- 1Dipartimento di Fisica, Università di Cagliari, Monserrato, Italy
- 2Unité Mixte de Physique, CNRS, Thales, Université Paris-Saclay, Palaiseau, France
- 3Universite Grenoble Alpes, CNRS, Grenoble INP, Institut Néel, Grenoble, France
The discovery of superconductivity in infinite-layer nickelates has attracted much attention due to their association to the high-Tc cuprates. Cuprate superconductivity was first demonstrated in bulk samples and subsequently in thin films. In the nickelates, however, the situation has been reversed: although surging as a bulk phenomenon, nickelate superconductivity has only been reported in thin films so far. At the same time, the specifics of infinite-layer nickelates yield distinct interface and surface effects that determine their bulk vs thin-film behavior. In this paper, we provide an overview on these important aspects.
1 Introduction
The infinite-layer nickelates RNiO2 (R = rare-earth element) have long been discussed as potential cuprate-like high-Tc superconductors [1–4]. This idea can now be scrutinized experimentally after the discovery of superconductivity in hole-doped NdNiO2 [5] and its subsequent verification in hole-doped PrNiO2 and LaNiO2 as well [6–13]. To date, however, this breakthrough remains limited to thin films (see e.g. [14–16]). This circumstance is apparently related to the thermodynamic fragility of these special phases, in which the interesting electronic properties require an unfavorably low valence of the nickel atom. Thus, the simultaneous control of both sample quality and doping necessary to promote superconductivity in these nickelates turns out to be a real experimental challenge. In this respect, the thin-film approach has proven its advantages. At the same time, superconductivity has been reported for thicknesses as large as 17 nm (i.e., ∼ 50 unit cells), with relatively large critical currents (≳ 200 kA/cm2), and also for thin films on different substrates. Consequently, there is consensus in that nickelate superconductivity is a genuine bulk phenomenon.
The verification and further investigation of nickelate superconductivity in actual bulk samples (ideally single-crystals) has therefore emerged as an important goal in the field. In parallel, the efforts made along the thin-film route have very recently enabled the observation of nickelate superconductivity in a quintuple layer system that is hardly realizable in its bulk form [17]. Thus, the thin-film approach continues to be the favoured option to gain further insight about nickelate superconductivity. At the same time, the specific effects that may be at play in thin-film vs bulk samples need to be better understood. In the following we provide an overview of the current research along this line.
2 Epitaxial-Growth Aspects
The synthesis of rare-earth infinite-layer nickelate thin films requires the epitaxial growth of the perovskite precursors RNiO3 in the first place. These perovskite nickelates have been grown by various techniques. The most popular is pulsed laser deposition (PLD) [18–21], but sputtering [22, 23] and molecular beam epitaxy [24] have also been used. At this stage, the main difficulty lies in the stabilization of the unfavorable high 3+ valence of Ni (instead of its most preferred 2+), reproducibility and off-stoichiometry issues have been reported in the literature [25]. This difficulty increases for Ca- or Sr-doped films, as required to obtain the subsequent infinite-layer phases in the appropriate regime of Ni 3d-electron filling for superconductivity (i.e.,
Even if single-phase perovskite thin films are obtained, it is important to keep in mind that the quality of the samples may be limited by the presence of additional atoms or defects. At the interfaces, in particular, epitaxial strain may favor the presence of oxygen or even A-site vacancies (see e.g., [29, 30]). What is more, achieving a single termination is difficult in many substrates and different interfacial configurations are generally possible in these systems (see e.g., [31, 32]). Consequently, the Ni atom may be embedded in multiple interfacial environments already at this stage.
After the precursor perovskite phase has been obtained, the samples are reduced by thermal annealing in a vacuum-sealed tube containing a small amount of a highly reducing agent (CaH2). The goal is to selectively remove one-third of oxygens (i.e., one full plane of apical oxygens) and thereby stabilize the oxygen poorer infinite-layer phase. The literature diverges regarding the exact conditions for this process, but it typically has to be performed between 200 and 360°C for several hours [6, 7, 10, 27, 28]. In the ideal case, X-ray diffraction then reveals the complete transformation of the perovskite phase into the infinite-layer phase, with a characteristic shift of the (002) peak position to higher angles (∼54°) signalling the contraction of the out-of-plane lattice constant, an intense (001) peak (its absence or reduced intensity is again the signature of spurious phases) and Laue fringes, attesting of the good structural coherence of the infinite-layer phase in the growth direction [9, 27, 28].
3 Epitaxial Strain
The most obvious additional ingredient that appears in thin films is the epitaxial—or biaxial—strain associated to the lattice mismatch between the nickelate and the substrate. For instance, the lattice parameter of the common substrate SrTiO3 is 3.905 Å while the lattice parameters of bulk LaNiO2 have been reported to be a = 3.96 Å and c = 3.37 Å [1]. Thus, for c-axis oriented thin films of LaNiO2 grown on SrTiO3(001) the epitaxial strain, which is defined as (asubstrate − a)/a, will be −1.4%. In general, this reduction of the a parameter is accompanied with an increase in c since the system is free to relax the stress in that direction. That is, imposing an in-plane compression at the interface results in out-of-plane tensile strain. Conversely, in-plane tensile strain—due to a different substrate for example—can be expected to produce an out-of-plane compression.
By means of these changes in the cell parameters, epitaxial strain can further modify the key features of the corresponding electronic structure (see e.g., [33]). The “cuprateness” of this structure, in particular, is generally defined from the nature of the states at the Fermi level and the so-called charge-transfer energy (see e.g., [3, 34–37]). In this respect, in-plane compressive strain increases both the bandwidth of the main Ni-3
If the film relaxes, epitaxial strain will typically decrease with the distance to the interface and the lattice parameters should eventually recover their bulk values as the thickness of the film increases. This overall relaxation should occur following a power-law behavior—rather than an exponential one—due to the long-range nature of the strain field. This has been quantified for (Nd,Sr)NiO2/SrTiO3 in [11]. Specifically, the c lattice parameter is found to decrease from 3.42 Å for their 5.1 nm thick sample to 3.36 Å whenever the thickness is larger than 7 nm. At the same time, the superconducting Tc is found to correlate with such a decrease in the strain as it displays an increase from 6 to 13 K. On the other hand, the Tc has been reported to increase in (Pr,Sr)NiO2 grown on
The apparent contradiction between these observations may be resolved due to different factors. Two of them may be just stoichiometry and sample quality. The R:Ni flux ratio used during the growth of the perovskite films, for example, has been reported to be an important factor for their subsequent reduction into the infinite-layer phase and hence for the observation superconductivity [40]. Beyond that, samples synthesized in nominally similar conditions can easily show substantial variations in the superconducting Tc and even stay non-superconducting [5]. In this respect, the recent report of superconductivity in (La,Sr)NiO2 emphasizes the importance of sufficiently low disorder and high crystallinity [9, 10]. Consequently, the quantification of the Tc as a function of the structure may be taken with a grain of salt (in the sense that the actual error bars may be quite large).
4 Chemical Reconstructions
4.1. Topotatic Hydrogen
Another factor that convolutes with the above is the composition itself. As mentioned above, infinite-layer nickelates are synthesized by topotactic reduction of perovskite precursors using reducing agents such as CaH2. This process, however, may result in the formation of oxide-hydrides RNiO2H. This possibility has been argued to correlate with both the R element and epitaxial strain [38, 41, 42]. Specifically, while the parent phases are prone to topotactically incorporate H, both R-element substitution and compressive epitaxial strain can limit this possibility. The latter is consistent with the transformation of the infinite-layer NdNiO2 phase into a fluorite-defect structure NdNiOxHy as a function of the distance to the interface with (001) SrTiO3 reported in [43].
4.2 Apical Oxygens
Beyond that, even without insertion of topotactic hydrogen, the reduction process can be different at the interface and further away from it and may result, in particular, in the presence of apical oxygens. This question was first addressed by means of DFT calculations in [44, 45]. Both these works predicted the presence of these interfacial apical oxygens, which have recently been confirmed in an ad hoc experimental study supplemented with DMFT calculations [46].
The presence of apical oxygens at the interfaces can be seen as a sort of “chemical” reconstruction of the nickelate that locally introduces hole doping. This is illustrated in Figure 1. Without reconstruction, the characteristic band structure of the bulk would be essentially preserved at the interface. Accordingly, the Ni atom would display the same oxidation state everywhere. However, if the apical oxygen remains at the interface, then the local band structure changes towards the RNiO3 —or rather RNiO2.5— case. This implies that the Ni oxidation state locally approaches +2 rather than +1. This is a very interesting possibility since it may emulate the hole doping obtained by means of the R → Sr or Ca substitution, necessary to further promote superconductivity. However, the additional charge introduced in this way may be distributed over a distance in the film. Thus, in the ultrathin limit, the effective degree of doping would eventually be controlled by the thickness of the film. In fact, a gradual increase of the Ni oxidation state as the thickness of the superconducting films decreases has been inferred from XAS data in [11]. These carriers, however, have been argued to be self-trapped at the interfaces due to Hund’s coupling according to DMFT calculations [46]. We note that this may be reminiscent of the metal-insulator transition that takes place in the perovskite counterparts. At the same time, carriers from B-site atom of the substrate—Ti in SrTiO3 vs Ga in LaGaO3 for example—also participate in the overall process so that the self-trapping may be surface specific.
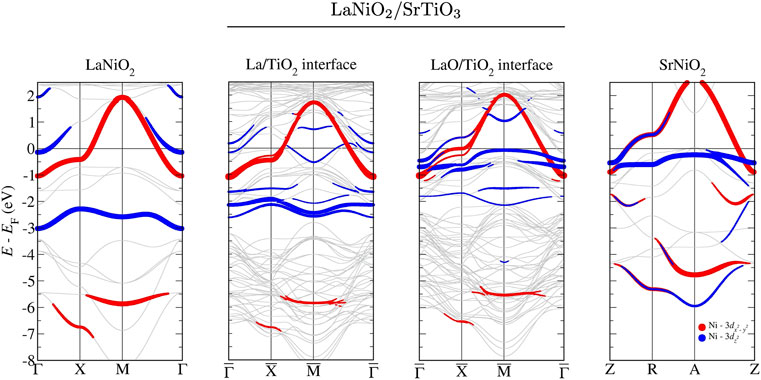
FIGURE 1. Electronic band structure of the LaNiO2/SrTiO3 heterostructure for different interfacial configurations compared to bulk LaNiO2 and SrNiO2 with the same crystal structure and lattice parameters (the Γ-X-M-Γ and Z-R-A-Z paths fold to
4.3 Additional Thin Film/Substrate Terminations
The situation may be even more complex since, depending on the actual synthesis conditions, additional chemical reconstructions have been shown to be possible at the interface [44]. In particular, the most likely RO/TiO2 configuration may be replaced by the NiO2/SrO one. That is, the termination may be different. In that case, there would be an extra local doping associated to the interfacial Sr, superimposed to the one from the apical oxygen. In fact, the interfacial cell would be La0.5Sr0.5NiO2.5 so that the oxidation state of the Ni atom would increase to +2.5. This overdoping again may be distributed over a distance so that the actual occupation of the Ni-3d states may be determined by the thickness of the film. In the ultrathin limit, in particular, the corresponding electronic structure has been shown to undergo drastic changes (see Figure 2). Specifically, the initial self-doping effect provided by the R-5d states in the bulk will be completely depleted by the local SrO overdoping at the interface. At the same time, the intefacial Ni-3
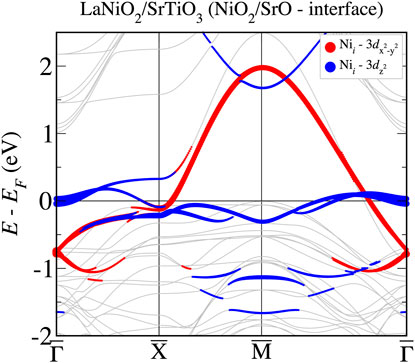
FIGURE 2. Electronic band structure of the LaNiO2/SrTiO3 heterostructure for the NiO2/SrO interfacial configuration (the colors highlight the main contributions of the interfacial Ni-3d states near the Fermi level and a 3/3 superlattice is considered). The effective interfacial doping for this configuration depletes the self-doping from the R-5d states characteristic of the bulk and places the Ni-3
4.4 Surface
The above changes with respect to the bulk may also happen in thin films with asymmetric boundaries where the effective built-in electric field that appears due to the corresponding polar discontinuities play a role. Specifically, the screening of this field implies a charge transfer that adds to the aforementioned effects. In the case of asymmetric boundaries, polar layers can be formed at both the surface and the interface [47, 48]. These layers display antiparallel NiO2 displacements, but otherwise are decoupled. Further, the aforementioned depletion of the R-5d states can also be obtained at the surface while a two-dimensional electron gas extending over several layers can be formed at the interface [45, 47]. Besides, the combined effect of magnetism—G-type antiferromagnetic order—and correlations at the DFT + U level has been found to enhance the itineracy of the Ni-3
The focus has been put on the surface properties in [49] taking into account the possibility of apical oxygens anticipated in [44, 45]. Thus, it has been confirmed that different terminations yield different electronic structures also at the surface (see Figure 1). This is further shown to modify qualitatively the corresponding Fermi surface. As a result of this modification, it is argued that the d-wave superconducting gap expected for the bulk may transform into a s±-wave one at the NiO2-terminated surface. This provides a rather natural explanation to the local changes in the tunneling spectrum observed in [13]. Further, it suggests that a surface s + id-wave state may also be realized under the appropriate conditions.
5 Outlook
The thin-film approach has proven its advantages to overcome the thermodynamic fragility of the infinite-layer nickelates, thereby demonstrating the emergence of unconventional superconductivity in these systems. Besides, this approach offers additional degrees of flexibility to further investigating this phenomenon.
Epitaxial strain can be used not only to fine tune the electronic structure of these nickelates and hence their “cuprateness”, but also the underlying crystal structure itself. Beyond that, the presence of apical oxygens at the interfaces/surfaces enables a local control of the effective doping without the need of rare-earth-element substitution. This apical-oxygen doping can be further supplemented with an extra contribution from the interfacial terminations themselves. This may be useful to bypass the sample-quality issues associated with doping at the rare-earth site. In fact, the overall local doping obtained in that way may be a particularly effective control parameter in the ultrathin limit.
Another route yet to be explored experimentally is the engineering of the electronic properties via nickelate-based superlattices. This idea was put forward some time ago with the perovskite phases as building blocks [50]. This concept can now be supplemented with the possibility reducing these phases, thereby mimicking higher-order layered nickelates. The
Author Contributions
All authors listed have made a substantial, direct, and intellectual contribution to the work and approved it for publication.
Conflict of Interest
The authors declare that the research was conducted in the absence of any commercial or financial relationships that could be construed as a potential conflict of interest.
Publisher’s Note
All claims expressed in this article are solely those of the authors and do not necessarily represent those of their affiliated organizations, or those of the publisher, the editors, and the reviewers. Any product that may be evaluated in this article, or claim that may be made by its manufacturer, is not guaranteed or endorsed by the publisher.
Acknowledgments
LI acknowledges the support and funding from the Île de France region and the European Union’s Horizon 2020 research and innovation programme under the Marie Sklodowska-Curie grant agreement N°21 004 513 (DOPNICKS project). AC was supported by ANR, Grant ANR-18-CE30-0018.
References
1. Hayward MA, Green MA, Rosseinsky MJ, Sloan J Sodium Hydride as a Powerful Reducing Agent for Topotactic Oxide Deintercalation: Synthesis and Characterization of the Nickel(I) Oxide LaNiO2. J Am Chem Soc (1999) 121:8843–54. doi:10.1021/ja991573i
2. Anisimov VI, Bukhvalov D, Rice TM Electronic Structure of Possible Nickelate Analogs to the Cuprates. Phys Rev B (1999) 59:7901–6. doi:10.1103/physrevb.59.7901
3. Lee K-W, Pickett WE Infinite-layerLaNiO2: Ni1+is notCu2+. Phys Rev B (2004) 70:165109. doi:10.1103/physrevb.70.165109
4. Norman MR Entering the Nickel Age of Superconductivity. Physics (2020) 13:85. doi:10.1103/physics.13.85
5. Li D, Lee K, Wang BY, Osada M, Crossley S, Lee HR, et al. Superconductivity in an Infinite-Layer Nickelate. Nature (2019) 572:624–7. doi:10.1038/s41586-019-1496-5
6. Zeng S, Tang CS, Yin X, Li C, Li M, Huang Z, et al. Phase Diagram and Superconducting Dome of Infinite-Layer Nd1−xSrxNiO2 Thin Films. Phys Rev Lett (2020) 125:147003. doi:10.1103/physrevlett.125.147003
7. Osada M, Wang BY, Goodge BH, Lee K, Yoon H, Sakuma K, et al. A Superconducting Praseodymium Nickelate with Infinite Layer Structure. Nano Lett (2020) 20:5735–40. doi:10.1021/acs.nanolett.0c01392
8. Osada M, Wang BY, Lee K, Li D, Hwang HY Phase Diagram of Infinite Layer Praseodymium Nickelate Pr1−xSrxNiO2 Thin Films. Phys Rev Mater (2020) 4:121801. doi:10.1103/physrevmaterials.4.121801
9. Osada M, Wang BY, Goodge BH, Harvey SP, Lee K, Li D, et al. Nickelate Superconductivity without Rare‐Earth Magnetism: (La,Sr)NiO 2. Adv Mater (2021) 33:2104083. doi:10.1002/adma.202104083
13. Gu QQ, Li YY, Wan SY, Li HZ, Guo W, Yang H, et al. Nat Commun (2020) 11:7. doi:10.1038/s41467-020-19908-1
14. Botana AS, Bernardini F, Cano A Nickelate Superconductors: an Ongoing Dialog between Theory and Experiments. Zh Eksp Teor Fiz (2021) 159:711–8. arXiv:2012.02764. doi:10.31857/s0044451021040131
17. Pan GA, Ferenc Segedin D, LaBollita H, Song Q, Nica EM, Goodge BH, et al. arXiv:2109.09726 (2021).
19. Guo Q, Farokhipoor S, Magén C, Rivadulla F, Noheda B Tunable Resistivity Exponents in the Metallic Phase of Epitaxial Nickelates. Nat Commun (2020) 11:2949. arXiv: 1909.06256. doi:10.1038/s41467-020-16740-5
20. Frano A, Benckiser E, Lu Y, Wu M, Castro-Colin M, Reehuis M, et al. Layer Selective Control of the Lattice Structure in Oxide Superlattices. Adv Mater (2014) 26:258–62. doi:10.1002/adma.201303483
21. Liu J, Kargarian M, Kareev M, Gray B, Ryan PJ, Cruz A, et al. Heterointerface Engineered Electronic and Magnetic Phases of NdNiO3 Thin Films. Nat Commun (2013) 4:2714. doi:10.1038/ncomms3714
22. Scherwitzl R, Zubko P, Lezama IG, Ono S, Morpurgo AF, Catalan G, et al. Electric-Field Control of the Metal-Insulator Transition in Ultrathin NdNiO3 Films. Adv Mater (2010) 22:5517–20. doi:10.1002/adma.201003241
23. Mikheev E, Hauser AJ, Himmetoglu B, Moreno NE, Janotti A, Van de Walle CG, et al. Sci Adv (2015) 1:e1500797. doi:10.1126/sciadv.1500797
24. King PDC, Wei HI, Nie YF, Uchida M, Adamo C, Zhu S, et al. Atomic-scale Control of Competing Electronic Phases in Ultrathin LaNiO3. Nat Nanotech (2014) 9:443–7. doi:10.1038/nnano.2014.59
25. Preziosi D, Sander A, Barthélémy A, Bibes M Reproducibility and Off-Stoichiometry Issues in Nickelate Thin Films Grown by Pulsed Laser Deposition. AIP Adv (2017) 7:015210. doi:10.1063/1.4975307
26. Lee K, Goodge BH, Li D, Osada M, Wang BY, Cui Y, et al. Aspects of the Synthesis of Thin Film Superconducting Infinite-Layer Nickelates. APL Mater (2020) 8:041107. doi:10.1063/5.0005103
27. Gao Q, Zhao Y, Zhou X-J, Zhu Z Preparation of Superconducting Thin Films of Infinite-Layer Nickelate Nd0.8Sr0.2NiO2. Chin Phys. Lett. (2021) 38:077401. doi:10.1088/0256-307x/38/7/077401
28. Lee K, Goodge BH, Li D, Osada M, Wang BY, Cui Y, et al. Aspects of the Synthesis of Thin Film Superconducting Infinite-Layer Nickelates. APL Mater (2020) 8:041107. doi:10.1063/5.0005103
29. Aschauer U, Pfenninger R, Selbach SM, Grande T, Spaldin NA Phys Rev B (2013) 88:054111. doi:10.1103/physrevb.88.054111
31. Kumah DP, Malashevich A, Disa AS, Arena DA, Walker FJ, Ismail-Beigi S, et al. Phys Rev Appl (2014) 2:054004. doi:10.1103/physrevapplied.2.054004
32. Li Y, Wrobel F, Yan X, Bhattacharya A, Sun J, Hong H, et al. Interface Creation on a Mixed-Terminated Perovskite Surface. Appl Phys Lett (2021) 118:061601. doi:10.1063/5.0036279
33. Kapeghian J, Botana AS Electronic Structure and Magnetism in Infinite-Layer Nickelates RNiO2 (R=La−Lu). Phys Rev B (2020) 102:205130. doi:10.1103/physrevb.102.205130
35. Olevano V, Bernardini F, Blase X, Cano A Ab Initio many-body GW Correlations in the Electronic Structure of LaNiO2. Phys Rev B (2020) 101:161102. doi:10.1103/physrevb.101.161102
36. Wang Y, Kang C-J, Miao H, Kotliar G Hund's Metal Physics: From SrNiO2 to LaNiO2. Phys Rev B (2020) 102:161118. doi:10.1103/physrevb.102.161118
40. Li Y, Sun W, Yang J, Cai X, Guo W, Gu Z, et al. Front Phys (2021) 9:443. doi:10.3389/fphy.2021.719534
41. Si L, Xiao W, Kaufmann J, Tomczak JM, Lu Y, Zhong Z, et al. Topotactic Hydrogen in Nickelate Superconductors and Akin Infinite-Layer Oxides ABO2. Phys Rev Lett (2020) 124:166402. doi:10.1103/physrevlett.124.166402
43. Onozuka T, Chikamatsu A, Katayama T, Fukumura T, Hasegawa T Formation of Defect-Fluorite Structured NdNiOxHy Epitaxial Thin Films via a Soft Chemical Route from NdNiO3 Precursors. Dalton Trans (2016) 45:12114–8. doi:10.1039/c6dt01737a
44. Bernardini F, Cano A Stability and Electronic Properties of LaNiO2/SrTiO3 Heterostructures. J Phys Mater (2020) 3:03LT01. doi:10.1088/2515-7639/ab9d0f
46. Ortiz RA, Menke H, Misják F, Mantadakis DT, Fürsich K, Schierle E, et al. Superlattice Approach to Doping Infinite-Layer Nickelates. Phys Rev B (2021) 104:165137. doi:10.1103/physrevb.104.165137
47. Zhang Y, Lin L-F, Hu W, Moreo A, Dong S, Dagotto E Similarities and Differences between Nickelate and Cuprate Films Grown on a SrTiO3 Substrate. Phys Rev B (2020) 102:195117. doi:10.1103/physrevb.102.195117
50. Hansmann P, Yang X, Toschi A, Khaliullin G, Andersen OK, Held K Phys Rev Lett (2009) 103:016401. doi:10.1103/physrevlett.103.016401
51. Rossi M, Osada M, Choi J, Agrestini S, Jost D, Lee Y, et al. arXiv e-prints , arXiv:2112.02484 (2021). arXiv:2112.02484 [cond-mat.supr-con].
Keywords: superconductivity, strongly correlated, first principles calculations, thin films, interfaces and surfaces, nickelates, cuprates
Citation: Bernardini F, Iglesias L, Bibes M and Cano A (2022) Thin-Film Aspects of Superconducting Nickelates. Front. Phys. 10:828007. doi: 10.3389/fphy.2022.828007
Received: 02 December 2021; Accepted: 17 January 2022;
Published: 09 February 2022.
Edited by:
Antia Botana, Arizona State University, United StatesReviewed by:
Kwan-Woo Lee, Korea University, South KoreaCopyright © 2022 Bernardini, Iglesias, Bibes and Cano. This is an open-access article distributed under the terms of the Creative Commons Attribution License (CC BY). The use, distribution or reproduction in other forums is permitted, provided the original author(s) and the copyright owner(s) are credited and that the original publication in this journal is cited, in accordance with accepted academic practice. No use, distribution or reproduction is permitted which does not comply with these terms.
*Correspondence: A. Cano, YW5kcmVzLmNhbm9AbmVlbC5jbnJzLmZy