we further investigate the response of the thermal transport of the biphenylene/graphene lateral heterostructure on external tensile stress along the x direction. We can see that the heat flux of the biphenylene/graphene heterostructure is increased while the interfacial thermal conductance is decreased by the strain, as shown in Figure 5A and Figure 5B, respectively. To clarify the intrinsic mechanism of the strain-dependent thermal transport in the biphenylene/graphene heterostructure, the vibrational density of states (VDOS) of pristine graphene and biphenylene is calculated, which is defined as follows [43]:
where ω and C(t) demonstrate the angular frequency and the velocity auto-correlation function, respectively. In the calculations of the total VDOS, C(t) is obtained by
where
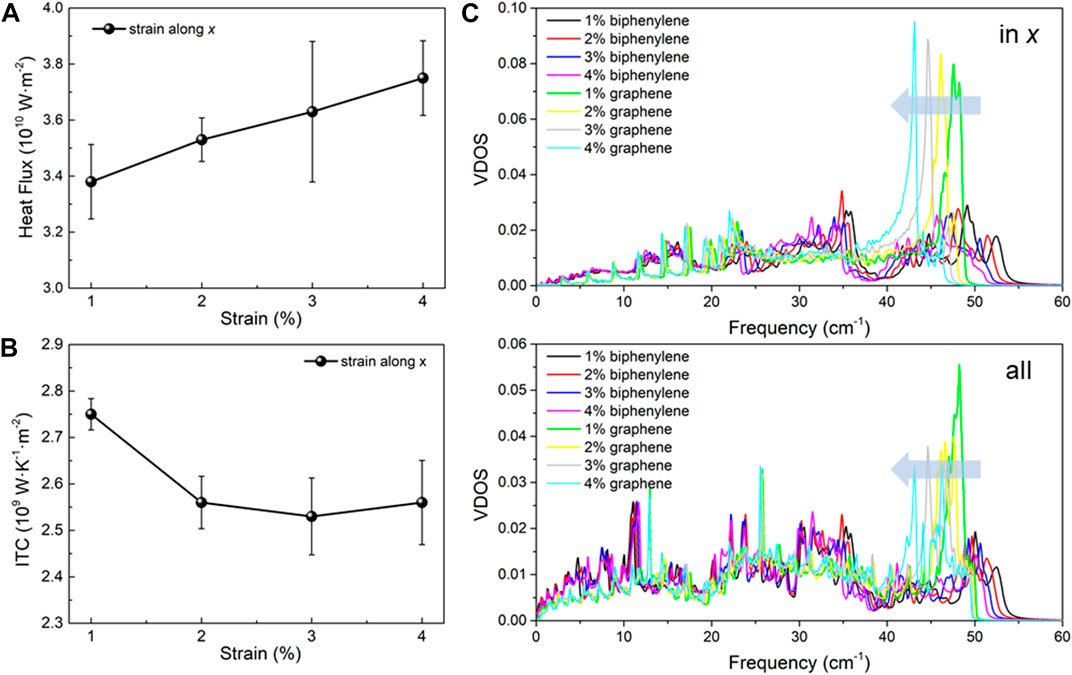
FIGURE 5. (A) Heat flux, (B) interfacial thermal conductance, and (C) VDOS of the biphenylene/graphene lateral heterostructure under external strain along the x direction.
Conclusion
In conclusion, the non-equilibrium molecular dynamics calculations in this work demonstrate that biphenylene possesses isotropic thermal transport characteristics. The thermal conductivity of biphenylene can be improved by length along the heat flux but reduced by increasing the temperature. The graphene/biphenylene heterostructure presents interfacial thermal conductance of about 2.84 × 109 W K−1 m−2. In addition, the interfacial thermal conductance of the biphenylene/graphene heterostructure is found to be dependent on strain, and the enhanced heat flux by the strain results from the larger overlap of the VDOS between graphene and biphenylene.
Data availability statement
The original contributions presented in the study are included in the article/Supplementary Material; further inquiries can be directed to the corresponding author.
Author contributions
Conceptualization, QC; methodology, KR and RZ; software, JL; validation, KR; investigation, JL; writing—original draft preparation, QC, KR and RZ; writing—review and editing, JL; funding acquisition, QC and KR. All authors have read and agreed to the published version of the manuscript.
Funding
This research is supported by the Key projects of natural science research in Colleges and Universities of Anhui Province (KJ2021A1520, KJ2018A0698, and KJ2020A0909) and the Natural Science Foundation of Jiangsu (No. BK20220407).
Conflict of interest
The authors declare that the research was conducted in the absence of any commercial or financial relationships that could be construed as a potential conflict of interest.
Publisher’s note
All claims expressed in this article are solely those of the authors and do not necessarily represent those of their affiliated organizations, or those of the publisher, the editors, and the reviewers. Any product that may be evaluated in this article, or claim that may be made by its manufacturer, is not guaranteed or endorsed by the publisher.
References
2. Zhang L, Ren K, Li J, Cui Z, Cheng H. The first-principles study of external strain tuning the electronic and optical properties of the 2D MoTe2/PtS2 van der Waals heterostructure. Front Chem (2022) 10:934048. doi:10.3389/fchem.2022.934048
3. Ren K, Chen Y, Qin H, Feng W, Zhang G. Graphene/biphenylene heterostructure: Interfacial thermal conduction and thermal rectification. Appl Phys Lett (2022) 121:082203. doi:10.1063/5.0100391
4. Zhang J, Zhang C, Ren K, Lin X, Cui Z. Tunable electronic and magnetic properties of Cr2Ge2Te6 monolayer by organic molecular adsorption. Nanotechnology (2022) 33:345705. doi:10.1088/1361-6528/ac715d
5. Wang G, Zhi Y, Bo M, Xiao S, Li Y, Zhao W, et al. 2D hexagonal boron nitride/cadmium sulfide heterostructure as a promising water-splitting photocatalyst. Phys Status Solidi B (2020) 257:1900431. doi:10.1002/pssb.201900431
6. Wang G, Zhang L, Li Y, Zhao W, Kuang A, Li Y, et al. Biaxial strain tunable photocatalytic properties of 2D ZnO/GeC heterostructure. J Phys D Appl Phys (2020) 53:015104. doi:10.1088/1361-6463/ab440e
7. Wang G, Gong L, Li Z, Wang B, Zhang W, Yuan B, et al. A two-dimensional CdO/CdS heterostructure used for visible light photocatalysis. Phys Chem Chem Phys (2020) 22:9587–92. doi:10.1039/d0cp00876a
8. Ren K, Wang K, Zhang G. Atomic adsorption-controlled magnetic properties of a two-dimensional (2D) janus monolayer. ACS Appl Electron Mater (2022) 4:4507–13. doi:10.1021/acsaelm.2c00740
9. Zhang H, Chhowalla M, Liu Z. 2D nanomaterials: Graphene and transition metal dichalcogenides. Chem Soc Rev (2018) 47:3015–7. doi:10.1039/c8cs90048e
10. Ren K, Sun M, Luo Y, Wang S, Yu J, Tang W. First-principle study of electronic and optical properties of two-dimensional materials-based heterostructures based on transition metal dichalcogenides and boron phosphide. Appl Surf Sci (2019) 476:70–5. doi:10.1016/j.apsusc.2019.01.005
11. Ren K, Zheng R, Xu P, Cheng D, Huo W, Yu J, et al. Electronic and optical properties of atomic-scale heterostructure based on MXene and mn (M = Al, Ga): A dft investigation. Nanomaterials (2021) 11:2236. doi:10.3390/nano11092236
12. Sun M, Re Fiorentin M, Schwingenschlögl U, Palummo M. Excitons and light-emission in semiconducting MoSi2X4 two-dimensional materials. Npj 2d Mater Appl (2022) 6:81. doi:10.1038/s41699-022-00355-z
13. Ren K, Ma X, Liu X, Xu Y, Huo W, Li W, et al. Prediction of 2D IV–VI semiconductors: Auxetic materials with direct bandgap and strong optical absorption. Nanoscale (2022) 14:8463–73. doi:10.1039/d2nr00818a
14. Ren K, Qin H, Liu H, Chen Y, Liu X, Zhang G. Manipulating interfacial thermal conduction of 2D janus heterostructure via a thermo-mechanical coupling. Adv Funct Mater (2022) 32:2110846. doi:10.1002/adfm.202110846
15. Ren K, Shu H, Huo W, Cui Z, Yu J, Xu Y. Mechanical, electronic and optical properties of a novel B2P6 monolayer: Ultrahigh carrier mobility and strong optical absorption. Phys Chem Chem Phys (2021) 23:24915–21. doi:10.1039/d1cp03838a
16. Pandey R, Rérat M, Darrigan C, Causà M. A theoretical study of stability, electronic, and optical properties of GeC and SnC. J Appl Phys (2000) 88:6462–6. doi:10.1063/1.1287225
17. Kamal C, Ezawa M. Arsenene: Two-dimensional buckled and puckered honeycomb arsenic systems. Phys Rev B (2015) 91:085423. doi:10.1103/physrevb.91.085423
18. Wang K, Ren K, Zhang D, Cheng Y, Zhang G. Phonon properties of biphenylene monolayer by first-principles calculations. Appl Phys Lett (2022) 121:042203. doi:10.1063/5.0102085
19. Qin H, Ren K, Zhang G, Dai Y, Zhang G. Lattice thermal conductivity of Janus MoSSe and WSSe monolayers. Phys Chem Chem Phys (2022) 24:20437–44. doi:10.1039/d2cp01692c
20. Balandin AA, Ghosh S, Bao W, Calizo I, Teweldebrhan D, Miao F, et al. Superior thermal conductivity of single-layer graphene. Nano Lett (2008) 8:902–7. doi:10.1021/nl0731872
21. Cai W, Moore AL, Zhu Y, Li X, Chen S, Shi L, et al. Thermal transport in suspended and supported monolayer graphene grown by chemical vapor deposition. Nano Lett (2010) 10:1645–51. doi:10.1021/nl9041966
22. Liu B, Baimova JA, Reddy CD, Law AW, Dmitriev SV, Wu H, et al. Interfacial thermal conductance of a silicene/graphene bilayer heterostructure and the effect of hydrogenation. ACS Appl Mater Inter (2014) 6:18180–8. doi:10.1021/am505173s
23. Liu X, Zhang G, Zhang YW. Topological defects at the graphene/h-BN interface abnormally enhance its thermal conductance. Nano Lett (2016) 16:4954–9. doi:10.1021/acs.nanolett.6b01565
24. Ren K, Liu X, Chen S, Cheng Y, Tang W, Zhang G. Remarkable reduction of interfacial thermal resistance in nanophononic heterostructures. Adv Funct Mater (2020) 30:2004003. doi:10.1002/adfm.202004003
25. Qin H, Pei QX, Liu Y, Zhang YW. The mechanical and thermal properties of MoS2-WSe2 lateral heterostructures. Phys Chem Chem Phys (2019) 21:15845–53. doi:10.1039/c9cp02499a
26. Fan Q, Yan L, Tripp MW, Krejčí O, Dimosthenous S, Kachel SR, et al. Biphenylene network: A nonbenzenoid carbon allotrope. Science (2021) 372:852–6. doi:10.1126/science.abg4509
27. Ren K, Shu H, Huo W, Cui Z, Xu Y. Tuning electronic, magnetic and catalytic behaviors of biphenylene network by atomic doping. Nanotechnology (2022) 33:345701. doi:10.1088/1361-6528/ac6f64
28. Luo Y, Ren C, Xu Y, Yu J, Wang S, Sun M. A first principles investigation on the structural, mechanical, electronic, and catalytic properties of biphenylene. Sci Rep (2021) 11:19008–6. doi:10.1038/s41598-021-98261-9
29. Liu T, Jing Y, Li Y. Two-dimensional biphenylene: A graphene allotrope with superior activity toward electrochemical oxygen reduction reaction. J Phys Chem Lett (2021) 12:12230–4. doi:10.1021/acs.jpclett.1c03851
30. Plimpton S. Fast parallel algorithms for short-range molecular dynamics. J Comput Phys (1995) 117:1–19. doi:10.1006/jcph.1995.1039
31. Togo A, Tanaka I. First principles phonon calculations in materials science. Scripta Materialia (2015) 108:1–5. doi:10.1016/j.scriptamat.2015.07.021
32. Togo A, Oba F, Tanaka IJPRB. First-principles calculations of the ferroelastic transition between rutile-type and CaCl2-type SiO2 at high pressures. Phys Rev B (2008) 78:134106. doi:10.1103/physrevb.78.134106
33. Qin H, Chen Y, Wu Y, Li M, Liu Y, Pei Q-X. Defect-engineered thermal transport in wrinkled graphene: A comprehensive molecular dynamics study. J Phys Chem C (2022) 126:5759–66. doi:10.1021/acs.jpcc.2c00324
34. Mao R, Kong B, Gong C, Xu S, Jayasekera T, Cho K, et al. First-principles calculation of thermal transport in metal/graphene systems. Phys Rev B (2013) 87:165410. doi:10.1103/physrevb.87.165410
35. Jiang J-W, Wang J-S. Manipulation of heat current by the interface between graphene and white graphene. Europhysics Lett (2011) 96:16003. doi:10.1209/0295-5075/96/16003
36. Liu X, Gao J, Zhang G, Zhang YW. Design of phosphorene/graphene heterojunctions for high and tunable interfacial thermal conductance. Nanoscale (2018) 10:19854–62. doi:10.1039/c8nr06110f
37. Liu X, Gao J, Zhang G, Zhang Y-W. MoS2-graphene in-plane contact for high interfacial thermal conduction. Nano Res (2017) 10:2944–53. doi:10.1007/s12274-017-1504-8
38. Desai SB, Seol G, Kang JS, Fang H, Battaglia C, Kapadia R, et al. Strain-induced indirect to direct bandgap transition in multilayer WSe2. Nano Lett (2014) 14:4592–7. doi:10.1021/nl501638a
39. Yu C, Zhang G, Zhang Y-W, Peng L-M. Strain engineering on the thermal conductivity and heat flux of thermoelectric Bi2Te3 nanofilm. Nano Energy (2015) 17:104–10. doi:10.1016/j.nanoen.2015.08.003
40. Zhang G, Zhang Y-W. Strain effects on thermoelectric properties of two-dimensional materials. Mech Mater (2015) 91:382–98. doi:10.1016/j.mechmat.2015.03.009
41. Guo Z, Zhang D, Gong X-G. Thermal conductivity of graphene nanoribbons. Appl Phys Lett (2009) 95:163103. doi:10.1063/1.3246155
42. Wei N, Xu L, Wang H-Q, Zheng J-C. Strain engineering of thermal conductivity in graphene sheets and nanoribbons: A demonstration of magic flexibility. Nanotechnology (2011) 22:105705. doi:10.1088/0957-4484/22/10/105705
Keywords: biphenylene, thermal conductivity, biphenylene/graphene heterostructure, interfacial thermal conductance, strain effect
Citation: Cui Q, Ren K, Zheng R, Zhang Q, Yu L and Li J (2022) Tunable thermal properties of the biphenylene and the lateral heterostructure formed with graphene: A molecular dynamics investigation. Front. Phys. 10:1085367. doi: 10.3389/fphy.2022.1085367
Received: 31 October 2022; Accepted: 14 November 2022;
Published: 25 November 2022.
Edited by:
Guangzhao Wang, Yangtze Normal University, ChinaReviewed by:
Chengyong Zhong, Chongqing Normal University, ChinaTang Yong, Huanghuai University, China
Copyright © 2022 Cui, Ren, Zheng, Zhang, Yu and Li. This is an open-access article distributed under the terms of the Creative Commons Attribution License (CC BY). The use, distribution or reproduction in other forums is permitted, provided the original author(s) and the copyright owner(s) are credited and that the original publication in this journal is cited, in accordance with accepted academic practice. No use, distribution or reproduction is permitted which does not comply with these terms.
*Correspondence: Jianping Li, c3p5bGpwMDE3MEBzenB0LmVkdS5jbg==