- 1Laboratory of Space Laser Engineering and Technology, Shanghai Institute of Optics and Fine Mechanics, Chinese Academy of Sciences, Shanghai, China
- 2Key Laboratory of Quantum Optics, Shanghai Institute of Optics and Fine Mechanics, Chinese Academy of Sciences, Shanghai, China
The technique of laser cooling of atoms gives an opportunity to improve the performance of atomic clocks by using laser-cooled atoms. The most successful cold atom clock, called the atomic fountain, is now widely used as the primary frequency standard in many labs. The cold atom clock for satellite applications, however, has not been reported so far due to special requirements of space applications. Here, we report the development of an engineering model of a satellite-borne cold atom clock, which satisfied all requirements of in-orbit operation. The core of the clock’s principle is the laser cooling of atoms by diffuse laser lights inside the microwave cavity. The structure of the physics package is presented, and its main parameters are also given. The principle and design of the optical bench are described. The initial test results are presented, and the possible improvements are also discussed.
1 Introduction
Atomic clocks with laser-cooled atoms have been developed rapidly since laser cooling of atoms was invented [1]. The atomic fountain is now widely used as a frequency standard in many labs, as summarized in Ref. [2], and even in space [3]. In a fountain clock, laser-cooled atoms, typically from a magneto-optical trap (MOT), are launched upward through a microwave cavity and drop downward through the cavity again due to gravity. The double interrogation between cold atoms and microwave gives Ramsey fringes, whose central fringe has a much narrower linewidth compared to the thermal beam. The fountain clock has excellent performance with accuracy and stability for 1 day around
Several schemes are realized to overcome the bulky volume of the atomic fountain. In these schemes, the MOT, microwave interrogation, and detection of atoms are performed inside the same cavity, and the Ramsey interaction is realized by two microwave pulses [4, 5]. Such an arrangement reduces the size of the fountain clock but keeps its character. Since the MOT requires a strong pulsed magnetic field, which affects the microwave cavity, this kind of setup has problems for long-term performance.
Diffuse laser cooling (DLC) is insensitive to the magnetic field and is therefore an ideal method to generate cold atoms in the microwave cavity [6–8]. The cold atom clocks based on DLC have been developed in Paris and Shanghai [9, 10]; Paris’ setup uses a spherical microwave cavity, while Shanghai’s setup is cylindrical. Both obtained excellent performance with a long-term stability around
In this study, we present the development and initial tests of an engineering model of a microwave frequency standard with DLC for satellite systems. Different from the principle model, the engineering model must be designed for non-intervening and long-term continuous operation in space.
2 Design and assembling
The satellite-borne cold atom clock based on DLC has four units, including the physics package, optical bench, microwave electronics, and control system. All units are integrated into one unit, which satisfies the environment inside a satellite.
2.1 Physics package
The physics package includes mainly a vacuum system, a microwave cavity, a cesium source, a magnetic shield, and detection optics, as shown in Figure 1.
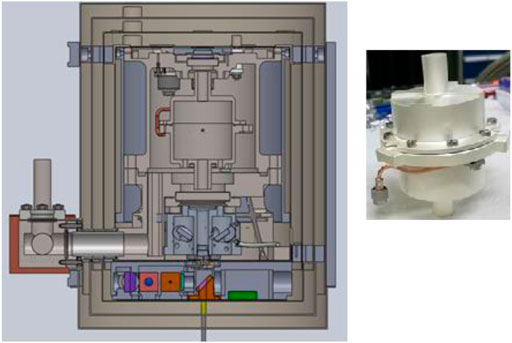
FIGURE 1. Physics package (left) and microwave cavity (right). The package includes a vacuum chamber whose vacuum is maintained using two ion pumps, and the chamber is covered by three layers of the magnetic shield. Inside the chamber is a cylindrical microwave cavity which is made of titanium and coated with silver. Cesium is spread into the microwave cavity from the source. The detection laser passes through the center of the cavity from the bottom to top and reflected.
The core of the physics package is a cylindrical microwave cavity, placed at the center of the vacuum chamber, shown in Figure 1. This cavity, made of titanium with a silver-coated inner surface, shown in Figure 1 (right), has two functions: one acts as a microwave resonator for the interrogation of microwaves with atoms, and the other is for diffuse laser cooling [10]. The cavity is tuned to be resonant with the atomic transition of two cesium ground states at 9.19 GHz with
Vacuum is maintained using two small ion pumps and a getter at around 10−7 Pa, and the vacuum chamber is connected to the Cs source, whose temperature can be adjusted in order to control background Cs vapor inside the vacuum chamber. Outside of the vacuum chamber, there are three layers of magnetic shields to keep the magnetic field inside the microwave cavity below 2 nT. The probing laser beam travels along the central axis of the microwave cavity from one end to another and is reflected back to form a standing wave.
2.2 Optical bench
The optical system is designed for laser cooling, pumping, and detection, and the principle is shown in Figure 2. The main laser beam, locked to the cross peak between
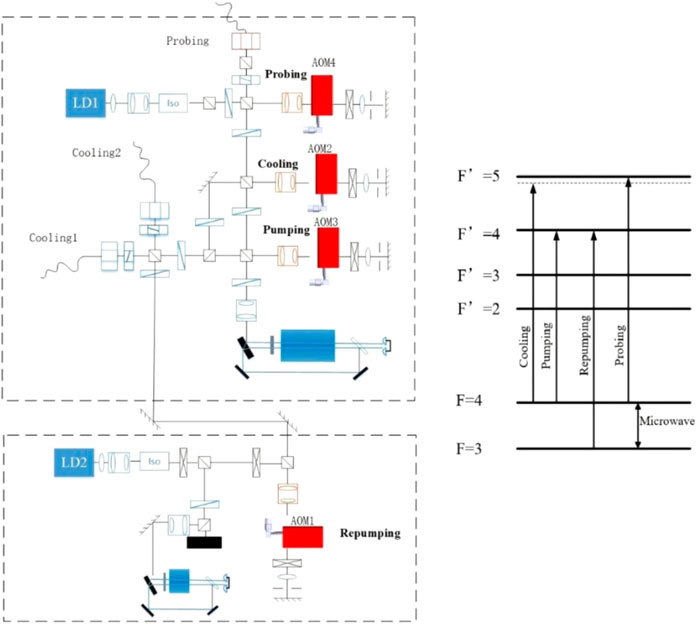
FIGURE 2. Principle of optical systems and energy levels. LD indicates a laser diode, AOM indicates an acoustic optical modulator, and Iso indicates an isolator. LD1 is used for cooling, probing, and pumping. LD2 is used only for repumping. Both lasers are locked by saturation spectroscopy and shifted by AOMs.
The main optical elements of the optical bench are integrated on an aluminum plate, as shown in the left side of Figure 3. Both sides of the plate are used. Moreover, another layer is added, mainly for backup elements, including a laser. Those elements can be switched on when necessary.
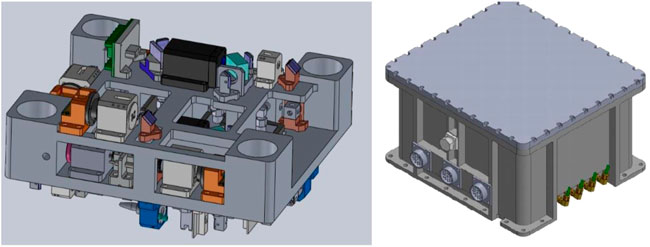
FIGURE 3. Optical bench (left) and its sealing box (right). All optical elements are firmly placed on an aluminum plate. The bench is placed in a sealing box, inside which the pressure is kept at around 1 atmosphere for a long time when the whole clock is placed in vacuum.
The bench is placed in a sealing box for stable operation in vacuum. The sealing box, as shown in the right side of Figure 3, is filled with dried air at 1 atmosphere at 23°C. The sealing is carefully tested so that the pressure inside the box is kept at about 80% of the original one in vacuum for 10 years. Such a change does not affect the performance of optics.
2.3 Microwave electronics and control system
All electronics are integrated into one unit, including microwave and control electronics. A 10 MHz signal from a low-noise OCXO crystal oscillator is synthesized into a 9.192 GHz microwave, which is injected into the cavity to interact with diffuse laser-cooled atoms. Two microwave pulses are used to interrogate cold atoms, as the Ramsey scheme requires.
The control system uses an FPGA to control the time sequence, including laser cooling, pumping, microwave interrogation, and probing, and evaluate the feedback error signal. Moreover, the control system is also used for data processing for laser power monitoring, temperature control, magnetic field control, and necessary communications.
2.4 Assembling
All units are assembled into one unit, as shown in Figure 4. The whole clock is fully functional, and it has a weight of 28 kg with a stable power consumption of around 60 W.
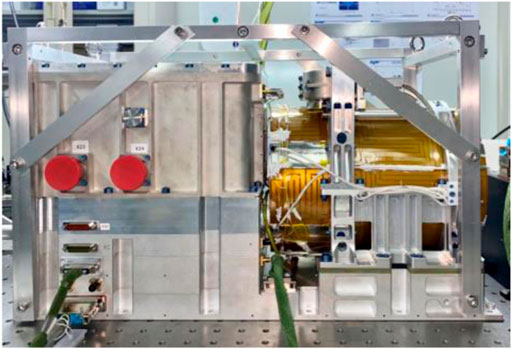
FIGURE 4. Assembled cold atom clock for satellite applications. The bottom shows electronics, top left shows the optical system, and top right shows the physics package.
The clock is designed and tested to satisfy the requirements of the satellite environment. On the ground, the clock is tested in a vacuum chamber which is temperature-controlled, similar to that in a satellite. When the clock is placed in vacuum, the electronics and physics packed are quickly pumped to vacuum, while the optical bench is still at 1 atmosphere due to the protection of the sealing box.
3 Initial tests and discussion
The time sequence for normal operation of the clock is given in Figure 5. The atoms are first cooled by diffuse light in the microwave cavity, typically the cooling and repumping time
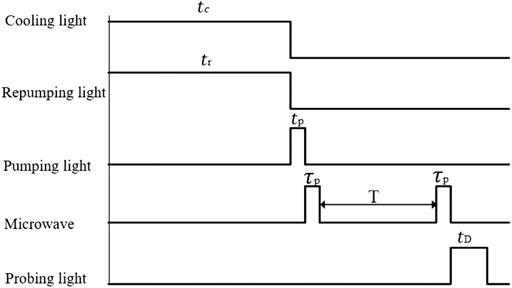
FIGURE 5. Time sequence of the clock operation. Cooling and repumping must be synchronized for effective diffuse laser cooling. A short pumping pulse is used to prepare the system ready for microwave interrogation. Two microwave pulses are used as Ramsey interactions with cold atoms, and the linewidth of the Ramsey fringe is determined by the separation of two pulses. The probing light is used to detect the atomic population after microwave interactions, which is directly related to the microwave frequency.
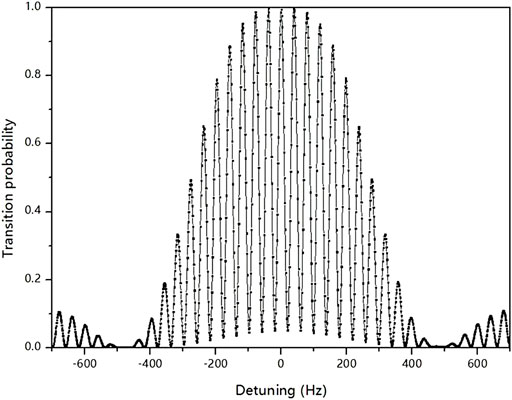
FIGURE 6. Typical Ramsey fringe. The linewidth of the central fringe is 20.0 Hz, and the signal-to-noise ratio is 650.
The full width at half-maximum of the central fringe is measured at around 20.0 Hz, which agrees with the theoretical value of
Figure 7 gives Allan deviation of the cold atom clock with diffuse laser cooling relative to an H-maser. The clock is operated in a temperature-controlled lab at
There is plenty of room for the improvement of the clock. The current time sequence is managed for the specific requirement of the satellite application. A more suitable time sequence can be modified for better performance.
In microgravity, the lifetime of cold atoms in the microwave cavity is much longer than on the ground [13]; thus, the interrogation time can be much longer, and thus, the width of the clock signal can be much narrower. A narrower linewidth leads to better short-term stability, which is expected to be half an order better than on the ground.
In microgravity, the cooling efficiency is several times higher than on the ground due to the lack of gravity [3, 14]. The higher efficiency leads to more cold atoms and a lower temperature, which benefits the signal-to-noise ratio of the clock signal and improves the short-term stability significantly.
DLC based on stimulated forces is also possible [15]. With such a new scheme of DLC, the cooling time can be shortened to even less than 1 ms, which makes the cycle time of clock operation greatly reduced. Such a reduction leads to an increase in short-term stability.
Moreover, the long-term performance of the cold atom clock can benefit from the stable environment inside a satellite in orbit.
4 Conclusion
We have successfully developed a satellite-borne atomic clock with diffuse laser-cooled atoms, tested it in the lab with promising performance, and even compared with the Deep Space Atomic Clock [16]. The clock passes the vibration and thermal tests and has demonstrated long-term operation in vacuum. The results show that a cold atom clock based on DLC can be constructed to satisfy the requirements for satellite application, and its performance can reach even as high as
The techniques developed in the compact cold atom clock can be used in further cold atom-based space quantum sensors.
Data availability statement
The original contributions presented in the study are included in the article/Supplementary Material; further inquiries can be directed to the corresponding authors.
Author contributions
Y-LM for the physics package, X-JJ for testing, JW for optics, M-FY for mechanical design, H-DC for physics principles, LNL for electronics and microwaves, and LGL for general.
Acknowledgments
The authors would like to thank Wang Yiqiu for his long-term support for this project. Back in the early 2000s, when people believed that an atomic clock with lasers could be used only as a lab device, Prof. Wang always encouraged us to continue this project. We all benefit from his deep insight. Now, we have proved that a cold atom clock is reliable enough for satellite applications. On the occasion of his 90th birthday, the authors would like to thank Prof. Wang Yiqiu again for his help to the development of the atomic clocks in our laboratory.
Conflict of interest
The authors declare that the research was conducted in the absence of any commercial or financial relationships that could be construed as a potential conflict of interest.
Publisher’s note
All claims expressed in this article are solely those of the authors and do not necessarily represent those of their affiliated organizations or those of the publisher, the editors, and the reviewers. Any product that may be evaluated in this article or claim that may be made by its manufacturer is not guaranteed or endorsed by the publisher.
References
2. Wang Q, Rong W, Wang YZ. Atomic fountain frequency standard: Principle and development. Acta Phys Sin (2018) 67:163202. doi:10.7498/aps.67.20180540
3. Liu L, Lü DS, Chen WB, Li T, Qu QZ, Wang B, et al. In-orbit operation of an atomic clock based on laser-cooled 87Rb atoms. Nat Commun (2018) 9:2760. doi:10.1038/s41467-018-05219-z
4. Müller ST, Magalhaes DV, Alves RF, Bagnato VS. Compact frequency standard based on an intracavity sample of cold cesium atoms. J Opt Soc Am B (2011) 28:2592. doi:10.1364/josab.28.002592
5. Lee S, Choi GW, Hong HG, Kwon TY, Lee SB, Heo MS, et al. A compact cold-atom clock based on a loop-gap cavity. Appl Phys Lett (2021) 119:064002. doi:10.1063/5.0057150
6. Guillot E, Pottie PE, Dimarcq N. Three-dimensional cooling of cesium atoms in a reflecting copper cylinder. Opt Lett (2001) 26:1639. doi:10.1364/ol.26.001639
7. Cheng HD, Zhang WZ, Ma HY, Liu L, Wang YZ. Laser cooling of rubidium atoms from background vapor in diffuse light. Phys Rev A (Coll Park) (2009) 79:023407. doi:10.1103/physreva.79.023407
8. Wan JY, Wang X, Zhang X, Meng YL, Wang WL, Sun Y, et al. Quasi-one-dimensional diffuse laser cooling of atoms. Phys Rev A (Coll Park) (2022) 105:033110. doi:10.1103/physreva.105.033110
9. Esnault FX, Holleville D, Rossetto N, Guerandel S, Dimarcq N. High-stability compact atomic clock based on isotropic laser cooling. Phys Rev A (Coll Park) (2010) 82:033436. doi:10.1103/physreva.82.033436
10. Liu P, Meng YL, Wan JY, Wang XM, Wang YN, Xiao L, et al. Scheme for a compact cold atom clock based on diffuse laser cooling in a cylindrical cavity. Phys Rev A (Coll Park) (2015) 92:062101. doi:10.1103/physreva.92.062101
11. Meng YL, Cheng HD, Liu P, Zheng Xiao BCL, Wan JY, Wang XM, et al. Increasing the cold atom density in an integrating spherical cavity. Phys Lett A (2014) 378:2034–7. doi:10.1016/j.physleta.2014.05.013
12. Meng YL, Cheng HD, Zheng BC, Wang XC, Xiao L, Liu L. Controlling the shape of a cold atom cloud in a cylindrical cavity. Chin Phys Lett (2013) 30:063701. doi:10.1088/0256-307x/30/6/063701
13. Xiao L, Wang XC, Zheng BC, Meng YL, Cheng HD, Liu L, et al. Kinetic behavior of cold 87Rb atoms in integrating sphere. Optik (2012) 123:442–6. doi:10.1016/j.ijleo.2011.03.035
14. Becker D, Lachmann MD, Seidel ST, Ahlers H, Dinkelaker AN, Grosse J, et al. Space-borne Bose–Einstein condensation for precision interferometry. Nature (2018) 562:391–5. doi:10.1038/s41586-018-0605-1
15. Metcalf H. Colloquium: Strong optical forces on atoms in multifrequency light. Rev Mod Phys (2017) 89:041001. doi:10.1103/revmodphys.89.041001
Keywords: diffuse laser cooling, satellite-borne, cold atom clock, microwave frequency standard, satellite navigation
Citation: Meng Y-L, Jiang X-J, Wu J, Ye M-F, Cheng H-D, Li L and Liu L (2022) Satellite-borne atomic clock based on diffuse laser-cooled atoms. Front. Phys. 10:985586. doi: 10.3389/fphy.2022.985586
Received: 04 July 2022; Accepted: 22 July 2022;
Published: 16 September 2022.
Edited by:
Jingbiao Chen, Peking University, ChinaReviewed by:
Bin Jian, National Research Council Canada (NRC-CNRC), CanadaJiteng Sheng, East China Normal University, China
Copyright © 2022 Meng, Jiang, Wu, Ye, Cheng, Li and Liu. This is an open-access article distributed under the terms of the Creative Commons Attribution License (CC BY). The use, distribution or reproduction in other forums is permitted, provided the original author(s) and the copyright owner(s) are credited and that the original publication in this journal is cited, in accordance with accepted academic practice. No use, distribution or reproduction is permitted which does not comply with these terms.
*Correspondence: Lin Li, bGlsaW5Ac2lvbS5hYy5jbg==; Liang Liu, bGlhbmcubGl1QHNpb20uYWMuY24=