Study on external cavity diode laser with a wide mode-hopping free tuning range
- 1Ceyear Technologies Co., Ltd, Qingdao, China
- 2Science and Technology on Electronic Test and Measurement Laboratory, Qingdao, China
- 3School of Electronic Engineering, Xidian University, Xi’an, China
- 4School of Information Science and Engineering, Shandong University, Qingdao, China
A wide mode-hopping free and narrow linewidth tunable laser diode source with a Littman-Metcalf arrangement based on a diffraction grating is presented. Several experiments are carried out to demonstrate the tuning characteristics and spectral linewidth of the proposed external-cavity diode laser source. A wide no mode-hopping continuous wavelength tuning range about 59.13 nm in ultra-C-band with a narrow spectral linewidth of less than 100 kHz is obtained. An optical signal to noise ratio of more than 65 dB and an output power more than 14.8 dBm over the whole tuning range can also be realized in a long-term free running condition. The proposed tunable laser allows simultaneously mode-hopping free and narrow linewidth tunable radiation, thus opening a door for practical application such as coherent detection.
Introduction
Compared to most other types of tunable laser (e.g., distributed Bragg reflector laser, vertical cavity surface emitting laser, and distributed feedback laser), external cavity diode laser (ECDL) has been broadly studied and acted as a prior selected laser source for its prestigious characteristics such as narrow linewidth, broad wavelength tuning range, single mode, and compactness. It therefore makes the ECDL a versatile measuring tool for many applications in fields including optical frequency-domain reflectometry (OFDR), coherent optical communication, precision spectroscopy, and atomic physics [1–7]. Particularly, most applications require that the output wavelength of the ECDL can be scanned continuously over time. Consequently, it is critical to build and maintain an ECDL system with a wide mode-hopping free (MHF) tuning range.
Shortly after the first tunable ECDL configuration was developed in the early 1980s [8], many novel external-cavity designs have been presented. Probably the most classical designs of the tunable ECDL can be divided into two types, the Littrow-type external-cavity configuration [9, 10] and the Littman-Metcalf-type external-cavity configuration [11, 12]. In the former configuration, the ECDL system basically consists of a laser diode (or called gain chip) and a wavelength selected element (or called dispersion element). The latter configuration is mainly composed of a gain chip, a dispersion element, and a cavity mirror added. These two classical structures use diffraction gratings for wavelength selection. K. Fedorova et al reported a 1494–1667 nm widely tunable coherent radiation based on an InGaAs-InP strained multiple quantum well (MQW) laser chip generated from Littrow-type external-cavity configuration [13]. The maximum side mode suppression ratio (SMSR) was about 50 dB at around 1550 nm with the linewidth of less than 0.4 nm. Yuan et al demonstrated an InAs/InP quantum-dot (QD) laser system with a classical Littrow-type external-cavity design operating at pulsed injection current, the tunable range of which reached 190 nm [14]. Wang et al proved that a proper high diffraction grating groove number (1200 lines/mm) and first-order diffraction efficiency (91%) can improve laser performance and achieve wider tuning range (209.9 nm) [15]. However, these works have barely focused on the MHF tuning characteristics. It also should be noted that the wavelength tuning through the dispersion element in Littrow-type external-cavity structure results in the displacement of the 0th-order output beam. Laterly, Guo et al successfully demonstrated a new and enhanced structure for mirror-grating Littrow-type ECDL to obtain a large MHF tuning range (4.34 nm) and remain the lateral displacement of the output beam (0.033 mm) almost unchanged when the diffraction grating was tuned [16]. Obviously, the presented mirror-grating method require rather complicated monitoring system in order to guarantee that the mirror and the diffraction grating rotate synchronously, and keep parallel to each other. These are not the cases in the Littman-Metcalf-type external-cavity design. The synchronization of the external-cavity mode and the diffraction grating-feedback wavelength is guaranteed by the special external-cavity configuration. K. Repasky et al studied the effect of the electronic feedback loop on the cavity resonance condition as the laser was tuned [17]. With electronic feedback, it can be found that the continuous tuning range can be extended to over 65 GHz based on the Littman-Metcalf external-cavity arrangement. Gong et al proposed a unique method to extend the MHF tuning range of a Littman-Metcalf-type ECDL by synchronous tuning with mode matching [18]. The maximum no mode-hop tuning range of 78 GHz was obtained operating at 774.5 nm with an unoptimized reflector pivot position. Meanwhile, some groups focus on studying the tuning performance of Littman-based ECDL with different hybrid methods. A high-power ECDL centered at 1329 nm with a tunable range of 111 nm was reported by K. Leung, and the total average output power was 131 mW, which was performed by incorporating two serial semiconductor optical amplifiers (SOA) in the laser cavity [19]. The wavelength tuning was realized by a compact 72-facet polygon scanner. Zhang et al presented a modified Littman-Metcalf structure based on a simple single-axis-MEMS mirror and a fused silica etalon [20]. The measured wavelength tuning range of the proposed ECDL was about 40 nm in C-band. Although there have been some reports on the widely tunable diffraction grating-coupled ECDL, the continuous wavelength tuning with a wide MHF tuning range seems less reported.
In this work, experimental results of a wide tunable external-cavity diode laser with classical Littman-Metcalf set-up are reported. The output power is about 16.5 dBm at the emitting wavelength of 1550 nm, and the maximum SMSR exceeds 60 dB is obtained in almost the full tuning spectral range of the presented Littman-Metcalf arrangement. Furthermore, the output wavelength can be changed continuously in a range of 59.13 nm without mode-hopping with an optical signal to noise ratio of more than 65 dB and an output power more than 14.8 dBm over the entire tuning range, which is about 95 times enhancement compared with the mode matching method [18]. The lasing wavelength is tunable and operating in single frequency with a narrow linewidth of no more than 100 kHz. Its output beam has good directional stability when tuned.
Experimental setup
The experimental setup of the proposed tunable ECDL is built in a Littman-Metcalf configuration, as shown schematically in Figure 1. The tunable ECDL consists of a commercially available single angled facet gain chip (Thorlabs, SAF1550S2) and an external cavity, which is generally formed by a molded aspherical lens (Edmund, 87155), a blazed diffraction grating (Newport Richardson, 33025FL01-155R, the S and P plane average diffraction efficiency of the m = 1 spectral order around 1500 nm is about 70%), a homemade gold mirror and a linear motor. The single angled facet gain chip with typical beam divergence of 31° in y-direction (transverse) and 17° in x-direction (lateral) fixed on a thermoelectric cooler (TEC) is coated a 10% reflectivity film on its normal facet (or called left/rear facet) and a 0.005% antireflection (AR) film on its angled facet (or called right/front facet). A continuous wave (CW) light from the gain chip is collimated by a molded aspherical lens (MAL) with a numerical aperture (NA) of 0.55 and a focal length of 4.51 mm, and then is incident on a 900 lines/mm blazed diffraction grating. The first-order diffraction of the blazed grating is diffracted on the surface of the cavity mirror, and then reflected back to the same blazed grating mounted in Littman-Metcalf configuration. Finally, the diffractive beam is coupled back into the single angled facet gain chip to form the resonator. The operation from one wavelength (mode) to another wavelength is achieved by rotating the gold mirror driven by a piezoelectric transducer (PZT) motor. The output of the selected wavelength is taken from the fiber output and is divided into two equal parts by a 50/50 commercial optical coupler (OC). After passing through the 3 dB OC, the power of light coupled from the external cavity could be measured using an optical power meter (Thorlabs, PM100D). Meanwhile, an optical spectrum analyzer (OSA: Yokogawa, AQ6370D) with a resolution of 20 pm. is applied to monitor the spectral changes of the ECDL scheme. In order to verify the ability to achieve a wide MHF tuning range at the same time, a wavelength meter (Yokogawa, AQ6151B) is used to obtain the MHF traces. It is worth noting that a variable optical attenuator (VOA) with an attenuation of 9 dBm is applied to prevent the OSA or the wavelength meter from being damaged due to the excessive output power.
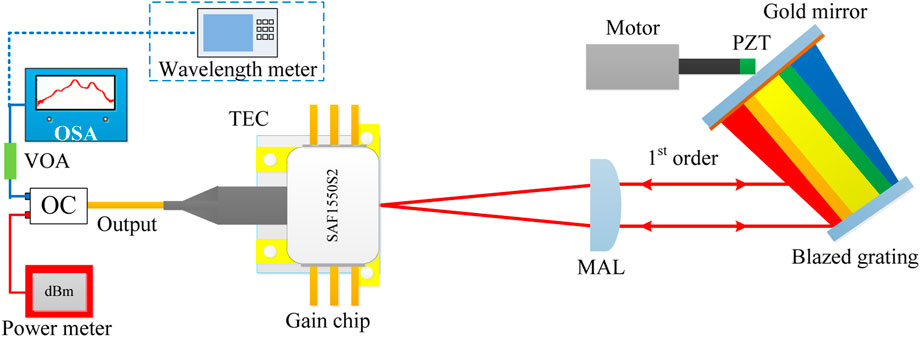
FIGURE 1. Schematic diagram of the ECDL built in the Littman-Metcalf configuration. TEC, thermoelectric cooler; MAL, molded aspherical lens; PZT, piezoelectric transducer; OC, optical coupler; VOA: variable optical attenuator.
Experimental results and discussion
After adding the external cavity to the commercially available single angled facet gain chip, MAL focal adjustment and wavelength selected element alignment are carried out to optimize the optical feedback. Optimum alignment is accomplished when the gain chip threshold current is reduced to a minimum value [21]. Figure 2A shows the output power characteristics of the ECDL versus the injection current (the step is 10 mA) at the absence of the commercial optical coupler, the output power increases linearly with the increasing of injection current. The output power at the maximum injection current of the home made laser diode driver (450 mA) is 45.3 mW. The slope efficiency and the gain chip threshold current are approximately 0.11 mW/mA and 60 mA, respectively. However, it should be noted that the output power will suddenly jump when the injection current tuned up from 300 mA to 310, 380–390, and 440–450 mA, respectively. It may result from current-induced mode jumps.
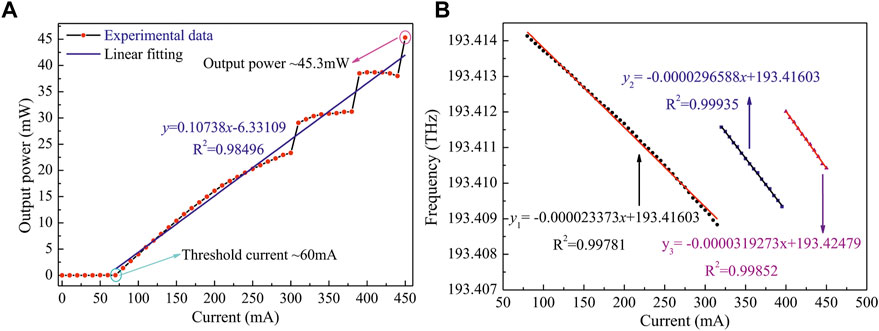
FIGURE 2. (A) Measurement of the output power characteristics of the ECDL as a function of the injection current at 1550 nm. (B) Measurement output frequency shift as a function of the injection current in the Littman-Metcalf-type external-cavity configuration.
To fully demonstrate the effect of injection current on the tuning characteristics of the ECDL output frequency (wavelength), the injection current is increased from 80 to 450 mA with 5 mA/step. Then the relation between injection current and output frequency in the Littman-Metcalf external cavity configuration is plotted in Figure 2B, where linear regression is performed to obtain the frequency tuning rate of the injection current, respectively. As displayed in Figure 2B, the frequency tuning rate of the injection current are determined to be approximately 0.0234 GHz/mA, 0.0297 GHz/mA and 0.0319 GHz/mA, respectively. Furthermore, it can be observed that the proposed ECDL has a mode hopping interval, which can reasonably explain the phenomenon in Figure 2A. The MHF interval with respect to the injection current adjustment range is changed when injection current changes and moves to the shorter MHF range as the injection current increases. Therefore, it is necessary to avoid this mode hopping interval when the ECDL is working.
Subsequently, the basic characteristics of the proposed ECDL are measured. Figure 3A displays the optical spectrum of the present ECDL operating 1,550.14 nm, single-mode operation with high optical signal to noise ratio (OSNR) of more than 65 dB can be observed. The designed total physical lengths of the laser cavity are changed to obtain superimposed optical spectra for different resonance wavelengths. The tunable wavelength range is about 59.13 nm from 1,520.82 nm to 1,579.95 nm, as shown in Figure 3B. What’s more, it can be seen in Figure 3B that the ECDL exhibits output power more than 14.8 dBm (taking the fixed attenuation value into account) over the whole tuning range when the injection current is about 410 mA while maintaining a high OSNR over 65 dB. Later, an experiment is carried out to demonstrate the wavelength long-term stability of the present ECDL. A wavelength meter is adopted to measure the wavelength fluctuations of the present ECDL during free running. The monitoring ambient temperature fluctuates by roughly 1 °C about an average roughly 22 °C, and the results of the wavelength long-term stability are obtained over period of 6 h with 0.1 h step. As depicted in Figure 3C, the maximum deviation in the ECDL wavelength is only 10 pm indicating an excellent passive wavelength long-term stability. Under the similar experimental conditions, the output power stability of the laser is measured. Then the relation between the output power and free running time in the proposed ECDL arrangement is plotted in Figure 3D. It can be observed that from Figure 3D that the maximum deviation in the output power is only 0.02 dB indicated good power stability.
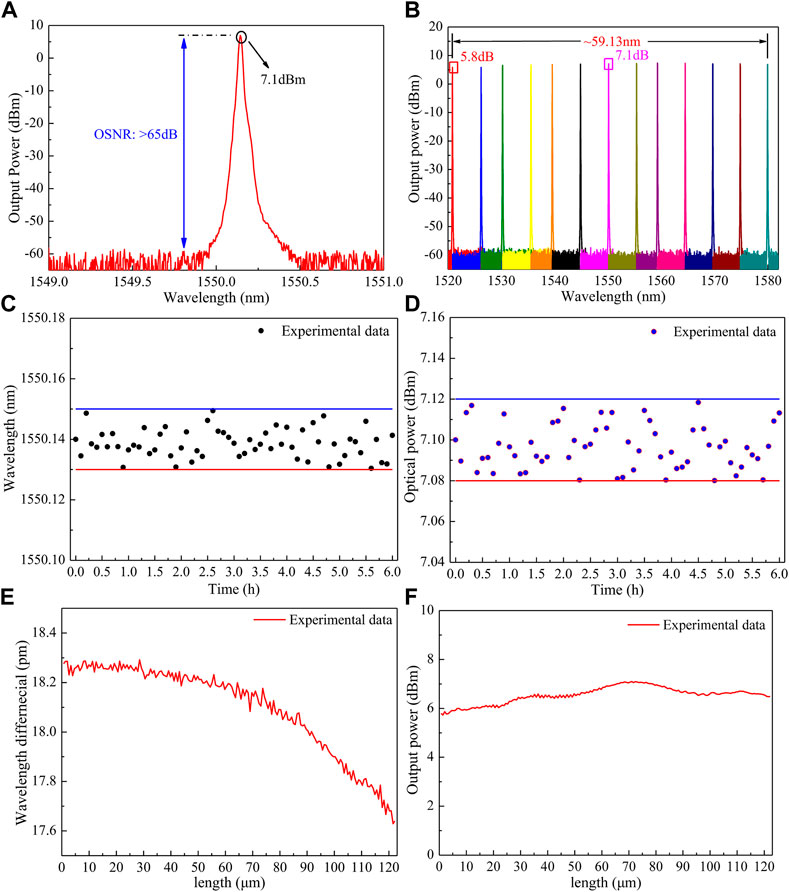
FIGURE 3. (A) The optical spectrum of the present ECDL operating 1,550.14 nm. (B) The superimposed optical spectra for different resonance wavelengths. (C) The wavelength long-term stability of the present ECDL. (D) The output power stability of the laser. (E) The mode-hopping free performance of the proposed ECDL. (F) The relationship between the optical output power and the physical length of the ECDL cavity.
To fully demonstrate the mode-hopping free performance of the proposed ECDL system, a corresponding experiment is implemented to valid the basis characteristic. In the current implementation, the overall physical length of the ECDL cavity is designed to be about 50 mm, namely from the gain chip rear (left) output facet to the gold mirror front facet, and corresponds to an axial mode spacing of 23.1 pm operating at 1520 nm [21]. The mode spacing is changed when laser wavelength (in vacuum) changes, and moves to the bigger axial mode spacing as the wavelength increases. The maximum axial mode spacing is about 24.9 pm operating at 1580 nm. For a given laser resonator mode, an about 0.76 μm variation in the physical length of the ECDL cavity causes a relative wavelength detuning of 23.1 pm. Therefore, as displayed in Figure 3E, when the variations of the total physical length are changed with 0.664 μm step, the variations of the adjacent wavelength subtract are less than one mode spacing, which indicates a mode-hopping free tunable output is ability to be realized. Figure 3F illustrates the relationship between the optical output power and the physical length of the ECDL cavity. It can be seen that the measured trace is smooth (no suddenly power jump) which also demonstrates a mode-hopping free tunable output can be achieved.
At last, the spectral linewidth performance of the proposed ECDL system is illustrated using traditional delayed self-heterodyne (DSH) arrangement with a total length of 20 km single-mode fiber (Corning, SMF28e) and an 80 MHz fibre-coupled acousto-optic modulator (AOM) which is fabricated by the Gooch & Housego [22, 23]. The spectral linewidth of the beat signal is depicted in Figure 4A. Thanks to the relative long laser cavity and low noise direct current (DC) source, the full width at half maximum (FWHM) of the Lorentzian fit is roughly 176 kHz operating at 1550 nm. In addition, as plotted in Figure 4B, the spectral linewidth of the proposed ECDL is less than 100 kHz over the entire tuning range. Notice that the obtained spectral linewidth of the designed lasing system in the central part of the whole tuning range is narrower than that in the two edges of the whole tuning range. This is because the resonator wavelengths in the two edges of the whole tuning range have larger diffraction angles which will result in an extra wavelength-dependent coupling loss.
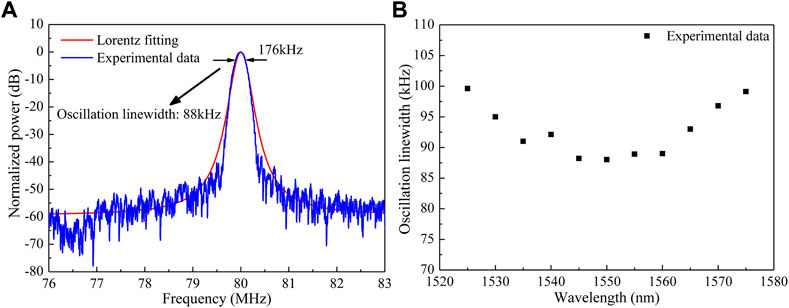
FIGURE 4. (A) Delayed self-heterodyne spectra of the proposed ECDL operating at wavelength of 1550 nm. (B) The linewidth vs oscillation wavelength. The linewidth performance is measured using the traditional DSH arrangement with a total length of 20 km single-mode fiber and an 80 MHz fibre-coupled AOM.
Conclusion
In conclusion, a wide mode-hopping free and narrow linewidth tunable laser source is designed, which is based on a classical Littman-Metcalf configuration. Meanwhile, the tuning characteristics and spectral linewidth of the proposed external-cavity diode laser are investigated experimentally. A wide no mode-hopping continuous wavelength tuning range about 59.13 nm in ultra-C-band (namely, 1,520.82–1,579.95 nm) with an optical signal to noise ratio of more than 65 dB and an output power more than 14.8 dBm over the whole tuning range can be achieved in a long-term free running. The spectral linewidth performance of the designed tunable laser source measured using delayed self-heterodyne method is less than 100 kHz. With the help of this designed tunable laser source, it has the potential in the application of higher rate (800 Gbit/s and 1 Tbit/s) coherent systems with higher-order modulation formats (coherent optical orthogonal frequency division multiplexing). Future work shall focus on the optimization of the length of the laser cavity design to further reduce the spectral linewidth.
Data availability statement
The raw data supporting the conclusion of this article will be made available by the authors, without undue reservation.
Author contributions
Conceptualization, LS, AZ, SQ, and LH; Methodology, LS, AZ, and LH; Software, PL, JJ, and ZZ; Validation, AZ, ZL, and BY; Formal analysis, SQ and JL; Investigation, YW and LS; Resources, LH and LS; Data curation, SQ; Writing—original draft preparation, LS and SQ; Writing—review and editing, LH and LS; Visualization, LS; Supervision, LH; Project administration, LS and LH; Funding acquisition, LS, SQ, and LH. All authors have read and agreed to the published version of the manuscript.
Funding
This research was funded in part by National Key Research and Development Plan (2022YFF0705900); In part by National Natural Science Foundation of China (61605034); In part by Qingdao Postdoctoral Applied Research Project (20266153, 21290111); In part by Special Support for Postdoctoral Creative Funding in Shandong Province (202103076); And in part by Science and Technology on Electronic Test and Measurement Laboratory Foundation (ZF332).
Acknowledgments
Thanks to Shuai Zhou and Jilei Han for their support during the experiments.
Conflict of interest
Authors AZ, SQ, LS, LH, ZL, JJ, ZZ, BY, PL, JL, and YW are employed by Ceyear Technologies Co., Ltd.
Publisher’s note
All claims expressed in this article are solely those of the authors and do not necessarily represent those of their affiliated organizations, or those of the publisher, the editors and the reviewers. Any product that may be evaluated in this article, or claim that may be made by its manufacturer, is not guaranteed or endorsed by the publisher.
References
1. Xu P, Yu X, Chen Z, Sheng L, Liu J, Zhou S, et al. Distributed refractive index sensing based on bending-induced multimodal interference and Rayleigh backscattering spectrum. Opt Express (2021) 29(14):21530–8. doi:10.1364/OE.430637
2. Yang X, Yin Y, Li X, Sheng L, Liu J, Zhou S, et al. External cavity diode laser as a stable-frequency light source for application in laser cooling of molecules. Chin Opt Lett (2016) 14(7):071403. doi:10.3788/COL201614.071403
3. Robins N, Lance A, Close J, Sheng L, Liu J, Zhou S, et al. Piezo-locking a diode laser with saturated absorption spectroscopy. Appl Opt (2008) 47(28):5163–6. doi:10.1364/AO.47.005163
4. Schioppo M, Brown R, McGrew W, Hinkley N, Fasano R, Beloy K, et al. Ultrastable optical clock with two cold-atom ensembles. Nat Photon (2017) 11(48):48–52. doi:10.1038/NPHOTON.2016.231
5. Ma Y, Yang Q, Tang Y, Chen S, Shieh W. 1-Tb/s single-channel coherent optical OFDM transmission over 600-km SSMF fiber with subwavelength bandwidth access. Opt Express (2009) 17(28):9421–7. doi:10.1364/OE.17.009421
6. Duan L, Lukin M, Cirac J, Zoller P. Long-distance quantum communication with atomic ensembles and linear optics. Nature (2011) 414(413):413–8. doi:10.1038/35106500
7. Doringsho K, Ernsting I, Rinkle R, Schiller S, Wicht A. Low-noise, tunable diode laser for ultra-high-resolution spectroscopy. Opt Lett (2007) 32(413):2876–8. doi:10.1364/OL.32.002876
8. Lang R, Kobayashi K. External optical feedback effects on semiconductor injection laser properties. IEEE J Quan Electron (1980) 16(413):347–55. doi:10.1109/jqe.1980.1070479
9. Arnold A, Wilson J, Boshier M. A simple extended-cavity diode laser. Rev Scientific Instr (1998) 69(413):1236–9. doi:10.1063/1.1148756
10. Hawthorn C, Weber K, Scholten R. Littrow configuration tunable external cavity diode laser with fixed direction output beam. Rev Scientific Instr (2001) 72(413):4477–9. doi:10.1063/1.1419217
11. Harvey K, Myatt C. External-cavity diode laser using a grazing-incidence diffraction grating. Opt Lett (1991) 16(413):910–2. doi:10.1364/OL.16.000910
12. Sheng L, Zhang A, Qiao S, Huang L, Zhang Z, Liu Z, et al. Study of longitudinal allowance error for mode-hopping free external cavity tunable semiconductor lasers. Eletro-optic Technol Appl (2021) 36(5):66–72.
13. Fedorova K, Cataluna M, Kudryashov I, Khalfin V, Rafailov E. Broadly tunable InGaAsP-InP strained multi-quantum-well external cavity diode laser. IEEE Photon Technol Lett (2010) 22(413):1205–7. doi:10.1109/LPT.2010.2051661
14. Yuan H, Gao F, Yang T. Ultra-broadband tunable single- and double-mode InAs/InP quantum dot external-cavity laser emitting around 1.65μm. Opt Lett (2018) 43(413):3025–8. doi:10.1364/OL.43.003025
15. Wang Y, Wu H, Chen C, Zhou Y, Wang Y, Liang L, et al. An ultra-high-SMSR external-cavity diode laser with a wide tunable range around 1550 nm. Appl Sci (2019) 9:4390. doi:10.3390/app9204390
16. Guo H, Olamax G. Analysis of no mode-hop tuning of mirror-grating external-cavity diode laser. Opt Commun (2018) 421:90–3. doi:10.1016/j.optcom.2018.03.074
17. Repasky K, Nehrir A, Hawthorne J, Switzer G, Carlsten J. Extending the continuous tuning range of an external-cavity diode laser. Appl Opt (2006) 45(35):9013–20. doi:10.1364/AO.45.009013
18. Gong H, Liu Z, Zhou Y, Zhang W. Extending the mode-hop-free tuning range of an external-cavity diode laser by synchronous tuning with mode matching. Appl Opt (2014) 53(33):7878–84. doi:10.1364/AO.53.007878
19. Leung M, Mariampillai A, Standish B, Lee K, Munce N, Vitkin I, et al. High-power wavelength-swept laser in Littman telescope-less polygon filter and dual-amplifier configuration for multichannel optical coherence tomography. Opt Lett (2009) 34(18):2814–6. doi:10.1364/OL.34.002814
20. Zhang D, Zhao J, Yang Q, Liu W, Fu Y, Li C, et al. Compact MEMS external cavity tunable laser with ultra-narrow linewidth for coherent detection. Opt Express (2012) 20(18):19670–82. doi:10.1364/OE.20.019670
21. Zhang L, Liu T, Chen L, Xu G, Jiang C, Liu J, et al. Development of an interference filter-stabilized external-cavity diode laser for space applications. Photonics (2020) 7(12):7010012. doi:10.3390/photonics7010012
22. Zhao Z, Bai Z, Jin D, Qi Y, Ding J, Yan B, et al. Narrow laser-linewidth measurement using short delay self-heterodyne interferometry. Opt Express (2022) 30(17):30600–10. doi:10.1364/OE.455028
Keywords: tunable laser, mode-hopping free, littman-metcalf arrangement, narrow linewidth, coherent detection
Citation: Zhang A, Qiao S, Sheng L, Huang L, Liu Z, Ju J, Zhang Z, Yin B, Li P, Liu J and Wei Y (2022) Study on external cavity diode laser with a wide mode-hopping free tuning range. Front. Phys. 10:1093179. doi: 10.3389/fphy.2022.1093179
Received: 08 November 2022; Accepted: 21 November 2022;
Published: 01 December 2022.
Edited by:
Zhenxu Bai, Hebei University of Technology, ChinaReviewed by:
Xiaobo Hu, Zhejiang Sci-Tech University, ChinaXiaotao Mi, Changchun Institute of Optics (CAS), China
Copyright © 2022 Zhang, Qiao, Sheng, Huang, Liu, Ju, Zhang, Yin, Li, Liu and Wei. This is an open-access article distributed under the terms of the Creative Commons Attribution License (CC BY). The use, distribution or reproduction in other forums is permitted, provided the original author(s) and the copyright owner(s) are credited and that the original publication in this journal is cited, in accordance with accepted academic practice. No use, distribution or reproduction is permitted which does not comply with these terms.
*Correspondence: Liwen Sheng, shengliwen2008@163.com; Lin Huang, huanglin@ceyear.com