- Department of Chemistry, Carnegie Mellon University, Pittsburgh, PA, United States
Photoluminescent nanosized quasi-spherical polymeric assemblies prepared by the hydrothermal reaction of polyacrylonitrile (PAN), ht-PLPPAN, were demonstrated to have the ability to photo-induce atom transfer radical polymerization (ATRP) catalyzed by low, parts per million concentrations of CuII complex with tris(2-pyridylmethyl)amine (TPMA). Such photo induced ATRP reactions of acrylate and methacrylate monomers were performed in water or organic solvents, using ht-PLPPAN as the photo-cocatalyst under blue or green light irradiation. Mechanistic studies indicate that ht-PLPPAN helps to sustain the polymerization by facilitating the activation of alkyl bromide species by two modes: 1) green or blue light-driven photoreduction of the CuII catalyst to the activating CuI form, and 2) direct activation of dormant alkyl bromide species which occurs only under blue light. The photoreduction of the CuII complex by ht-PLPPAN was confirmed by linear sweep voltammetry performed under illumination. Analysis of the polymerization kinetics in aqueous media indicated even though CuI complexes comprised only 1–1.4% of all Cu species at equilibrium, they exhibited high activation rate constant and activated the alkyl bromide initiators five to six orders of magnitude faster than ht-PLPPAN.
Introduction
Photocatalytic reactions play a profound role in various areas of chemical research (Zhu and Wang, 2017; Melchionna and Fornasiero, 2020). As alternatives to traditionally used transition metal complexes, organic photocatalysts (OPC) have received great attention, due to their low cost, highly tunable structures and photophysical properties (Romero and Nicewicz, 2016). More recently, organic photoactive nanostructured objects (OPNO) have emerged as promising materials owing to the growing interest in combining organic chemistry and nanotechnology (Han et al., 2018; Zhang et al., 2020). OPNO can span the range from covalently bonded “carbon dots” (Sun et al., 2006) to “polymer dots” (Zhu et al., 2015a; Zhu et al., 2015b; Tao et al., 2019; Xia et al., 2019) held together via non-covalent interactions. The synthesis of the latter type of OPNOs often relies on the pre-assembly or self-assembly of polymeric substrates, which afford additional control over the structures and properties of OPNOs (Chen and Tseng, 2017; Jia et al., 2017). Because of the heterogeneous nature of their photoactive domains, many OPNOs absorb broadly from UV to near infrared (NIR) light, making them promising candidates as photocatalysts, due to the tunable irradiation wavelength (Zhu et al., 2015b).
Nonetheless, two main limitations remain for OPNO-based OPCs. First, most reports only focused on reactions in organic solvents. Water-soluble photocatalysts and aqueous photochemical reactions were largely omitted. Second, reactions using long-wavelength irradiations (other than blue or UV light) were underexplored, despite the advantages of long-wavelength irradiations, such as better penetration depths (Romero and Nicewicz, 2016; Han et al., 2018). Overcoming these limitations is necessary for expanding the applications of OPNO-based OPCs, obtaining deeper understandings of the photocatalytic mechanism, and economizing the photocatalytic processes (Zhang et al., 2020).
The key to solve these challenges is advancing the synthesis of OPNOs, which is traditionally limited to a narrow selection of substrates, such as citric acid/ethylenediamine (Zhu et al., 2013), conjugated polymers (Zhao et al., 2019) etc., Recently, polyacrylonitrile (PAN) has caught the attention of many researchers. PAN has been used as a substrate in the manufacturing of carbon fibers and heteroatom-doped nanocarbons (Tang et al., 2005; Zhong et al., 2012; Kopeć et al., 2017; Gottlieb et al., 2019; Kopeć et al., 2019; Yuan et al., 2019; Yuan et al., 2020). The photoluminescent properties of PAN were largely ignored, since untreated isolated nitrile groups do not form photoactive conjugated structures. However, recent reports point out that PAN can generate photoluminescence when dissolved at high concentrations or densely grafted from flat silica surfaces (Jang et al., 2006; Zhou et al., 2016; Kopeć et al., 2020). Additionally, there are recent reports of chemical conversion of PAN into photoactive species via microwave (Go et al., 2017), pyrolysis (Cao et al., 2020) and hydrothermal reactions (Ermakov et al., 2000; Sun et al., 2020), which all can induce the crosslinking or the hydrolysis of -C≡N, resulting in photoluminescent crosslinked nanoparticles or polymers. In particular, we recently reported the synthesis of visible light-absorbing photoluminescent polymers (PLPs) from PAN via a one-step hydrothermal reaction (Sun et al., 2020). In certain solvents (e.g., water), the resulting PLPs can self-assemble into polymer dot-like OPNOs. For example, the hydrothermally synthesized photoluminescent polymer (ht-PLPPAN) assembles in water into spherical aggregates with an average diameter of ca. 20 nm, due to the presence of both hydrophilic (i.e., carboxylic) and hydrophobic (i.e., aliphatic carbons) moieties. The assembly behavior was studied mainly by dynamic light scattering (DLS) in multiple solvents, including water and dimethyl sulfoxide (DMSO) (Sun et al., 2020). The high water solubility (>100 mg/ml) makes ht-PLPPAN a promising candidate as an OPNO-based OPC in aqueous systems.
One of the most particularly promising photochemical processes in polymer chemistry is photoinduced controlled radical polymerization (CRP), which has emerged as a powerful and versatile method for controlled polymer syntheses (Pan et al., 2016a; Chen et al., 2016; Dadashi-Silab et al., 2016; Corrigan et al., 2020; Parkatzidis et al., 2020). Similar to other externally controlled CRP methods (Chmielarz et al., 2017; Mohapatra et al., 2017; Wang et al., 2017; Pan et al., 2018), photoinduced CRPs exhibit many advantages, such as excellent temporal control. In particular, photoinduced atom transfer radical polymerization (ATRP) (Wang and Matyjaszewski, 1995; Matyjaszewski and Xia, 2001; Matyjaszewski, 2012; Dadashi-Silab et al., 2014; Matyjaszewski and Tsarevsky, 2014; Theriot et al., 2016; Matyjaszewski, 2018; Lorandi and Matyjaszewski, 2020) and reversible addition-fragmentation chain-transfer (RAFT) polymerization (Xu et al., 2015; Pearson et al., 2016; Perrier, 2017; Allegrezza and Konkolewicz, 2021) have largely benefited from the development of OPCs, such as phenothiazine derivatives (Treat et al., 2014; Pan et al., 2016b; Theriot et al., 2016; Dadashi-Silab et al., 2021), eosin Y (Kutahya et al., 2016; El Achi et al., 2020) and halogenated xanthene dyes (Wu et al., 2019). Besides small-molecule OPCs, OPNOs have been applied in photoinduced CRP (Jiang et al., 2018; Kütahya et al., 2020; Hao et al., 2021; Kütahya et al., 2021). For example, heteroatom-doped carbon dots were applied in photoinduced energy/electron transfer RAFT (PET-RAFT) polymerization of (meth)acrylate monomers (Jiang et al., 2018). Doping S or P to the catalyst enabled successful PET-RAFT polymerization under red light. Additionally, photoinduced ATRP using low ppm (parts per million) loadings of Cu complexes was performed using carbon dots or polymer dots under blue light irradiation (Kütahya et al., 2020; Kütahya et al., 2021). In these systems, carbon dots reduced the CuII complex to the corresponding CuI complex, which activated the alkyl bromide initiator. CuII complexes functioned as deactivators to provide control over the polymerization (Kütahya et al., 2020).
Nevertheless, photoinduced CRPs in the presence of OPNOs primarily employed organic solvents and oleophilic monomers (Jiang et al., 2018; Kütahya et al., 2020; Kütahya et al., 2021). Although photoinduced CRPs in aqueous media were reported using other types of catalysts (Konkolewicz et al., 2012; Pan et al., 2015; Szczepaniak et al., 2020), extending these processes to new catalysts with different structures and photoluminescent mechanisms is needed to expand the understanding of the reaction mechanisms. Furthermore, ATRP using OPNOs under long-wavelength irradiations is underexplored compared to RAFT polymerization.
Herein, we expand the applicability of photoinduced ATRP by developing Cu-catalyzed ATRP in the presence of ht-PLPPAN as OPCs under blue (λmax = 450 nm) and green (λmax = 520 nm) light irradiation. During initial attempts, ht-PLPPAN did not initiate the polymerization when used as a photoinitiator in free radical polymerization (FRP) under inert atmosphere (N2). Nonetheless, well-controlled polymerizations were successfully performed in water and in organic solvents (DMSO, dimethylformamide or DMF, and anisole) in the presence of alkyl bromide initiators and 25–500 ppm of CuII complexes (Br-CuII/L, L = ligand). A low loading of ht-PLPPAN (<1 mg/ml) and moderate light intensities (4–6 mW.cm−2) were used. Aqueous Cu-catalyzed ATRP of a water-soluble monomer, oligo (ethylene glycol) methyl ether methacrylate (OEGMA), is shown in Scheme 1. Studies performed under different irradiation wavelengths revealed that under blue light irradiation ht-PLPPAN could both reduce the CuII complexes and activate alkyl halides. In contrast, under green light irradiation it was only capable to reduce the CuII complexes.
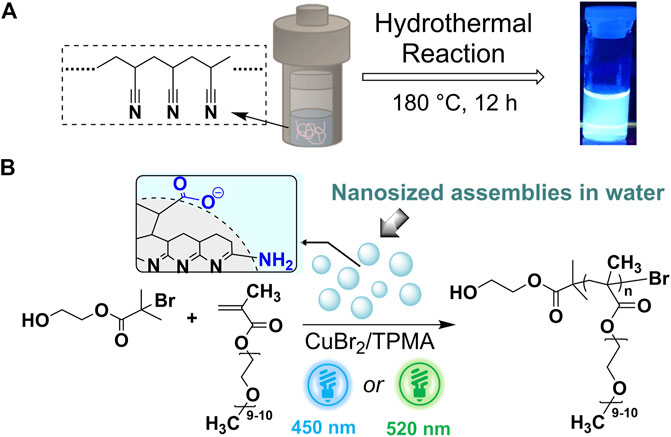
SCHEME 1. (A) Schematic representation of the synthesis of ht-PLPPAN, and an image of ht-PLPPAN dissolved in water under UV light excitation (365 nm) (B) Proposed assembled structure of ht-PLPPAN based on the previous report (Sun et al., 2020), and the use of ht-PLPPAN as the photo-cocatalyst in Cu-catalyzed light-mediated ATRP. Functional groups highlighted in blue represent typical hydrophilic fragments.
Materials and Methods
Materials
Methyl methacrylate (MMA, 99%, Sigma-Aldrich, United States), oligo (ethylene glycol) methyl ether methacrylate (OEGMA, average MW = 500, Sigma-Aldrich, United States), acrylonitrile (AN, 99%, Sigma-Aldrich) and methyl acrylate (MA, 99%, Sigma-Aldrich, United States) were purified by passing the monomers through a column filled with basic alumina to remove the inhibitor. Deionized water (DI water) was obtained from Millipore-Sigma Milli-Q water purification system. Azobisisobutyronitrile (AIBN, 98%, Sigma-Aldrich, United States) was recrystallized in anisole and stored at 4°C in dark. Tris (2-pyridylmethyl)amine (TPMA) was synthesized based on previously reported methods (Xia and Matyjaszewski, 1999). Copper bromide (CuBr2, 99%, Acros Organics, United States), ethyl α-bromoisobutyrate (EBiB, 98%, Sigma-Aldrich, United States), ethyl α-bromophenylacetate (EBPA, 97%, Sigma-Aldrich, United States), 2-hydroxyethyl 2-bromoisobutyrate (HO-EBiB, 95%, Sigma-Aldrich, United States), 2-bromopropionitrile (BPN, 97%, Sigma-Aldrich, United States), deuterated DMSO (DMSO-d6, 99.9%, Cambridge Isotope Laboratories, United States), deuterium oxide (D2O, 99.9%, Cambridge Isotope Laboratories), anisole (99%, Sigma-Aldrich, United States), dimethyl sulfoxide (DMSO, 99.7%, Fisher, United States), toluene (99%, Fisher, United States), and dimethylformamide (DMF, 99.8%, Fisher, United States) were used as received. Tetraethylammonium tetrafluoroborate (Et4NBF4, 99%, Alfa Aesar, United States), used as a supporting electrolyte for electrochemical analysis, was recrystallized from ethanol, and dried in a vacuum oven at 70°C for 48 h.
Instruments
1H nuclear magnetic resonance (NMR) was conducted on a Bruker 500 MHz AVANCE III NMR spectrometer. Polymerizations in organic solvents used DMSO-d6 as the solvent for 1H NMR, and D2O for aqueous ATRP. DMF GPC was equipped with a refractive index (RI) detector, an Agilent 1260 Infinity II pump and a Wyatt Optilab T-rEX RI detector, with a PSS GRAM analytical column set (10 µm particle size) and LiBr-containing HPLC grade DMF as the eluent (LiBr: 0.05 M). Agilent EasiVial poly (methyl methacrylate) (PMMA) calibration kit (part number: PL 2020-0200) was used to calibrate the RI detector of GPC. Blue (λmax = 450 nm, 5.0 mW.cm−2) and green (λmax = 520 nm, 4.7 mW.cm−2) LED lights were purchased from aspectLED. The light intensity was measured by a ThorLabs PM100D compact power and energy meter console. The photoreactor for polymerization was prepared by mounting LED strips inside a round glass container, and air flow was applied to limit temperature increase during the polymerization. Solvent evaporation was performed on a Biotage V10 rapid solvent removal system. UV-vis spectra were taken on an Agilent Cary 60 UV-vis spectrometer and recorded by Cary WinUV software. Linear sweep voltammetries were measured by an Autolab PGSTAT302N potentiostat/galvanostat (Metrohm) run by a computer with NOVA 2.0 software. Dynamic light scattering (DLS) was measured by a Malvern Zetasizer Ultra light scattering system. Transmission electron microscopy (TEM) was performed using a JEOL JEM-2000EX TEM.
Synthesis of ht-PLPPAN
ht-PLPPAN, was synthesized via previously reported procedures (Sun et al., 2020). PAN165 (subscript defines the degree of polymerization) was used to prepare ht-PLPPAN and was synthesized by initiators for continuous activator regeneration (ICAR) ATRP using BPN as the initiator (Lamson et al., 2016; Kopeć et al., 2017). In a typical procedure, a 10 mg/ml suspension of ball-milled PAN165 in DI water was prepared. 10 ml suspension was stirred for 10 min and then transferred to a 25 ml autoclave reactor with high-temperature resistant liner. The autoclave reactor was securely sealed and placed in a pre-heated oven (180°C). The heating was turned off after 12 h and the oven was let to cool down to room temperature naturally. The dark brown solution was passed through a 0.22 µm syringe filter with a polyethersulfone membrane. The solution was directly dried by vacuum to yield brown solid ht-PLPPAN.
General Procedure for Light-Mediated ATRP in the Presence of ht-PLPPAN
In a typical procedure, 5 mg ht-PLPPAN was added to a 10 ml Schlenk flask containing 0.3 ml DMF and 7.2 ml DI water with a magnetic stir bar. HO-EBiB (3.9 µL, 0.027 mmol, 1 eq), CuBr2 (0.60 mg, 2.7 µmol, 0.1 eq), TPMA (2.35 mg, 8.1 µmol, 0.3 eq), NaBr (41.17 mg, 0.4 mmol), OEGMA (2.5 ml, 5.4 mmol, 200 eq) were subsequently added to the Schlenk flask. The Schlenk flask was then purged with N2 for approx. 25 min, and 0.1 ml of the reaction was withdrawn and was used as the “t = 0” sample. Finally, the Schlenk flask was placed in the photoreactor, and light was turned on to start the polymerization. The conversion of OEGMA was monitored by 1H NMR by withdrawing samples (∼0.1 ml each time) from the reaction mixture at different time points.
General Procedures for Voltammetric Measurements
Linear sweep voltammetries were carried out in a 5-neck electrochemical cell placed inside the photoreactor, equipped with a 3-electrode system and connected to an Autolab PGSTAT302N potentiostat/galvanostat (Metrohm) controlled by NOVA 2.0 software. The 3-electrode system was composed by: 1) a Pt foil counter electrode; 2) a homemade quasi-reference electrode: Ag|AgI|(0.1 M n-Bu4NI in DMF); 3) a glassy carbon (GC) disk tip (3 mm dia, Metrohm), connected to a rotating disk electrode (RDE) system, as working electrode. Before each experiment, the GC disk was cleaned by polishing with a 0.25-μm diamond paste, followed by ultrasonic rinsing in ethanol for 5 min. Ferrocene (Fc) was added at the end of each experiment as an internal standard, to refer all potentials to the saturated calomel electrode [SCE, E°(Fc+|Fc) = 0.475 V vs SCE in DMF]. A steady air flow was applied to limit the temperature increase caused by light irradiation, and all experiments were performed under inert atmosphere (N2).
Results and Discussion
Photophysical Properties of ht-PLPPAN
ht-PLPPAN was synthesized based on a previously reported hydrothermal reaction (Sun et al., 2020). Dynamic light scattering (DLS) measurement suggested that ht-PLPPAN formed aggregates in water (Supplementary Figure 1). Transmission electron microscopy (TEM) revealed that the average diameter of the quasi-spherical assemblies was ca. 20 nm (Supplementary Figure 2). At first, using ht-PLPPAN as a photoinitiator in FRP was attempted under blue or green light irradiation. However, no polymerization was observed under N2 atmosphere in the absence of additional initiators (Table 1, entries 5 and 9, discussed in detail later). Thus, our focus shifted to using ht-PLPPAN as a reducing agent for the CuBr2 complex with a common ATRP ligand, tris(2-pyridylmethyl)amine (TPMA). (Xia and Matyjaszewski, 1999).
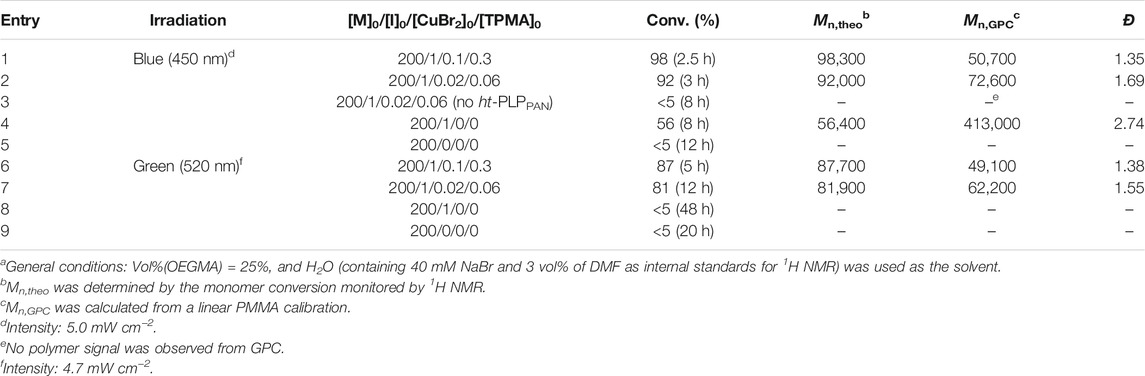
TABLE 1. Cu-catalyzed photoinduced ATRP of OEGMA500 in watera with HO-EBiB as the initiator and ht-PLPPAN as the photo-cocatalyst (0.5 mg/ml).
To evaluate the capability of ht-PLPPAN in photoreducing the CuBr2/TPMA complex, photophysical properties of ht-PLPPAN (Figure 1) were analyzed. Our previous study reported a broad UV-vis absorption profile (Figure 1A) and a short lifetime of the excited state ht-PLPPAN (<5 ns), suggesting the singlet nature (Sun et al., 2020). Putative photophysical properties of ht-PLPPAN were modeled using density functional theory (DFT) calculations with model oligoimine-based structures of different conjugation lengths oIN (where N = 3–10 denotes the number of nitrogen atoms along the backbone), which were deemed likely to arise in the course of hydrothermal treatment (Figure 1B, see Supplementary Material for Cartesian coordinates). (Sun et al., 2020) In all instances, the energies of the lowest unoccupied molecular orbital (LUMO) of oIN were higher than the LUMO (β) of [Br-CuII/TPMA]+, indicating conditions favorable for reduction of the CuII complex upon photoexcitation (Figure 1C). The LUMOoIN-LUMO[Br-CuII/TPMA]+ gap increased, and the wavelength of the lowest-energy transition determined by time-dependent DFT (TDDFT) decreased with the decrease of N (Supplementary Figure 3), suggesting stronger “reducing power” of blue light absorbing species, in agreement with experimental observations. Experimental studies on the photoreduction of the CuII complex were performed using linear sweep voltammetry (LSV), which are discussed in Photocatalytic Mechanism and Comparison Between Activation by CuIComplex and by ht-PLPPAN section.
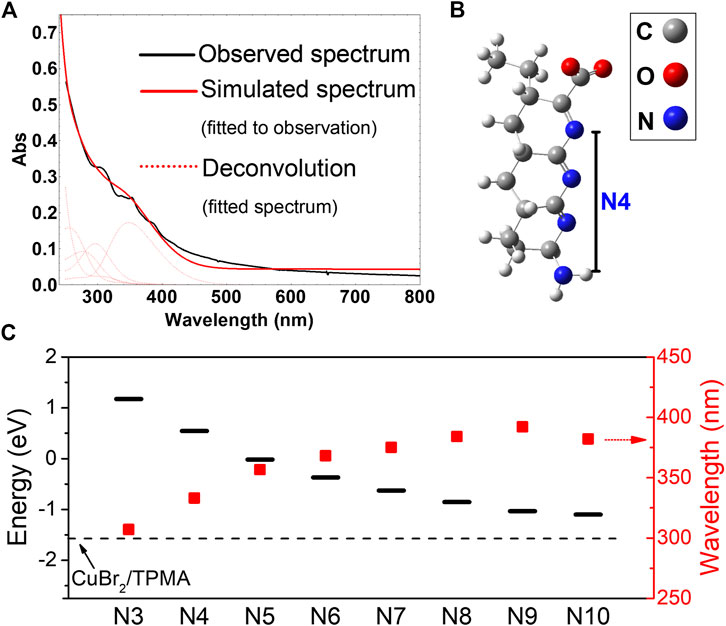
FIGURE 1. Photophysical properties of ht-PLPPAN(A) Experimental and simulated UV-vis spectra of ht-PLPPAN calculated by time-dependent density functional theory (TDDFT). Structure optimization and TDDFT calculations were performed in simulated water environment using a polarizable continuum model, at the ωB97X-D/6-31g-d and cam-b3lyp/6-31g-d levels, respectively (B) A representative model structure for photoactive domains of ht-PLPPAN with four nitrogen atoms (oI4), optimized at the ωB97X-D/6-31g-d level (Stephens et al., 1994; Frisch et al., 2016). (C) Energy levels of the lowest unoccupied molecular orbital (LUMO, black bars) and wavelengths of the lowest energy transition (red squares) of model structures (oI3 to oI10) with different conjugation lengths represented by the number of nitrogen atoms within the backbone (Sun et al., 2020). The dashed line represents the energy of the LUMO (β) of the [Br-CuII/TPMA]+ complex in water [optimized at the ωB97X-D level with def2TZVP basis set on Cu and 6-31g-d basis set on the other atoms (Miertuš and Tomasi, 1981; Chai and Head-Gordon, 2008)].
Cu-Catalyzed ATRP in Water Using ht-PLPPAN
Blue (λmax = 450 nm) and green light sources (λmax = 520 nm) were chosen for photoinduced ATRP using ht-PLPPAN. Oligo (ethylene glycol) methyl ether methacrylate with an average molecular weight of 500 (OEGMA500) was polymerized using ATRP catalyzed by a CuBr2-complex with TPMA as the ligand (molar ratio: CuBr2/TPMA = 1/3) (Table 1). 100 ppm or 500 ppm (relative to the monomer concentration) of CuBr2/TPMA were used, with 2-hydroxyethyl 2-bromoisobutyrate (HO-EBiB) as the ATRP initiator and 0.5 mg/ml ht-PLPPAN as the photo-cocatalyst. Additionally, 40 mM of NaBr was added to suppress the dissociation of the weak CuII-Br bond in the ATRP deactivator (Simakova et al., 2012; Fu et al., 2018).
First, polymerizations were performed under blue light irradiation. Linear semilogarithmic kinetic plots for ATRP using both 500 ppm (entry 1, Table 1) and 100 ppm (entry 2, Table 1) of the CuII complex are shown in Figure 2A. Polymer molecular weight and dispersity (Đ) were measured by gel permeation chromatography (GPC) using dimethylformamide (DMF) as the mobile phase (Figure 2B). Increasing the loading of CuBr2/TPMA from 100 ppm (entry 2) to 500 ppm (entry 1) resulted in a decreased Đ from 1.69 (entry 2) to 1.35 (entry 1), due to the higher equilibrium concentration of the deactivator. Clean shifts of the molecular weight distribution (MWD) traces are shown in Supplementary Figure 4. It is worth mentioning that the difference in the molecular weight measured from the light scattering (LS) detector of GPC (Mn,GPC) and the theoretical molecular weight (Mn,theo) was likely due to different polymer-column interactions between poly (OEGMA) and the calibration standard (PMMA) used for the calibration of the LS detector. Figure 2A also illustrates that the rate of polymerization for entry 1 (500 ppm of Cu complex) was faster than that for entry 2 (100 ppm of Cu complex). This difference was attributed to the higher concentration of propagating radicals in entry 1 and faster reduction of the CuII complex. Similar kinetic results were previously reported by several ATRP methods based on activator regeneration (Simakova et al., 2012; Mendonça et al., 2014). Despite the lower monomer conversion determined by 1H NMR, entry 2 showed a higher Mn,GPC than entry 1 (Table 1). This difference indicated that the initiation efficiency of HO-EBiB was lower when a lower loading of CuBr2/TPMA was used in entry 1.
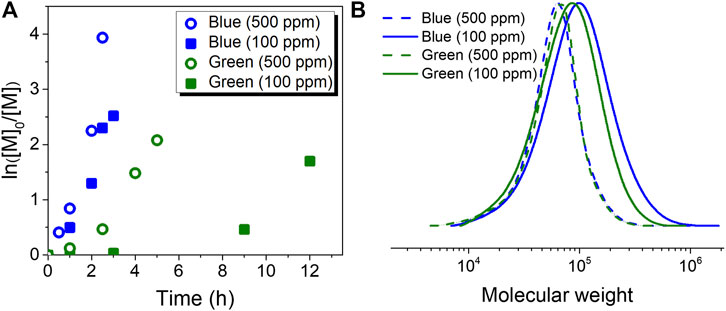
FIGURE 2. Photoinduced ATRP in water (A) First-order kinetic plots corresponding to entries 1 (blue, 500 ppm), 2 (blue, 100 ppm), 6 (green, 500 ppm) and 7 (green, 100 ppm) of Table 1(B) GPC traces corresponding to the polymer product of entries 1, 2, 6 and 7 of Table 1.
In order to confirm the role of ht-PLPPAN, a control experiment was performed in its absence (entry 3, Table 1). No substantial monomer conversion (<5% after 8 h) was observed, indicating that the ht-PLPPAN was required to generate the CuI activator complex. In addition, the possibility of direct activation of the alkyl bromide (R-Br) initiator by ht-PLPPAN was considered. A control experiment (entry 4, Table 1) showed that under blue light irradiation HO-EBiB was indeed activated in the presence of ht-PLPPAN and in the absence of the CuII complex. The polymerization was uncontrolled (Đ = 2.74) due to the absence of CuII deactivators. In comparison, no polymerization occurred when no HO-EBiB was added, indicating that ht-PLPPAN did not generate radicals directly from the monomer. A quantitative comparison between the activation of R-Br by the CuI complex and by ht-PLPPAN is presented in the Photocatalytic Mechanism section.
Similar polymerizations were conducted under green light irradiation (entries 6–7, Table 1 and Figure 2). In general, polymerization rates under green light irradiation were slower than those under blue light irradiation, and proceeded only after an induction period (Figure 2A). Finally, similar control experiments (entries 8 and 9, Table 1) were performed under green light. In contrast with the control experiments performed under blue light, no polymerization was observed under green light irradiation in the absence of CuII, indicating that ht-PLPPAN luminophores excited by this range of wavelengths were not capable to activate the alkyl bromide initiator under green light, even after prolonged irradiation (48 h, entry 8, Table 1).
In addition to the already discussed lower “reducing power” of longer-wavelength luminophores inferred from DFT model calculations, two other factors that could be responsible for the slower polymerization and absence of ht-PLPPAN-driven activation of R-Br under green light are: 1) the lower light absorption of ht-PLPPAN at 520 nm (Figure 1), and 2) the slightly lower intensity of the green light photoreactor (4.7 mW.cm−2, vs 5.0 mW.cm−2 of the blue light photoreactor). Nevertheless, green light irradiation still resulted in reasonably well-controlled polymerization of OEGMA500 (Đ = 1.38) using 500 ppm of CuII/TPMA (entry 6, Table 1).
Cu-Catalyzed ATRP in Organic Solvents Using ht-PLPPAN
To demonstrate the versatility of ht-PLPPAN as OPCs, a mixed solvent containing 1:1 (v/v) of DMSO and DMF was first used to polymerize MMA with ethyl α-bromophenylacetate (EBPA) as the initiator. It is worth mentioning that ht-PLPPAN only partially dissolved when using DMSO/DMF due to the lower polarity of the reaction media, especially after the addition of MMA. Despite the weaker solubility of ht-PLPPAN, well-controlled polymerizations were obtained with ht-PLPPAN dispersed in the solvent using 100 ppm or 25 ppm of CuBr2/TPMA as the catalyst (Table 2).
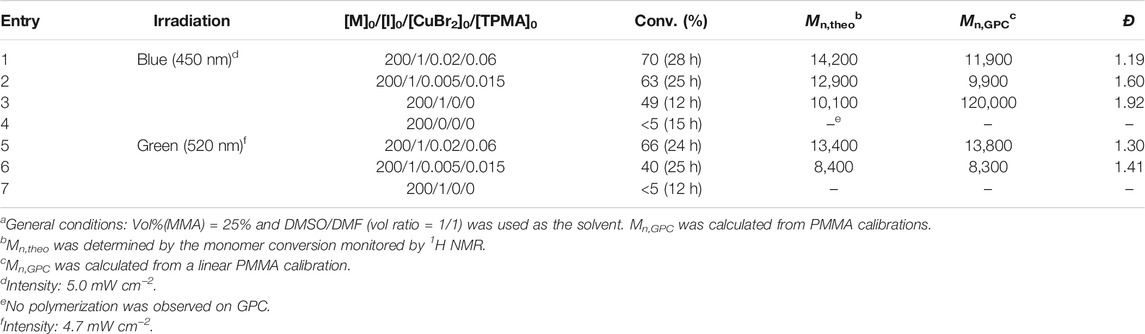
TABLE 2. Cu-catalyzed light-mediated ATRP of MMA in DMSO/DMFa with EBPA as the initiator and ht-PLPPAN as the photo-cocatalyst (0.95 mg/ml).
Similar to the case of aqueous media, better control was obtained for the polymerization of MMA with a higher loading of CuBr2/TPMA (entries 1 and 5, Table 2). However, 500 ppm of the Cu complex were required to control the process in aqueous media (entries 1 and 6, Table 1), while concentrations of CuII complex as low as 25 ppm were sufficient in organic solvents (entry 6, Table 2). Linear semilogarithmic kinetic plots and the GPC traces observed under those conditions are shown in Figure 3. Clean shifts of GPC traces were also observed (Supplementary Figure 5). Additionally, the direct activation of EBPA by ht-PLPPAN was evaluated by control experiments in the absence of CuBr2/TPMA (entries 3 and 7, Table 1). Similar to results in aqueous media, ht-PLPPAN activated EBPA only under blue light irradiation. The supplemental activation of EBPA under blue light irradiation likely contributed to the higher dispersity when 25 ppm loading of CuBr2/TPMA was used (entry 2). The uncontrolled polymerization in the absence of any Cu complexes indicated that no deactivations occurred due to the absence of deactivators.
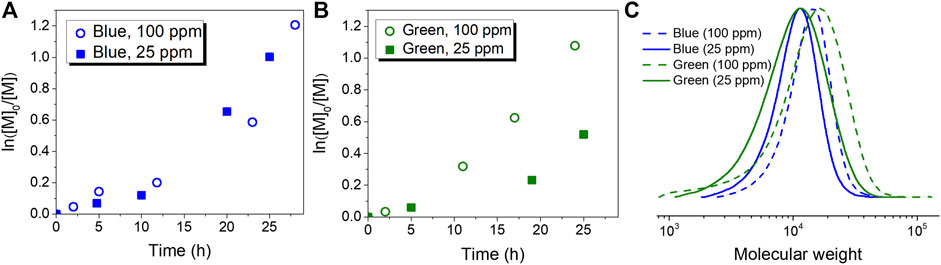
FIGURE 3. Photoinduced ATRP in organic solvents. First-order kinetic plots corresponding to (A) entries 1 (blue, 100 ppm) and 2 (blue, 25 ppm) (B) entries 5 (green, 100 ppm) and 6 (green, 25 ppm) of Table 2. (C) GPC traces corresponding to the polymer product of entries 1, 2, 5 and 6 of Table 2.
Finally, ATRP using ht-PLPPAN was extended to other monomers and solvents (Supplementary Table 1). For example, methyl acrylate (MA) was polymerized using 100 ppm of the CuBr2/TPMA complex and ethyl α-bromoisobutyrate (EBiB) as the initiator (entry 1, Supplementary Table 1). Good control over the polymerization was illustrated by the low Đ = 1.16 of the polymer (Supplementary Figures 6A,B). Additionally, ATRP of MMA was performed using 100 ppm of the CuBr2/TPMA complex and anisole as a less polar solvent (entry 2, Supplementary Table 1). The rate of polymerization was slower in anisole compared to DMSO/DMF, as expected from the variation of the ATRP equilibrium constant, KATRP, with solvent polarity (Ribelli et al., 2019). Nonetheless, well-controlled polymerization (Đ = 1.19) was still observed (Supplementary Figures 6C,D).
Photocatalytic Mechanism and Comparison Between Activation by CuI Complex and by ht-PLPPAN
In some ATRP methods with regeneration of the CuI/L activator, the agent used for the activator regeneration can also contribute to the activation of dormant alkyl bromides to form propagating radicals, which generally occurs by reaction between the agent and the alkyl bromide (Konkolewicz et al., 2013; Konkolewicz et al., 2014a; Konkolewicz et al., 2014b; Zhang et al., 2011). To better understand the polymerization mechanism of the current system, the photoreduction of the CuII complex by ht-PLPPAN was first monitored by linear sweep voltammetry (LSV). As shown in Figure 4, LSV was scanned from ca. 0.5 V (vs SCE) where the CuI complex is oxidized if it exists. Indeed, the increased intensity of the anodic current under blue or green light irradiation confirmed the formation of CuI/TPMA complex in DMSO/DMF (volume ratio: 1/1) (Dadashi-Silab et al., 2021). The role of ht-PLPPAN as the primary factor behind the observed reduction of CuII/TPMA complexes after 3 h of blue light irradiation was confirmed by comparing the fraction of CuI complexes estimated from the current values in the presence of ht-PLPPAN (ca. 2.5%) and in its absence (<0.5%, Supplementary Figure 7A). The small extent of photoreduction in the latter case was likely caused by the presence of excess ligand that can act as the electron donor. Notably, the LSV traces acquired in water did not show a consistent increase of CuI/L concentration (Supplementary Figure 7B), in contrast with polymerization results (Table 1) that indicated ht-PLPPAN was capable of photoreducing the CuII complex in water. This apparent discrepancy indicates that the amount of CuI produced by ht-PLPPAN in water was below the reliable LSV detectability threshold but was still sufficient to effectively initiate polymerization owing to the higher KATRP of Cu catalysts in water (Tang et al., 2008; Fantin et al., 2015). It should be pointed out that the apparent lower reducing efficiency of ht-PLPPAN in water in comparison with organic solvents could be caused by its nano-assembly, which, while important for allowing these mostly hydrophobic species to function in water, would inevitably decrease the number of accessible photocatalytic sites.
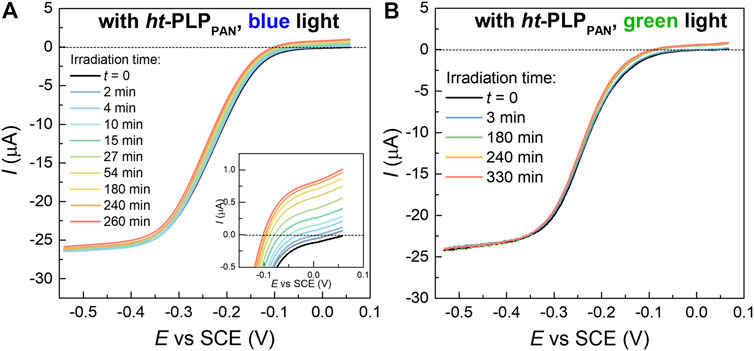
FIGURE 4. LSV of 0.6 mM CuBr2/TPMA (L/Cu = 3) in DMSO-DMF (volume ratio: 1/1) + 0.1 M Et4NBF4 at v = 0.01 V.s−1 and RDE rotation speed 4,000 rpm, under (A) blue light and (B) green light irradiation, in the presence of ht-PLPPAN (1.5 mg/ml).
To further understand the composition of the catalytic system for aqueous ATRP under blue light irradiation, the following calculations were performed to quantify the concentrations of CuI and CuII species in polymerizations corresponding to conditions in Table 1. The activation rates of alkyl bromides by CuI/L and ht-PLPPAN were estimated for the polymerization of OEGMA (entries 1, 2 and 4 of Table 1). First, the rate of activation by the CuI complex (
where, [RX], [M], [CuI/L] and [Br-CuII/L] correspond to the concentration of the alkyl bromide initiator, monomer, CuI complex and CuII complex, respectively.
On the other hand, the activation rate of alkyl bromides by ht-PLPPAN (
where,
Details of the calculation, including the scaling of kinetic parameters, are included in the Supplementary Material (Supplementary Tables 2–4) (Fantin et al., 2015; Fantin et al., 2017). Specifically,
As shown in Table 3, the calculated

TABLE 3. Calculated reaction parameters during light-mediated ATRP of OEGMA catalyzed by CuBr2/TPMA. Total concentrations for Cu species were 0.054 mM (entry 1, Table 1) and 0.27 mM (entry 2, Table 1), respectively. The concentration of HO-EBiB was 2.7 mM in both entries.
Conclusions and Perspectives
In conclusion, a dispersible PAN-derived photo-cocatalyst, ht-PLPPAN, was applied as OPCs in Cu-catalyzed ATRP under blue or green light irradiation. Hydrophilic and hydrophobic functional groups prompted ht-PLPPAN to assemble into spherical OPNOs in water. Compared to previously reported photoinduced CRPs using self-assembled photocatalysts, this work expanded the utilization of OPNO-based photocatalysts or photo-cocatalysts from organic solvents (DMF/DMSO, anisole) to aqueous media. Well-controlled polymerization of acrylate and methacrylate monomers were reported. Furthermore, analysis of the R-Br activation demonstrated how the irradiation wavelength affected the activation mechanism of Cu-catalyzed ATRP. Control experiments showed that both ht-PLPPAN and the CuI complex could activate R-Br under blue light irradiation. While under green light irradiation, the CuI complex was the only activator for R-Br. But the reaction between the CuI complex and R-Br was five to six orders of magnitude faster than the activation of R-Br by ht-PLPPAN, due to the high activation rate constant of the CuI complex, particularly in aqueous media.
Data Availability Statement
The original contributions presented in the study are included in the article/Supplementary Material, further inquiries can be directed to the corresponding author.
Author Contributions
MS designed and performed the experiments. FL designed and performed the voltammetric analysis. RY prepared the polymer substrate. MS, FL, SD-S, TK and KM discussed the photocatalytic mechanism. TK performed the DFT calculations. All authors contributed to the preparation and revision of the manuscript.
Funding
Financial support from the Department of Energy (ER45998) is greatly appreciated.
Conflict of Interest
The authors declare that the research was conducted in the absence of any commercial or financial relationships that could be construed as a potential conflict of interest.
The handling Editor declared a past co-authorship with one of the authors KM.
Publisher’s Note
All claims expressed in this article are solely those of the authors and do not necessarily represent those of their affiliated organizations, or those of the publisher, the editors and the reviewers. Any product that may be evaluated in this article, or claim that may be made by its manufacturer, is not guaranteed or endorsed by the publisher.
Acknowledgments
The authors acknowledge use of the Materials Characterization Facility at Carnegie Mellon University supported by grant MCF-677785.
Supplementary Material
The Supplementary Material for this article can be found online at: https://www.frontiersin.org/articles/10.3389/fchem.2021.734076/full#supplementary-material
References
Allegrezza, M. L., and Konkolewicz, D. (2021). PET-RAFT Polymerization: Mechanistic Perspectives for Future Materials. ACS Macro Lett. 10, 433–446. doi:10.1021/acsmacrolett.1c00046
Cao, S., Le, A. N., Chen, A., and Zhong, M. (2020). Scalable Synthesis of Fluorescent Organic Nanodots by Block Copolymer Templating. J. Polym. Sci. 58, 30–34. doi:10.1002/pola.2946610.1002/pol.20190265
Chai, J.-D., and Head-Gordon, M. (2008). Long-range Corrected Hybrid Density Functionals with Damped Atom-Atom Dispersion Corrections. Phys. Chem. Chem. Phys. 10, 6615–6620. doi:10.1039/B810189B
Chen, M., Zhong, M., and Johnson, J. A. (2016). Light-Controlled Radical Polymerization: Mechanisms, Methods, and Applications. Chem. Rev. 116, 10167–10211. doi:10.1021/acs.chemrev.5b00671
Chen, T.-H., and Tseng, W.-L. (2017). Self-Assembly of Monodisperse Carbon Dots into High-Brightness Nanoaggregates for Cellular Uptake Imaging and Iron(III) Sensing. Anal. Chem. 89, 11348–11356. doi:10.1021/acs.analchem.7b02193
Chmielarz, P., Fantin, M., Park, S., Isse, A. A., Gennaro, A., Magenau, A. J. D., et al. (2017). Electrochemically Mediated Atom Transfer Radical Polymerization (eATRP). Prog. Polym. Sci. 69, 47–78. doi:10.1016/j.progpolymsci.2017.02.005
Corrigan, N., Jung, K., Moad, G., Hawker, C. J., Matyjaszewski, K., and Boyer, C. (2020). Reversible-deactivation Radical Polymerization (Controlled/living Radical Polymerization): From Discovery to Materials Design and Applications. Prog. Polym. Sci. 111, 101311. doi:10.1016/j.progpolymsci.2020.101311
Dadashi-Silab, S., Atilla Tasdelen, M., and Yagci, Y. (2014). Photoinitiated Atom Transfer Radical Polymerization: Current Status and Future Perspectives. J. Polym. Sci. Part. A: Polym. Chem. 52, 2878–2888. doi:10.1002/pola.27327
Dadashi-Silab, S., Doran, S., and Yagci, Y. (2016). Photoinduced Electron Transfer Reactions for Macromolecular Syntheses. Chem. Rev. 116, 10212–10275. doi:10.1021/acs.chemrev.5b00586
Dadashi-Silab, S., Lorandi, F., DiTucci, M. J., Sun, M., Szczepaniak, G., Liu, T., et al. (2021). Conjugated Cross-Linked Phenothiazines as Green or Red Light Heterogeneous Photocatalysts for Copper-Catalyzed Atom Transfer Radical Polymerization J. Am. Chem. Soc. 143, 9630–9638. doi:10.1021/jacs.1c04428
El Achi, N., Bakkour, Y., Adhami, W., Molina, J., Penhoat, M., Azaroual, N., et al. (2020). Metal-Free ATRP Catalyzed by Visible Light in Continuous Flow. Front. Chem. 8, 740. doi:10.3389/fchem.2020.00740
Ermakov, I. V., Rebrov, A. I., Litmanovich, A. D., and Platé, N. A. (2000). Alkaline Hydrolysis of Polyacrylonitrile, 1. Structure of the Reaction Products. Macromol. Chem. Phys. 201, 1415–1418. doi:10.1002/1521-3935(20000801)201:13<1415::AID-MACP1415>3.0.CO;2-R
Fantin, M., Isse, A. A., Gennaro, A., and Matyjaszewski, K. (2015). Understanding the Fundamentals of Aqueous ATRP and Defining Conditions for Better Control. Macromolecules 48, 6862–6875. doi:10.1021/acs.macromol.5b01454
Fantin, M., Isse, A. A., Matyjaszewski, K., and Gennaro, A. (2017). ATRP in Water: Kinetic Analysis of Active and Super-active Catalysts for Enhanced Polymerization Control. Macromolecules 50, 2696–2705. doi:10.1021/acs.macromol.7b00246
Frisch, M. J., Trucks, G. W., Schlegel, H. B., Scuseria, G. E., Robb, M. A., Cheeseman, J. R., et al. (2016). Gaussian 09. Wallingford, CT: Rev. A.02.
Fu, L., Simakova, A., Fantin, M., Wang, Y., and Matyjaszewski, K. (2018). Direct ATRP of Methacrylic Acid with Iron-Porphyrin Based Catalysts. ACS Macro Lett. 7, 26–30. doi:10.1021/acsmacrolett.7b00909
Go, D., Jurásková, A., Hoffmann, A., Kapiti, G., and Kuehne, A. J. C. (2017). Deep-Blue Fluorescent Particles via Microwave Heating of Polyacrylonitrile Dispersions. Macromol. Rapid Commun. 38, 1600775. doi:10.1002/marc.201600775
Gottlieb, E., Matyjaszewski, K., and Kowalewski, T. (2019). Polymer‐Based Synthetic Routes to Carbon‐Based Metal‐Free Catalysts. Adv. Mater. 31, 1804626. doi:10.1002/adma.201804626
Han, M., Zhu, S., Lu, S., Song, Y., Feng, T., Tao, S., et al. (2018). Recent Progress on the Photocatalysis of Carbon Dots: Classification, Mechanism and Applications. Nano Today 19, 201–218. doi:10.1016/j.nantod.2018.02.008
Hao, Q., Qiao, L., Shi, G., He, Y., Cui, Z., Fu, P., et al. (2021). Effect of Nitrogen Type on Carbon Dot Photocatalysts for Visible-Light-Induced Atom Transfer Radical Polymerization. Polym. Chem. 12, 3060–3066. doi:10.1039/D1PY00148E
Jang, J., Bae, J., and Park, E. (2006). Polyacrylonitrile Nanofibers: Formation Mechanism and Applications as a Photoluminescent Material and Carbon-Nanofiber Precursor. Adv. Funct. Mater. 16, 1400–1406. doi:10.1002/adfm.200500598
Jia, Q., Ge, J., Liu, W., Guo, L., Zheng, X., Chen, S., et al. (2017). Self‐Assembled Carbon Dot Nanosphere: A Robust, Near‐Infrared Light‐Responsive, and Vein Injectable Photosensitizer. Adv. Healthc. Mater. 6, 1601419. doi:10.1002/adhm.201601419
Jiang, J., Ye, G., Wang, Z., Lu, Y., Chen, J., and Matyjaszewski, K. (2018). Heteroatom‐Doped Carbon Dots (CDs) as a Class of Metal‐Free Photocatalysts for PET‐RAFT Polymerization under Visible Light and Sunlight. Angew. Chem. Int. Ed. 57, 12037–12042. doi:10.1002/anie.201807385
Konkolewicz, D., Krys, P., Góis, J. R., Mendonça, P. V., Zhong, M., Wang, Y., et al. (2014). Aqueous RDRP in the Presence of Cu0: The Exceptional Activity of CuI Confirms the SARA ATRP Mechanism. Macromolecules 47, 560–570. doi:10.1021/ma4022983
Konkolewicz, D., Schröder, K., Buback, J., Bernhard, S., and Matyjaszewski, K. (2012). Visible Light and Sunlight Photoinduced ATRP with Ppm of Cu Catalyst. ACS Macro Lett. 1, 1219–1223. doi:10.1021/mz300457e
Konkolewicz, D., Wang, Y., Krys, P., Zhong, M., Isse, A. A., Gennaro, A., et al. (2014). SARA ATRP or SET-LRP. End of Controversy? Polym. Chem. 5, 4409–4417. doi:10.1039/C4PY00149D
Konkolewicz, D., Wang, Y., Zhong, M., Krys, P., Isse, A. A., Gennaro, A., et al. (2013). Reversible-Deactivation Radical Polymerization in the Presence of Metallic Copper. A Critical Assessment of the SARA ATRP and SET-LRP Mechanisms. Macromolecules 46, 8749–8772. doi:10.1021/ma401243k
Kopeć, M., Lamson, M., Yuan, R., Tang, C., Kruk, M., Zhong, M., et al. (2019). Polyacrylonitrile-derived Nanostructured Carbon Materials. Prog. Polym. Sci. 92, 89–134. doi:10.1016/j.progpolymsci.2019.02.003
Kopeć, M., Pikiel, M., and Vancso, G. J. (2020). Surface-grafted Polyacrylonitrile Brushes with Aggregation-Induced Emission Properties. Polym. Chem. 11, 669–674. doi:10.1039/C9PY01213C
Kopeć, M., Yuan, R., Gottlieb, E., Abreu, C. M. R., Song, Y., Wang, Z., et al. (2017). Polyacrylonitrile-b-poly(butyl Acrylate) Block Copolymers as Precursors to Mesoporous Nitrogen-Doped Carbons: Synthesis and Nanostructure. Macromolecules 50, 2759–2767. doi:10.1021/acs.macromol.6b02678
Krys, P., and Matyjaszewski, K. (2017). Kinetics of Atom Transfer Radical Polymerization. Eur. Polym. J. 89, 482–523. doi:10.1016/j.eurpolymj.2017.02.034
Krys, P., Ribelli, T. G., Matyjaszewski, K., and Gennaro, A. (2016). Relation between Overall Rate of ATRP and Rates of Activation of Dormant Species. Macromolecules 49, 2467–2476. doi:10.1021/acs.macromol.6b00058
Kutahya, C., Aykac, F. S., Yilmaz, G., and Yagci, Y. (2016). LED and Visible Light-Induced Metal Free ATRP Using Reducible Dyes in the Presence of Amines. Polym. Chem. 7, 6094–6098. doi:10.1039/C6PY01417H
Kütahya, C., Wang, P., Li, S., Liu, S., Li, J., Chen, Z., et al. (2020). Carbon Dots as a Promising Green Photocatalyst for Free Radical and ATRP‐Based Radical Photopolymerization with Blue LEDs. Angew. Chem. Int. Ed. 59, 3166–3171. doi:10.1002/anie.201912343
Kütahya, C., Zhai, Y., Li, S., Liu, S., Li, J., Strehmel, V., et al. (2021). Distinct Sustainable Carbon Nanodots Enable Free Radical Photopolymerization, Photo‐ATRP and Photo‐CuAAC Chemistry. Angew. Chem. Int. Ed. 60, 10983–10991. doi:10.1002/anie.202015677
Lamson, M., Kopeć, M., Ding, H., Zhong, M., and Matyjaszewski, K. (2016). Synthesis of Well-Defined Polyacrylonitrile by ICARATRP with Low Concentrations of Catalyst. J. Polym. Sci. Part. A: Polym. Chem. 54, 1961–1968. doi:10.1002/pola.28055
Lorandi, F., and Matyjaszewski, K. (2020). Why Do We Need More Active ATRP Catalysts?. Isr. J. Chem. 60, 108–123. doi:10.1002/ijch.201900079
Matyjaszewski, K. (2018). Advanced Materials by Atom Transfer Radical Polymerization. Adv. Mater. 30, 1706441. doi:10.1002/adma.201706441
Matyjaszewski, K. (2012). Atom Transfer Radical Polymerization (ATRP): Current Status and Future Perspectives. Macromolecules 45, 4015–4039. doi:10.1021/ma3001719
Matyjaszewski, K., and Tsarevsky, N. V. (2014). Macromolecular Engineering by Atom Transfer Radical Polymerization. J. Am. Chem. Soc. 136, 6513–6533. doi:10.1021/ja408069v
Matyjaszewski, K., and Xia, J. (2001). Atom Transfer Radical Polymerization. Chem. Rev. 101, 2921–2990. doi:10.1021/cr940534g
Melchionna, M., and Fornasiero, P. (2020). Updates on the Roadmap for Photocatalysis. ACS Catal. 10, 5493–5501. doi:10.1021/acscatal.0c01204
Mendonça, P. V., Averick, S. E., Konkolewicz, D., Serra, A. C., Popov, A. V., Guliashvili, T., et al. (2014). Straightforward ARGET ATRP for the Synthesis of Primary Amine Polymethacrylate with Improved Chain-End Functionality under Mild Reaction Conditions. Macromolecules 47, 4615–4621. doi:10.1021/ma501007j
Miertuš, S. S., and Tomasi, J. (1981). Electrostatic Interaction of a Solute with a Continuum. A Direct Utilizaion of Ab Initio Molecular Potentials for the Prevision of Solvent Effects. Chem. Phys. 55, 117–129. doi:10.1016/0301-0104(81)85090-2
Mohapatra, H., Kleiman, M., and Esser-Kahn, A. P. (2017). Mechanically Controlled Radical Polymerization Initiated by Ultrasound. Nat. Chem 9, 135–139. doi:10.1038/nchem.2633
Pan, X., Fang, C., Fantin, M., Malhotra, N., So, W. Y., Peteanu, L. A., et al. (2016). Mechanism of Photoinduced Metal-free Atom Transfer Radical Polymerization: Experimental and Computational Studies. J. Am. Chem. Soc. 138, 2411–2425. doi:10.1021/jacs.5b13455
Pan, X., Fantin, M., Yuan, F., and Matyjaszewski, K. (2018). Externally Controlled Atom Transfer Radical Polymerization. Chem. Soc. Rev. 47, 5457–5490. doi:10.1039/C8CS00259B
Pan, X., Malhotra, N., Simakova, A., Wang, Z., Konkolewicz, D., and Matyjaszewski, K. (2015). Photoinduced Atom Transfer Radical Polymerization with Ppm-Level Cu Catalyst by Visible Light in Aqueous Media. J. Am. Chem. Soc. 137, 15430–15433. doi:10.1021/jacs.5b11599
Pan, X., Tasdelen, M. A., Laun, J., Junkers, T., Yagci, Y., and Matyjaszewski, K. (2016). Photomediated Controlled Radical Polymerization. Prog. Polym. Sci. 62, 73–125. doi:10.1016/j.progpolymsci.2016.06.005
Parkatzidis, K., Wang, H. S., Truong, N. P., and Anastasaki, A. (2020). Recent Developments and Future Challenges in Controlled Radical Polymerization: A 2020 Update. Chem. 6, 1575–1588. doi:10.1016/j.chempr.2020.06.014
Pearson, R. M., Lim, C.-H., McCarthy, B. G., Musgrave, C. B., and Miyake, G. M. (2016). Organocatalyzed Atom Transfer Radical Polymerization Using N-Aryl Phenoxazines as Photoredox Catalysts. J. Am. Chem. Soc. 138, 11399–11407. doi:10.1021/jacs.6b08068
Perrier, S. (2017). 50th Anniversary Perspective: RAFT Polymerization-A User Guide. Macromolecules 50, 7433–7447. doi:10.1021/acs.macromol.7b00767
Ribelli, T. G., Lorandi, F., Fantin, M., and Matyjaszewski, K. (2019). Atom Transfer Radical Polymerization: Billion Times More Active Catalysts and New Initiation Systems. Macromol. Rapid Commun. 40, 1800616. doi:10.1002/marc.201800616
Romero, N. A., and Nicewicz, D. A. (2016). Organic Photoredox Catalysis. Chem. Rev. 116, 10075–10166. doi:10.1021/acs.chemrev.6b00057
Simakova, A., Averick, S. E., Konkolewicz, D., and Matyjaszewski, K. (2012). Aqueous ARGET ATRP. Macromolecules 45, 6371–6379. doi:10.1021/ma301303b
Smolne, S., Weber, S., and Buback, M. (2016). Propagation and Termination Kinetics of Poly(Ethylene Glycol) Methyl Ether Methacrylate in Aqueous Solution. Macromol. Chem. Phys. 217, 2391–2401. doi:10.1002/macp.201600302
Stephens, P. J., Devlin, F. J., Chabalowski, C. F., and Frisch, M. J. (1994). Ab Initio Calculation of Vibrational Absorption and Circular Dichroism Spectra Using Density Functional Force Fields. J. Phys. Chem. 98, 11623–11627. doi:10.1021/j100096a001
Sun, M., Gottlieb, E., Yuan, R., Ghosh, S., Wang, H., Selhorst, R., et al. (2020). Polyene-Free Photoluminescent Polymers via Hydrothermal Hydrolysis of Polyacrylonitrile in Neutral Water. ACS Macro Lett. 9, 1403–1408. doi:10.1021/acsmacrolett.0c00410
Sun, Y.-P., Zhou, B., Lin, Y., Wang, W., Fernando, K. A. S., Pathak, P., et al. (2006). Quantum-sized Carbon Dots for Bright and Colorful Photoluminescence. J. Am. Chem. Soc. 128, 7756–7757. doi:10.1021/ja062677d
Szczepaniak, G., Łagodzińska, M., Dadashi-Silab, S., Gorczyński, A., and Matyjaszewski, K. (2020). Fully Oxygen-Tolerant Atom Transfer Radical Polymerization Triggered by Sodium Pyruvate. Chem. Sci. 11, 8809–8816. doi:10.1039/D0SC03179H
Tang, C., Tracz, A., Kruk, M., Zhang, R., Smilgies, D.-M., Matyjaszewski, K., et al. (2005). Long-range Ordered Thin Films of Block Copolymers Prepared by Zone-Casting and Their thermal Conversion into Ordered Nanostructured Carbon. J. Am. Chem. Soc. 127, 6918–6919. doi:10.1021/ja0508929
Tang, W., Kwak, Y., Braunecker, W., Tsarevsky, N. V., Coote, M. L., and Matyjaszewski, K. (2008). Understanding Atom Transfer Radical Polymerization: Effect of Ligand and Initiator Structures on the Equilibrium Constants. J. Am. Chem. Soc. 130, 10702–10713. doi:10.1021/ja802290a
Tao, S., Feng, T., Zheng, C., Zhu, S., and Yang, B. (2019). Carbonized Polymer Dots: A Brand New Perspective to Recognize Luminescent Carbon-Based Nanomaterials. J. Phys. Chem. Lett. 10, 5182–5188. doi:10.1021/acs.jpclett.9b01384
Theriot, J. C., Lim, C.-H., Yang, H., Ryan, M. D., Musgrave, C. B., and Miyake, G. M. (2016). Organocatalyzed Atom Transfer Radical Polymerization Driven by Visible Light. Science 352, 1082–1086. doi:10.1126/science.aaf3935
Treat, N. J., Sprafke, H., Kramer, J. W., Clark, P. G., Barton, B. E., Read de Alaniz, J., et al. (2014). Metal-Free Atom Transfer Radical Polymerization. J. Am. Chem. Soc. 136, 16096–16101. doi:10.1021/ja510389m
Wang, J.-S., and Matyjaszewski, K. (1995). Controlled/"living" Radical Polymerization. Atom Transfer Radical Polymerization in the Presence of Transition-Metal Complexes. J. Am. Chem. Soc. 117, 5614–5615. doi:10.1021/ja00125a035
Wang, Z., Pan, X., Li, L., Fantin, M., Yan, J., Wang, Z., et al. (2017). Enhancing Mechanically Induced ATRP by Promoting Interfacial Electron Transfer from Piezoelectric Nanoparticles to Cu Catalysts. Macromolecules 50, 7940–7948. doi:10.1021/acs.macromol.7b01597
Wu, C., Corrigan, N., Lim, C.-H., Jung, K., Zhu, J., Miyake, G., et al. (2019). Guiding the Design of Organic Photocatalyst for PET-RAFT Polymerization: Halogenated Xanthene Dyes. Macromolecules 52, 236–248. doi:10.1021/acs.macromol.8b02517
Xia, C., Zhu, S., Feng, T., Yang, M., and Yang, B. (2019). Evolution and Synthesis of Carbon Dots: From Carbon Dots to Carbonized Polymer Dots. Adv. Sci. 6, 1901316. doi:10.1002/advs.201901316
Xia, J., and Matyjaszewski, K. (1999). Controlled/"Living" Radical Polymerization. Atom Transfer Radical Polymerization Catalyzed by Copper(I) and Picolylamine Complexes. Macromolecules 32, 2434–2437. doi:10.1021/ma981694n
Xu, J., Shanmugam, S., Duong, H. T., and Boyer, C. (2015). Organo-photocatalysts for Photoinduced Electron Transfer-Reversible Addition-Fragmentation Chain Transfer (PET-RAFT) Polymerization. Polym. Chem. 6, 5615–5624. doi:10.1039/C4PY01317D
Yuan, R., Wang, H., Sun, M., Damodaran, K., Gottlieb, E., Kopeć, M., et al. (2019). Well-Defined N/S Co-doped Nanocarbons from Sulfurized PAN-B-PBA Block Copolymers: Structure and Supercapacitor Performance. ACS Appl. Nano Mater. 2, 2467–2474. doi:10.1021/acsanm.9b00340
Yuan, R., Wang, H., Sun, M., Whitacre, J., Matyjaszewski, K., and Kowalewski, T. (2020). Copolymer‐Derived N/B Co‐Doped Nanocarbons with Controlled Porosity and Highly Active Surface. J. Polym. Sci. 58, 225–232. doi:10.1002/pol.20190002
Zhang, Y., Wang, Y., and Matyjaszewski, K. (2011). ATRP of Methyl Acrylate with Metallic Zinc, Magnesium, and Iron as Reducing Agents and Supplemental Activators. Macromolecules 44, 683–685. doi:10.1021/ma102492c
Zhang, Z., Yi, G., Li, P., Zhang, X., Fan, H., Zhang, Y., et al. (2020). A Minireview on Doped Carbon Dots for Photocatalytic and Electrocatalytic Applications. Nanoscale 12, 13899–13906. doi:10.1039/D0NR03163A
Zhao, P., Wang, L., Wu, Y., Yang, T., Ding, Y., Yang, H. G., et al. (2019). Hyperbranched Conjugated Polymer Dots: The Enhanced Photocatalytic Activity for Visible Light-Driven Hydrogen Production. Macromolecules 52, 4376–4384. doi:10.1021/acs.macromol.9b00551
Zhong, M., Kim, E. K., McGann, J. P., Chun, S.-E., Whitacre, J. F., Jaroniec, M., et al. (2012). Electrochemically Active Nitrogen-Enriched Nanocarbons with Well-Defined Morphology Synthesized by Pyrolysis of Self-Assembled Block Copolymer. J. Am. Chem. Soc. 134, 14846–14857. doi:10.1021/ja304352n
Zhou, Q., Cao, B., Zhu, C., Xu, S., Gong, Y., Yuan, W. Z., et al. (2016). Clustering-Triggered Emission of Nonconjugated Polyacrylonitrile. Small 12, 6586–6592. doi:10.1002/smll.201601545
Zhu, S., Meng, Q., Wang, L., Zhang, J., Song, Y., Jin, H., et al. (2013). Highly Photoluminescent Carbon Dots for Multicolor Patterning, Sensors, and Bioimaging. Angew. Chem. Int. Ed. 52, 3953–3957. doi:10.1002/anie.201300519
Zhu, S., Song, Y., Shao, J., Zhao, X., and Yang, B. (2015). Non‐Conjugated Polymer Dots with Crosslink‐Enhanced Emission in the Absence of Fluorophore Units. Angew. Chem. Int. Ed. 54, 14626–14637. doi:10.1002/anie.201504951
Zhu, S., Song, Y., Zhao, X., Shao, J., Zhang, J., and Yang, B. (2015). The Photoluminescence Mechanism in Carbon Dots (Graphene Quantum Dots, Carbon Nanodots, and Polymer Dots): Current State and Future Perspective. Nano Res. 8, 355–381. doi:10.1007/s12274-014-0644-3
Keywords: ATRP, photocatalyst, polyacrylonitrile, carbon dot, luminescence, self-assembly
Citation: Sun M, Lorandi F, Yuan R, Dadashi-Silab S, Kowalewski T and Matyjaszewski K (2021) Assemblies of Polyacrylonitrile-Derived Photoactive Polymers as Blue and Green Light Photo-Cocatalysts for Cu-Catalyzed ATRP in Water and Organic Solvents. Front. Chem. 9:734076. doi: 10.3389/fchem.2021.734076
Received: 30 June 2021; Accepted: 04 August 2021;
Published: 13 August 2021.
Edited by:
Joanna Pietrasik, Lodz University of Technology, PolandReviewed by:
Yusuf Yagci, Istanbul Technical University, TurkeyAjaya Bhattarai, Tribhuvan University, Nepal
Copyright © 2021 Sun, Lorandi, Yuan, Dadashi-Silab, Kowalewski and Matyjaszewski. This is an open-access article distributed under the terms of the Creative Commons Attribution License (CC BY). The use, distribution or reproduction in other forums is permitted, provided the original author(s) and the copyright owner(s) are credited and that the original publication in this journal is cited, in accordance with accepted academic practice. No use, distribution or reproduction is permitted which does not comply with these terms.
*Correspondence: Krzysztof Matyjaszewski, bWF0eWphc3pld3NraUBjbXUuZWR1