Improvement of Coral Sand With MICP Using Various Calcium Sources in Sea Water Environment
- Key Laboratory of Ministry of Education for Geomechanics and Embankment Engineering, Hohai University, Nanjing, China
Microbial-induced calcium carbonate precipitation (MICP) is a promising technique for strengthening soil. The influences calcium sources (calcium chloride, calcium acetate, and calcium nitrate) and seawater/freshwater environment on the improvement of coral sand were studied. A microbial-induced calcium carbonate precipitation (MICP) aqueous solution experiment was carried out to explore the influence of the different calcium source and seawater/freshwater on the relationship between calcium carbonate precipitation and time. Coral sand column treatment tests were conducted to investigate the environmental impact on soil strengthening. The results show that the actual production of calcium carbonate is 20% lower than the theoretical value. Calcium chloride was found to be the optimal calcium source in terms of the seawater environment affect 2% of calcium carbonate production compared with the freshwater environment. The results of tests on three different calcium sources (calcium chloride, calcium acetate, and calcium nitrate) showed that seawater adversely affected the unconfined compression strength (UCS) and the tensile splitting strength (TSS) of strengthened specimens compared with freshwater. The UCS and TSS values in the freshwater environment were approximately 30–45% higher than those in the seawater environment. Nevertheless the strengthened specimens in the seawater environment exhibited high strength, and the MICP improvement effect can be guaranteed. Therefore, The MICP technology can be used to improve coral sand for construction work in seawater environments.
1 Introduction
Biomineralization has been extensively studied over the recent decades [1–4]. The mechanism of microbial-induced calcium carbonate precipitation (MICP) involves bacterial hydrolysis of urea to metabolites, which react with ambient substances to produce a large quantity of precipitates. Thus, microorganisms are used to facilitate urea hydrolysis to produce carbonate and ammonium ions. In an alkaline environment, carbonate ions in solution react with calcium ions to form calcium carbonate [5, 6]. Studies have been performed to investigate the effects of microbial characteristics (including the type of bacteria), the cementation solution, temperature, salinity, and the pH on MICP-treated sand [7–13]. The environment and the calcium source are important factors in reactions. Bacterial growth is affected by freshwater and seawater [14]. Different types of crystals are induced by different calcium sources [15, 16]. MICP has been studied as a potential solution to environmental problems and soil stabilization in many engineering fields, such as the remediation of heavy-metal-contaminated soil [17, 18], the mitigation of soil liquefaction [19–21], reduction of permeability and improve the durability of concretes material [22], the sequestration of atmospheric CO2 [23], and the suppression of sand dust emissions [24].
Coral sand is mainly composed of calcium carbonate (almost 95%), It is a special kind of sand deposited and mixed by calcareous biological skeleton and other marine debris under temperature and pressure [25, 26]. As calcium carbonate has internal pores and a unique mineral composition, calcium carbonate particles are easily broken, and coral sand is considerably lower in strength than silica sand [27, 28]. The use of traditional ground improvement techniques, such as cementing, lime grouting, or other types of chemical pulp, in site construction can pollute the sea environment [29, 30]. In addition, applying these traditional methods to solidify coral sand involves numerous challenges, including the absence of engineering classification for coral sand, poor in situ drilling results and inadequate geophysical testing methods [31]. Therefore, microbial-induced calcium carbonate precipitation (MICP) has been introduced to improve coral sand. It has been reported that bacteria can form crystals in water, which explains the extensive deposits of CaCO3 in places such as Grand Bahama [32]. Using common microbial bacteria to solidify loose sand will increase the required ratio of urea to calcium sources. The initial shear stiffness and shear strength of the treated samples increased, and the failed samples exhibited the characteristics of strain-softened [33]. Sporosarcina pasteurii was used as a nucleation site to study the physical and biochemical properties of calcium carbonate precipitation [34]. Four bacterial strains—Thalassospira sp, Halomonas sp, Bacillus pumilus, and Pseudomonas qrimontii—were isolated from sediments and deep seawater, and the induced precipitates contained different percentages of magnesium calcite (including MgCO3 and CaCO3), aragonite and monohydrocalcite, thus a hypothesis was formulated that carbonate precipitation can enable the survival of bacteria populations in some habitats [35]. Wei et al [36]. sequentially investigated different precipitates induced by isolated marine bacteria. Previous studies have focused on enhancing the mechanical properties of coral sand in freshwater environments. In recent years, numerous studies have been conducted on the biological treatment of coral sand, especially in seawater environments.
The main objective of this study is to investigate the influence of the environment and the calcium source on strengthening coral sand. Two control groups (freshwater and seawater) were used in this study. Preliminary experiments were conducted on aqueous solutions containing seawater. Then, experiments were conducted to obtain the stress-strain curve and failure modes of strengthened specimens. X-ray diffraction (XRD) analysis and scanning election microscopy (SEM) were conducted on the obtained calcium carbonate crystals. Different calcium carbonate samples exhibited quite different crystal forms and microstructures, showing that the influence of MICP technology on coral sand specimens requires further study. Experiments were performed using three common calcium sources (calcium chloride, calcium carbonate, and calcium nitrate) and two different environments (seawater and freshwater) to determine the effects of the calcium source and the environment on the quantity and structure of the crystals formed. The results of this study can serve as a reference for future coral sand column tests.
2 Experimental Investigation
2.1 Materials
2.1.1 Characteristics of Loose Coral Sand
Coral sand was obtained from the Sanya region of the South China Sea. The sand particles were full of intragranular pores, and the calcium carbonate content was more than 95%. The mechanical properties of the coral sand particles were as follows: specific gravity Gs = 2.7–2.8 g/mm³, porosity e = 0.94–1.01, dry density ρd = 1.38–1.41 g/cm³, average grain diameter D50 = 0.36, coefficient of uniformity Cu = 5.49, and coefficient of curvature Cc = 7.87.
2.1.2 Bacterial Suspension
Sporosarcina pasteurii (ATCC 11859) was used as the urease microorganism. The culture medium contained the following components per liter of deionized water: 20 g of yeast extract, 10 g of NH4Cl, 2.4 g of NiCl·6H2O, 1 g of MnSO4·H2O; the medium had a pH of 8.5-9 and was sterilized at 121°C for 30 min, followed by cooling to 25°C. The organisms were then incubated under aerobic conditions at 30°C at 121 r/min for approximately 24 h. A bacterial suspension was grown to a urease activity of 1.45 mM urea/min.
2.1.3 Cementation Solution
A total of 6 groups of different cementation solutions were prepared with freshwater (FW)/seawater (SW) and different calcium sources: CaCl2 with freshwater (CF), CaCl2 with seawater (CS), Ca(CH3COO)2 with freshwater (HW), Ca(CH3COO)2 with seawater (HS), Ca(NO3)2 with freshwater (NW), and Ca(NO3)2 with seawater (NS). Deionized water produced by laboratory water purification was used as freshwater, and the seawater was obtained from the Sanya region. Both the freshwater and seawater were disinfected with ultraviolet light before the experiments were conducted. Different calcium sources, corresponding to CaCl2, Ca(CH3COO)2, and Ca(NO3)2, were easily obtained and used to study the effects of different environments on MICP. The cementation solution was a mixture of urea and the calcium source. A 1:1 ratio of the calcium source and urea, both at a concentration of 0.5 M, was used. The cementation solution was not sterilized. The composition of the liquid medium used for each group of samples is shown in Table 1.
2.2 Methods
2.2.1 Preliminary Experiment
Tests on MICP aqueous solutions were conducted using six groups of specimens under different experimental conditions. Each solution consisted of 5 ml of the bacterial suspension and 250 ml of the cementation solution that were mixed in a conical flask. A preliminary investigation of the effects of seawater and the different calcium sources was conducted, ensuring sufficient contacting between the bacterial suspension and the cementation solution during the entire test process. The flasks containing the solutions were placed on a magnetic stirring apparatus. The same rotating speed was maintained for all the solution mixtures. A volume of 1 ml was extracted from each sample mixture and analysed to determine the Ca2+ consumption, pH and final production of calcium carbonate.
2.2.2 MICP Treatment for Strength Enhancement of Loose Coral Sand
The same bacterial suspension was used in sand column experiments to measure the Ca2+ consumption and the final production of calcium carbonate. The sand column moulds were fabricated from a stainless-steel wire sieve (diameter: 42 mm, height: 79 mm). The coral sand column was prepared by sand pourer. All the samples had an approximately 0.5 pore volume and were grouted with the bacteria solution. A volume of 800 ml of the cementation solution was used per beaker. The samples were soaked in a 0.5 M cementation solution in glass beakers, which were placed on a magnetic stirring apparatus. A seawater environment was simulated by maintaining rotors at the same speed in the glass beakers. The sand columns were left in the cementation solution for 10 days. A schematic of the experimental apparatus is shown in Figure 1.
2.2.3 Mechanical Tests
The unconfined compression strength (UCS) and tensile splitting strength (TSS) are important indexes used to measure the effect of reinforcements in a sample. Cylindrical specimens of coral sand with a 44-mm diameter and a 79-mm height reinforced by MICP were tested. Before the experiment each of specimen was dried to dry state. The UCS and TSS of the coral sand column specimens were tested using an Instron-3367 electronic universal testing machine. The UCS test was conducted under strain-controlled conditions at a uniform loading rate of 1 mm/min. In a Brazilian test, two pads are placed on the top and bottom of a cylindrical sample. Opposing linear loads are applied simultaneously until splitting failure occurs along the sample diameter. The splitting strength of the sample is thus obtained. The Brazilian test was conducted under strain-controlled conditions at a uniform loading rate of 0.25 mm/min in this study.
2.2.4 Microscale Analysis
Six samples of calcium carbonate precipitates were ground to powders. The sample mineralogy was determined by subjecting the powders to XRD analysis (using a Bruker D8 advance powder X-ray diffraction slow scanning device). The accelerating voltage was set to 40 kV, and an electric current of 35 mA was used. Scans were run from 10° to 80° 2θ at 10°/min. After completion of the test, the sample components were comparatively analysed using Jade 6.5 software. The sample morphology was determined by SEM (using a Nano Nava S230 field emission electron scanning microscope produced by FEI). A Cressington 208HR sputter-coater was used to prepare the samples for SEM analysis.
3 Results
The results of several coral sand column experiments were used to determine the effect of a seawater environment on samples treated with MICP using different calcium sources (calcium chloride, calcium acetate, and calcium nitrate).
3.1 pH
During the preliminary test (Figure 2), the pH values of six samples in group A were measured to study the differences between freshwater and seawater environments. The carbon dioxide produced by bacterial respiration reduced the pH of the solution [37]. As all the final products were acidic, the pH values of all the specimens were less than 7. Ammonium chloride was the most acidic substance, followed by ammonium nitrate, and ammonium acetate was neutral.
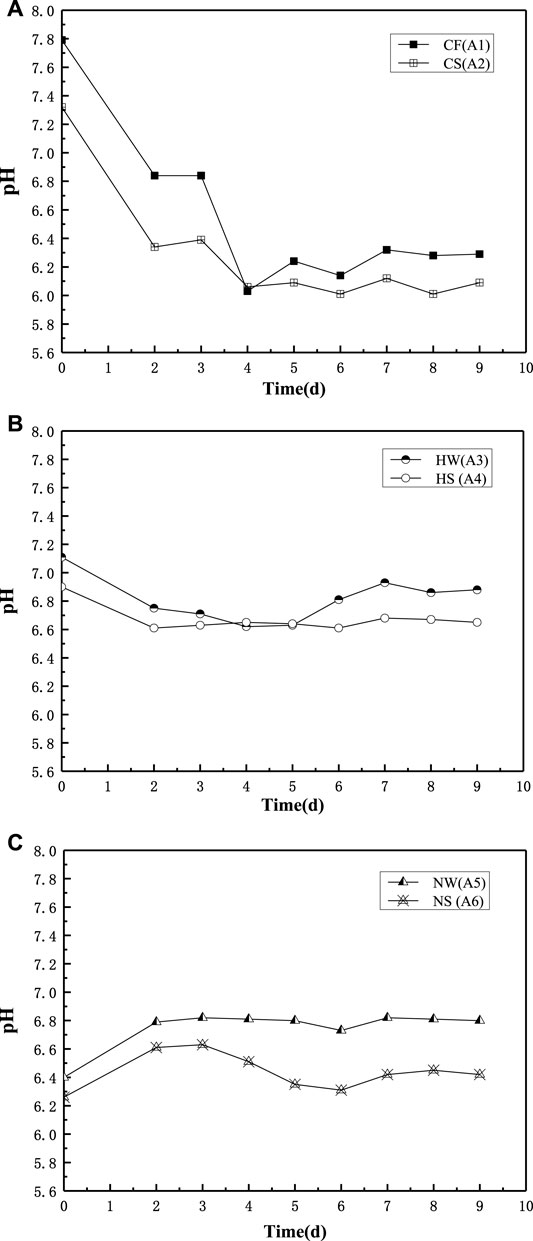
FIGURE 2. pH in Group A of freshwater and seawater environment. (A) Group A CaCl2, (B) Group A Ca(CH3COO)2; (C) Group A Ca(NO3)2.
3.2 Ca2+ Consumption
Figure 3 shows the calcium ion consumption with time for group A in different environments. The calcium ion consumption for the calcium chloride group increased over the first 150 h, whereas that of the calcium acetate group increased during the first 100 h, and that of the calcium nitrate group increased during the first 12 h. The average calcium ion consumption in the SW environment was lower than that in FW. The highest and lowest quantity of calcium ions were consumed by the calcium chloride and calcium nitrate groups, respectively, in both FW and SW.
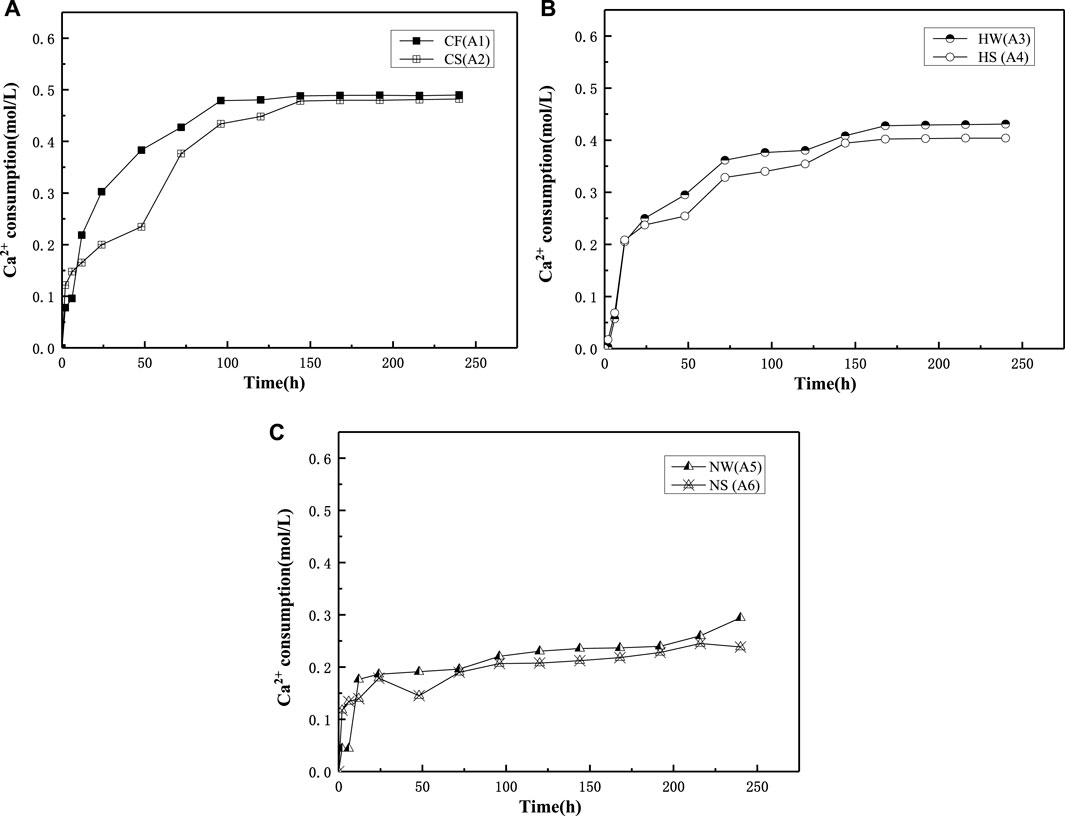
FIGURE 3. Ca2+ consumption in Group A of freshwater and seawater environment. (A) Group A CaCl2; (B) Group A Ca(CH3COO)2; (C) Group A Ca(NO3)2.
3.3 CaCO3 Final Product
The differences between the theoretical and actual CaCO3 content are shown in Tables 2, 3 and in which Table 2 is correspond to the preliminary experiment and Table 3 is correspond to sand column experiment. The theoretical CaCO3 content was estimated from Ca2+ consumption determined using the EDTA method, and actual content was determined from a dry precipitation experiment. In the preliminary experiment, the actual CaCO3 content was lower than the theoretical CaCO3 content, and the actual reduction rate was approximately 20%. The same trend was observed in the sand column experiments as in the preliminary experiment, and the actual reduction rate was approximately 20–50%. Comparing Tables 2, 3 shows that the final production of calcium carbonate in the seawater environment was slightly lower than in freshwater. Thus, the seawater environment had little influence on the calcium chloride group.

TABLE 2. Relationships between theoretical mass value of calcium carbonate product and actual mass value of aqueous solution experiment.

TABLE 3. Relationships between theoretical mass value of calcium carbonate product and actual mass value of cementation solution experiment.
3.4 UCS and TSS Values
In Figure 4, the UCS of the samples is higher in the freshwater environment than in the seawater environment. In Figure 4A (CaCl2 group), the UCS is approximately 7.3 MPa for sample B1 is 39.7% higher than that of sample B2. In Figure 4B (Ca(CH3COO)2 group), the UCS of approximately 5.5 MPa for sample B3 is 30.9% higher than that of sample B4. In Figure 4C (Ca(NO3)2 group), the UCS of approximately 5 MPa for sample B5 is 39.7% higher than that of sample B6.
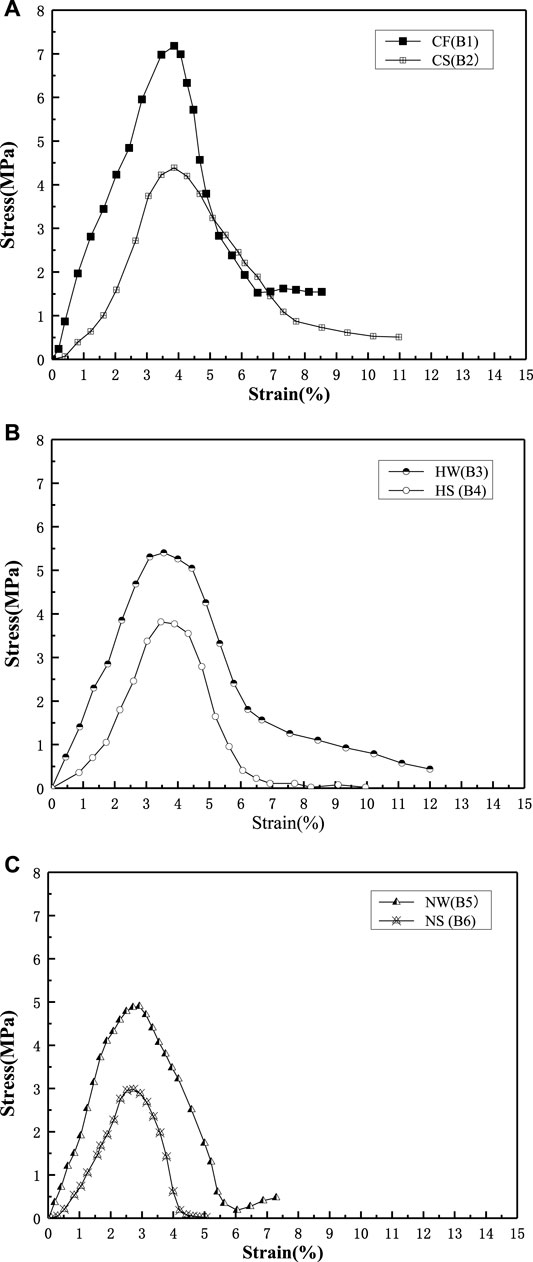
FIGURE 4. Unconfined compression strength (UCS) in Group B of freshwater and seawater environment. (A) Group B CaCl2; (B) Group B Ca(CH3COO)2; (C) Group B Ca(NO3)2.
The trends in the TSS and UCS values are consistent. Therefore, the TSS of the samples is higher in the FW environment than in SW. In Figure 5, the TSS of approximately 3.03 MPa for sample C1 is 34.6% higher than that of sample C2. The TSS of approximately 2.2 MPa for sample C3 is 43.2% higher than that of sample C4. The TSS of approximately 1.85 MPa for sample C5 is 29.7% higher than that of sample C6. Seawater has the strongest effect on Ca(CH3COO)2, followed by CaCl2, and Ca(NO3)2.
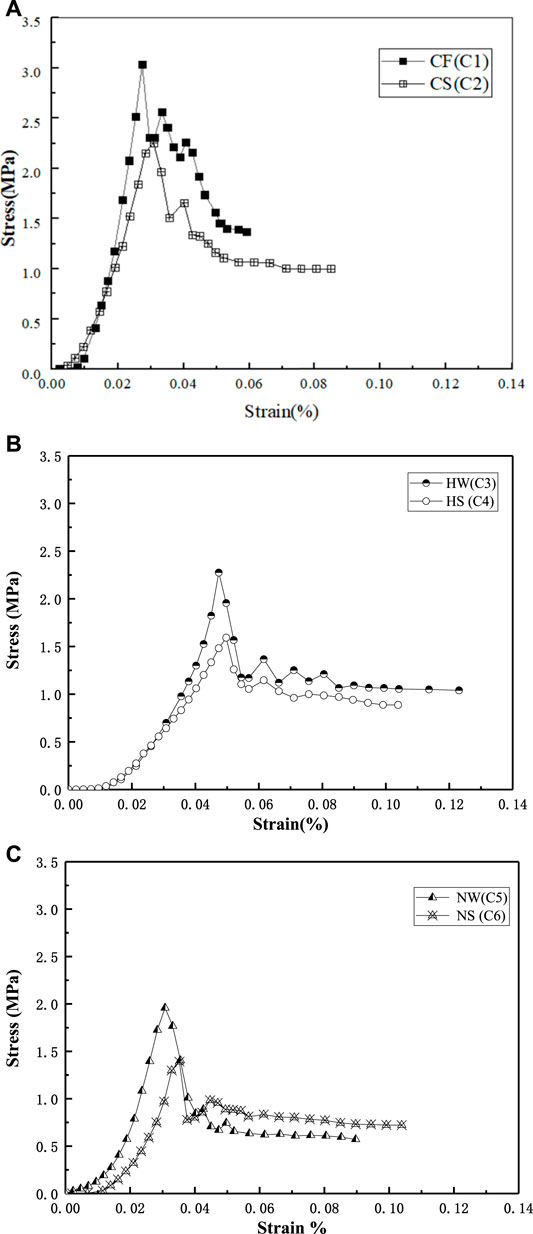
FIGURE 5. Tensile splitting strength (TSS) in Group C of freshwater and seawater environment. (A) Group C CaCl2; (B) Ca(CH3COO)2; (C) Ca(NO3)2.
3.5 Relationship Between UCS and TSS
The relationship between the uniaxial compressive strength and the tensile splitting strength is plotted in Figure 6.
The ratio of the TSS value to the UCS value (the tensile-compressive ratio) is approximately 1:2.4, that is, the tensile strength is approximately 40% of the compressive strength. The approximate linearity of this ratio shows that the tension-compression ratio has little relation to the calcium source and the reinforcement environment.
3.6 XRD
Salinity is an important index for distinguishing seawater from freshwater. The average salinity of the seawater was 34.15‰, and the trace elements in seawater affected the calcium carbonate crystals. In Figure 7A (CaCl2 group), the calcium carbonate crystal in A1 (FW) is calcite, whereas the A2 (SW) crystal is a mixture of calcite and aragonite. In seawater, partial calcite induced by microorganisms was transformed into aragonite. In Figure 7B (Ca(CH3COO)2 group), the A3 (FW) crystal is a mixture of calcite and vaterite, whereas the A4(SW) crystal is a mixture of aragonite and vaterite. In Figure 7C (Ca(NO3)2 group), the A5 (FW) is calcite, whereas the A6 (SW) crystal is aragonite. Thus, the calcite crystals were completely transformed into aragonite in seawater.
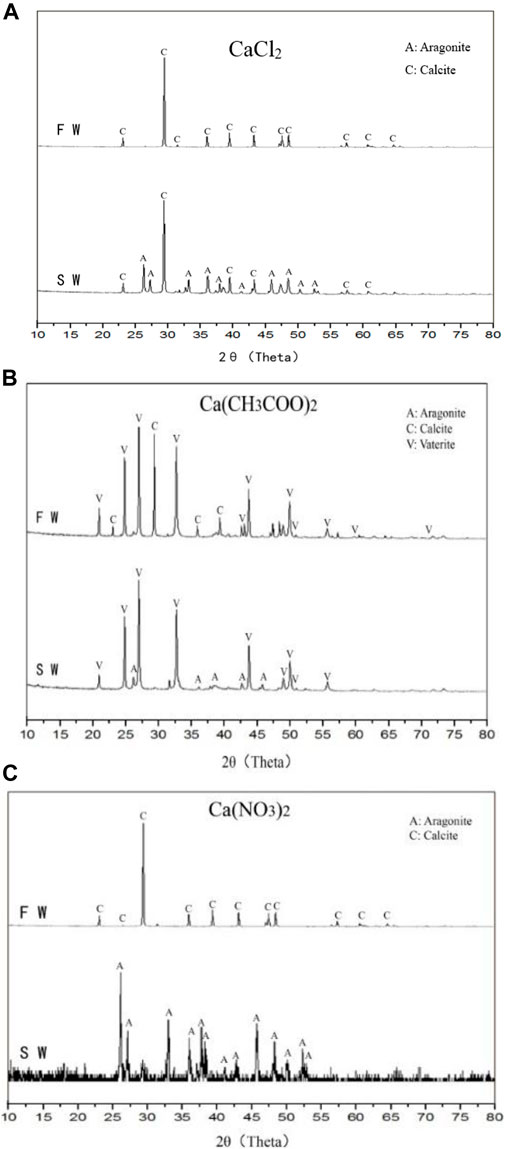
FIGURE 7. X-ray diffraction (XRD) images of calcium carbonate crystals from different samples: (A) CaCl2; (B) Ca(CH3COO)2; (C) Ca(NO3)2.
3.7 SEM
Figure 8 shows the morphology of calcium carbonate obtained in the preliminary experiment (Group A). The following morphologies can be observed in the figure: Figure 8A: blocky and flaky calcite are combined into aggregates; Figure 8B: blocky calcite and columnar aragonite are closely agglomerated; Figure 8C: hexagonal vaterite and a small quantity of columnar aragonite are slightly clustered; Figure 8D: acicular (columnar) aragonite is scattered along with a small quantity of spherical vaterite; Figure 8E: agglomerates of blocky calcite and Figure 8F: scattered local clusters of long columnar and acicular aragonite.
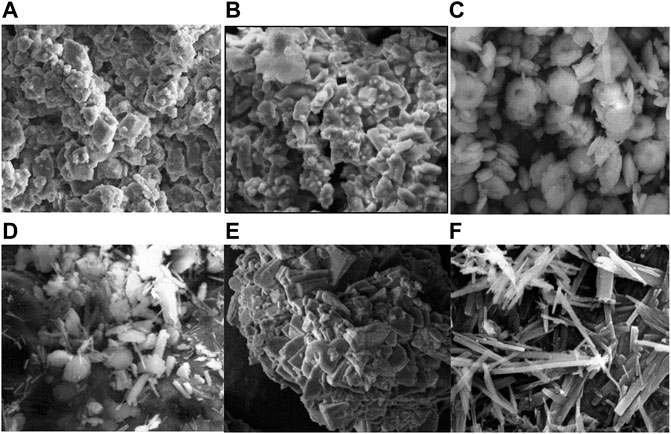
FIGURE 8. Scanning electron microscopy (SEM) images of carbonate precipitation from group A. (A) CaCl2 (FW); (B) CaCl2 (SW); (C) Ca(CH3COO)2 (FW); (D) Ca(CH3COO)2 (SW); (E) Ca(NO3)2 (FW); (F) Ca(NO3)2 (SW).
Figure 9 shows the morphology of calcium carbonate obtained in the column experiments (Group B). The crystals in group B were mainly calcite and aragonite, with a small quantity of vaterite. The morphologies were as follows: Figure 9A: calcite; Figure 9B: aragonite and a small quantity of calcite; Figure 9C: aragonite and calcite; Figure 9D: aragonite and a small quantity of vaterite; Figure 9E: calcite and Figure 9F: aragonite.
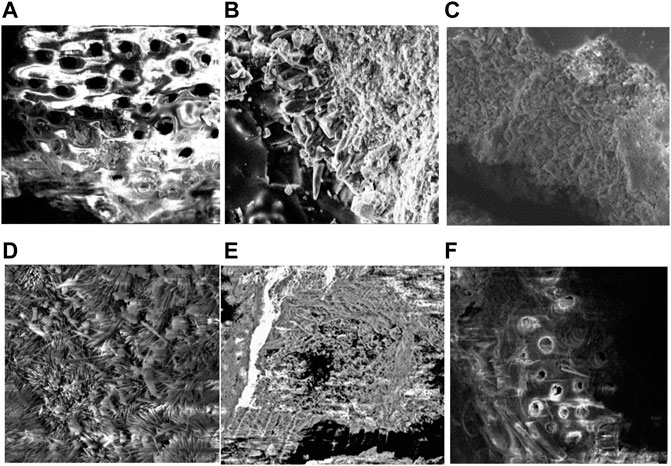
FIGURE 9. Scanning electron microscopy (SEM) images of carbonate precipitation connected with coral sand from group B. (A) CaCl2 (FW); (B) CaCl2 (SW); (C) Ca(CH3COO)2 (FW); (D) Ca(CH3COO)2 (SW); (E) Ca(NO3)2 (FW); (F) Ca(NO3)2 (SW).
4 Discussion
During the biochemical reaction, urea was hydrolyzed to ammonia and carbon dioxide that were converted to ammonium and carbonate ions in the alkaline environment. Ca2+ in aqueous solution combined with CO32− and was adsorbed on the surface of sand particles to function as an intergranular cement [38].
4.1 Analysis of CaCO3 Precipitates
The formation of aragonite was promoted by the magnesium ions in SW by two main mechanisms. First, as the magnesium ions had a smaller particle size and a higher hydration ability than the calcium ions [39], the magnesium ions could be considered as impurities that inhibited the growth of the calcite crystal [40]. Second, at a high magnesium ion content in SW, magnesium ions entered the inner calcite lattice and existed as magnesium-containing calcium carbonate. However, magnesium ions could not enter the inner lattice of aragonite [41].
As amorphous calcium carbonate (ACC) was transformed into calcite under the continuous action of magnesium ions, ACC crystals gradually dissolved and self-assembled into linear ordered nanoparticles. The number of spherical particles decreased with time and gradually turned into acicular aragonite [40]. These particles eventually formed a substance at steady state. The aragonite content increased with the magnesium ion concentration, and the crystal transformed from columnar to acicular. The formation of a calcite crystal was strongly inhibited by the presence of magnesium ions, especially at magnesium ion concentrations above 0.5 mol/L [42]. In addition, acicular aragonite was induced by trace sulfate ions.
It was confirmed that when Ca(CH3COO)2 was the calcium source, calcium carbonate was mainly produced as acicular aragonite. When CaCl2 and Ca(NO3)2 were the calcium sources, calcium carbonate was mainly produced as massive calcite.
4.2 Analysis of Bacterial Metabolic Process
It is mainly considered in seawater environment that the effects of ions (sodium, magnesium, calcium, chloride, sulfate, bromide, and carbonate ions) on bacterial metabolism. In 1957, Danish scientists first discovered that ATPase was the only active when coexisting with magnesium, sodium and potassium ions. ATP was hydrolyzed into ADP and phosphoric acid (Pi), and energy was released autonomously and efficiently transport K+ into the cell and Na+ outside of the cell. The same calcium source and urea were used in the two trials (FW and SW) performed in this study. Thus, the same carbonate ions were obtained from bacterial hydrolysis of urea. The carbon dioxide produced by microbial respiration dissolved in water to form carbonate ions. In the presence of Na+ and other ions in SW, bacterial respiration weakens to maintain vital characteristics and cell electrolyte balance [43]. Therefore, a relatively small quantity of energy was used in the bacterial metabolism to produce CO2 in SW and a small quantity of calcium carbonate was precipitated compared with FW.
5 Conclusion
The objective of this paper was to study the influence of a seawater environment and different calcium sources on strengthening coral sand. The following conclusions are drawn.
The results showed that the actual production of calcium carbonate is 20% lower than the theoretical value. Calcium carbonate production was lower in the seawater environment than in the freshwater environment. The theoretical calcium carbonate production in the calcium chloride group was 2% less in seawater than in freshwater. The corresponding decrease for the calcium acetate and calcium nitrate groups was 7 and 20%, respectively. It can be concluded that the seawater environment had a slight influence on the calcium chloride group. Thus, calcium chloride is an optimal calcium source for reinforcement.
The results of tests on three different calcium sources (calcium chloride, calcium acetate, and calcium nitrate) showed that the seawater environment adversely affected the UCS and TSS of enhanced coral sand columns compared with a freshwater environment. The results of MICP experiments on aqueous solutions showed that the pH and calcium ion consumption were lower in SW than in FW for the same calcium source. The UCS and TSS were higher in the freshwater environment than in the seawater environment. The UCS and TSS values were approximately 30–45% higher in the freshwater environment than in seawater. Nevertheless, the enhanced coral sand column exhibited high strength in a seawater environment. Therefore, MICP technology can be used to solidify coral sand foundations in seawater environments.
Data Availability Statement
The original contributions presented in the study are included in the article/Supplementary material, further inquiries can be directed to the corresponding author.
Author Contributions
TC: Writing—original draft, review and editing. DD: Methodology, Investigation, data curation, Writing—original draft, review and editing. YT: Writing—original draft, review and editing. JP: Conceptualization, Methodology, Supervision, Project administration, Funding acquisition.
Funding
The study presented in this article was substantially supported by National Natural Science Foundation of China (Nos. 51578214 and 51608169). The support is gratefully acknowledged. The fund moderator is JP.
Conflict of Interest
The authors declare that the research was conducted in the absence of any commercial or financial relationships that could be construed as a potential conflict of interest.
Publisher’s Note
All claims expressed in this article are solely those of the authors and do not necessarily represent those of their affiliated organizations, or those of the publisher, the editors and the reviewers. Any product that may be evaluated in this article, orclaim that may be made by its manufacturer, is not guaranteed or endorsed by the publisher.
References
1. Dejong JT, Mortensen BM, Martinez BC, Nelson DC. Bio-mediated Soil Improvement. Ecol Eng (2010) 36(2):197–210. doi:10.1016/j.ecoleng.2008.12.029
2. Tan Y, Xie X, Wu S, Wu T. Microbially Induced CaCO3 Precipitation: Hydraulic Response and Micro-scale Mechanism in Porous media. ScienceAsia (2017) 43(1):1. doi:10.2306/scienceasia1513-1874.2017.43.001
3. Liu B, Zhu C, Tang C-S, Xie Y-H, Yin L-Y, Cheng Q, et al. Bio-remediation of Desiccation Cracking in Clayey Soils through Microbially Induced Calcite Precipitation (Micp). Eng Geology (2020) 264:105389. doi:10.1016/j.enggeo.2019.105389
4. Xiao Y, He X, Wu W, Stuedlein AW, Evans TM, Chu J, et al. Kinetic Biomineralization through Microfluidic Chip Tests. Acta Geotech. (2021) 16(10):3229–37. doi:10.1007/s11440-021-01205-w
5. Cheng L, Cord-Ruwisch R, Shahin MA. Cementation of Sand Soil by Microbially Induced Calcite Precipitation at Various Degrees of Saturation. Can Geotech J (2013) 50(1):81–90. doi:10.1139/cgj-2012-0023
6. Achal V, Mukherjee A. A Review of Microbial Precipitation for Sustainable Construction. Construction Building Mater (2015) 93:1224–35. doi:10.1016/j.conbuildmat.2015.04.051
7. Su Jf., Zhang H, Huang Tl., Hu Xf., Chen Cl., Liu Jr. The Performance and Mechanism of Simultaneous Removal of Fluoride, Calcium, and Nitrate by Calcium Precipitating Strain Acinetobacter Sp. H12. Ecotoxicology Environ Saf (2020) 187:109855. doi:10.1016/j.ecoenv.2019.109855
8. Gowthaman S, Iki T, Nakashima K, Ebina K, Kawasaki S. Feasibility Study for Slope Soil Stabilization by Microbial Induced Carbonate Precipitation (Micp) Using Indigenous Bacteria Isolated from Cold Subarctic Region. SN Appl Sci (2019) 1(11):1480. doi:10.1007/s42452-019-1508-y
9. Peng J, Liu Z. Influence of Temperature on Microbially Induced Calcium Carbonate Precipitation for Soil Treatment. PLoS ONE (2019) 14(6):e0218396. doi:10.1371/journal.pone.0218396
10. Dupraz S, Ménez B, Gouze P, Leprovost R, Bénézeth P, Pokrovsky OS, et al. Experimental Approach of Co2 Biomineralization in Deep saline Aquifers. Chem Geology (2009) 265(1):54–62. doi:10.1016/j.chemgeo.2008.12.012
11. Oualha M, Bibi S, Sulaiman M, Zouari N. Microbially Induced Calcite Precipitation in Calcareous Soils by Endogenous bacillus Cereus, at High Ph and Harsh Weather. J Environ Manage (2020) 257:109965. 109965. doi:10.1016/j.jenvman.2019.109965
12. Wen K, Li Y, Liu S, Bu C, Li L. Evaluation of MICP Treatment through EC and pH Tests in Urea Hydrolysis Process. Environ Geotechnics (2021) 8:274–81. doi:10.1680/jenge.17.00108
13. Mortensen BM, Haber MJ, DeJong JT, Caslake LF, Nelson DC. Effects of Environmental Factors on Microbial Induced Calcium Carbonate Precipitation. J Appl Microbiol (2011) 111(2):338–49. doi:10.1111/j.1365-2672.2011.05065.x
14. Gorospe CM, Han S-H, Kim S-G, Park J-Y, Kang C-H, Jeong J-H, et al. Effects of Different Calcium Salts on Calcium Carbonate crystal Formation by Sporosarcina Pasteurii Kctc 3558. Biotechnol Bioproc E (2013) 18(5):903–8. doi:10.1007/s12257-013-0030-0
15. Achal V, Pan X, Zhang D. Remediation of Copper-Contaminated Soil by Kocuria Flava Cr1, Based on Microbially Induced Calcite Precipitation. Ecol Eng (2011) 37(10):1601–5. doi:10.1016/j.ecoleng.2011.06.008
16. Achal V, Pan X. Influence of Calcium Sources on Microbially Induced Calcium Carbonate Precipitation by bacillus Sp. Cr2. Appl Biochem Biotechnol (2014) 173:307–17. doi:10.1007/s12010-014-0842-1
17. Kumari D, Qian X-Y, Pan X, Achal V, Li Q, Gadd GM. Microbially-induced Carbonate Precipitation for Immobilization of Toxic Metals. Adv Appl Microbiol (2016) 94:79–108. doi:10.1016/bs.aambs.2015.12.002
18. He J, Chu J, Liu H-L, Gao Y-F. Microbial Soil Desaturation for the Mitigation of Earthquake Liquefaction. JGS Spec Publ (2016) 2(21):784–7. doi:10.3208/jgssp.SEA-05
19. Liu H, Peng X, Yang X, Wang J, Chen Y, Chu J. Dynamic Behaviors of Micp-Treated Calcareous Sand in Cyclic Tests. Chin J Geotechnical Eng (2018).
20. De Muynck W, De Belie N, Verstraete W. Microbial Carbonate Precipitation in Construction Materials: A Review. Ecol Eng (2010) 36(2):118–36. doi:10.1016/j.ecoleng.2009.02.006
21. Xiao P, Liu H, Xiao Y, Stuedlein AW, Evans TM. Liquefaction Resistance of Bio-Cemented Calcareous Sand. Soil Dyn Earthquake Eng (2018) 107:9–19. doi:10.1016/j.soildyn.2018.01.008
22. Manzur T, Shams Huq R, Hasan Efaz I, Afroz S, Rahman F, Hossain K. Performance Enhancement of brick Aggregate concrete Using Microbiologically Induced Calcite Precipitation. Case Stud Construction Mater (2019) 11:e00248. doi:10.1016/j.cscm.2019.e00248
23. Stabnikov V, Chu J, Myo AN, Ivanov V. Immobilization of Sand Dust and Associated Pollutants Using Bioaggregation. Water Air Soil Pollut (2013) 224(9):1–9. doi:10.1007/s11270-013-1631-0
24. Gomez . Field Scale Bio-Cementation for the Improvement of Loose Sands. Dissertations & Theses - Gradworks (2013).
25. Liu C, Ren W. Preliminary Research on Physical and Mechanical Properties of Calcareous Sand. ROCK SOIL MECHANICS (1998) 19(1). doi:10.16285/j.rsm.1998.01.006
26. Liu C, Yang Z, Ren W. The Present Condition and Development in Studies of Mechanical Properties of Calcareous Soils. Rock Soil Mech (1995) 16(4). doi:10.16285/j.rsm.1995.04.010
27. Zhu C, Chen H, Meng Q, Meng R. Microscopic Characterization of Intra-pore Structures of Calcareous Sands. Rock Soil Mech (2014) 5(7):6. doi:10.16285/j.rsm.2014.07.005
28. Lu H, Wang R, Kong L. Preliminary Mesoscopic Analysis on Factors of Breakage in Calcareous Soil. Chin J Rock Mech Eng (2001) 20(Suppl. 1):890–2.
29. Qian C, Wang A, Wang X. Advances of Soil Improvement with Bio-Grouting. Rock Soil Mech (2015) 36(6):1537–48. doi:10.16285/j.rsm.2015.06.003
30. Peng J, Liu H, Chen Y. Mechanism of Foundation Strengthening by Vacuum-Surcharge Preloading Method. Journal of Hohai University Natural Sciences (2003). doi:10.1007/s11769-003-0044-1
31. Cheng X, Liu X, Shu L. Investigation of Cross-Sea Bridge for Atoll and Engineering Significance. Port & Waterway Engineering (2018).
32. Dejong JT, Fritzges MB, Nüsslein K. Microbially Induced Cementation to Control Sand Response to Undrained Shear. J Geotech Geoenviron Eng (2006) 132132(11):138111–1392. doi:10.1061/(ASCE)1090-024110.1061/(asce)1090-0241(2006)132:11(1381)
33. Silva-Castro GA, Uad I, Gonzalez-Martinez A, Rivadeneyra A, Gonzalez-Lopez J, Rivadeneyra MA. Bioprecipitation of Calcium Carbonate Crystals by Bacteria Isolated from saline Environments Grown in Culture media Amended with Seawater and Real Brine. Biomed Res Int (2015) 2015:1–12. doi:10.1155/2015/816102
34. Silva-Castro GA, Uad I, Rivadeneyra A, Vilchez JI, Martin-Ramos D, González-López J, et al. Carbonate Precipitation of Bacterial Strains Isolated from Sediments and Seawater: Formation Mechanisms. Geomicrobiology J (2013) 30(9):840–50. doi:10.1080/01490451.2013.777492
35. Gat D, Tsesarsky M, Shamir D, Ronen Z. Accelerated Microbial-Induced CaCO3 Precipitation in a Defined Coculture of Ureolytic and Non-ureolytic Bacteria. Biogeosciences (2013) 11(11):2561–9. doi:10.5194/bg-11-2561-2014
36. Wei S, Cui H, Jiang Z, Liu H, He H, Fang N. Biomineralization Processes of Calcite Induced by Bacteria Isolated from marine Sediments. Braz J Microbiol (2015) 46(2):455–64. doi:10.1590/S1517-838246220140533
37. Guo X, Cheng X, Zhang Y. Influences of Calcium Sources on Microbially Induced Carbonate Precipitation in Porous mediaMaterials Research Innovations (2014).
38. Davis KJ, The Role of Mg2+ as an Impurity in Calcite Growth. Science (2000) 290(5494):1134–7. doi:10.1126/science.290.5494.1134
39. Brečević , Noethig L, Kralj V, Damir P, Stanko . Effect of Divalent Cations on the Formation and Structure of Calcium Carbonate Polymorphs. J.chem.soc.faraday Trans (1996). doi:10.1039/FT9969201017
41. Liu Z, Yan M, Zhang L, Li H. Study on the Effect of Magnesium Ion on the crystal Form of Calcium Carbonate. Tech Water Treat (2011) 37(10):60–2. doi:10.1002/clc.20818
42. Jiang L, Lin D, Zhang Z. Study on Mechanism during Synthesis of Nano-Meter Calcium Carbonate. China Powder Science and Technology (2002).
Keywords: MICP, coral sand, freshwater, seawater, calcium source
Citation: Peng J, Cao T, He J, Dai D and Tian Y (2022) Improvement of Coral Sand With MICP Using Various Calcium Sources in Sea Water Environment. Front. Phys. 10:825409. doi: 10.3389/fphy.2022.825409
Received: 30 November 2021; Accepted: 24 January 2022;
Published: 14 March 2022.
Edited by:
Wanqing Shen, Université de Lille, FranceCopyright © 2022 Peng, Cao, He, Dai and Tian. This is an open-access article distributed under the terms of the Creative Commons Attribution License (CC BY). The use, distribution or reproduction in other forums is permitted, provided the original author(s) and the copyright owner(s) are credited and that the original publication in this journal is cited, in accordance with accepted academic practice. No use, distribution or reproduction is permitted which does not comply with these terms.
*Correspondence: Jie Peng, peng-jie@hhu.edu.cn