- 1Department of Health Sciences, University of Magna Graecia Catanzaro, Catanzaro, Italy
- 2Department of Pharmacy, Health and Nutritional Sciences, University of Calabria, Rende, Italy
- 3Galascreen Laboratories, University of Calabria, Rende, Italy
- 4Research Division, Dynamical Business & Science Society – DBSS International SAS, Bogotá, Colombia
- 5Grupo de Investigación NUTRAL, Facultad de Ciencias de la Nutrición y los Alimentos, Universidad CES, Medellín, Colombia
- 6Hologenomiks Research Group, Department of Genetics, Physical Anthropology and Animal Physiology, University of the Basque Country (UPV/EHU), Leioa, Spain
Cognition is a mental process of understanding and learning driven by memory. Recent advances in molecular biology and neuroscience have revealed a fascinating interplay between cognitive function and microRNAs (miRNAs). The ketogenic diet (KD) is a low-carbohydrate, high-fat, and adequate-protein diet that triggers the synthesis of ketone bodies, establishing ketosis. Recent and accumulating studies on human and animal models have shown that the KD benefits neurodegenerative diseases, where cognition is affected. The KD can also modulate miRNAs, molecules that are dysregulated in the brains of individuals with Alzheimer’s disease, where cognition is lost. In this mini-review, we provide an overview of the function of miRNAs in neurodevelopment and cognition. We also explore how the KD in human studies can enhance cognitive function and highlight the protective role of microRNAs in neurological conditions.
Introduction
Cognitive function refers to the mental processes involved in acquiring knowledge, reasoning, and manipulating information. It includes the domains of language abilities, perception, memory, learning, decision-making, and attention (1). Conventional models of human cognition have been hypothesized by cognitive scientists within an information-processing paradigm. The mechanisms underlying cognitive processes remain an active area of research. One growing focus is the role of micronutrients (2, 3) and/or a dietary regimen known as the KD (4) in regulating cognitive function, with microRNAs (miRNAs) emerging as key contributors (5). miRNAs are short, non-coding RNA molecules that play a significant role in post-transcriptional gene regulation in several organs and tissues. They are also used as diagnostic tools in some conditions (6, 7). In addition, Orellana et al. proposed miRNAs as potential biomarkers in neurodegenerative disease, identifying four miRNAs with variable expression in patients with Alzheimer’s disease (AD) and frontotemporal dementia (8). The regulatory role of miRNAs has profound implications for cognitive function, as numerous miRNAs are expressed in the brain and actively participate in various neurological processes (9) and cognitive performance (10). In this mini-review, we provide an overview of the role of miRNAs in sustaining neurodevelopment and cognition functions. We also discuss how the KD affects neurological conditions by modulating miRNAs, its potential benefits in AD, and which conserved miRNAs were found to be linked to this condition.
miRNAs sustain neurodevelopment and cognitive functions
MiRNAs play an important role in synaptic function and neurotransmission (11). The impact of miRNAs on cognitive function begins during the development of cortical neurons, oligodendrocytes, and astroglia (12). These small regulators are essential for the formation and maintenance of neural circuits. miR-124 is the most abundant miRNA in the brain and is known to promote neuronal differentiation and axon growth (13), while miR-9, a neuronal-specific miRNA, is highly expressed in the brain (14), where it controls neural stem cell numbers (15). miR-132 regulates neuronal differentiation and maturation and participates in axon growth, neural migration, and plasticity (16). On the other hand, miR-219 is crucial for the coordinated transition of oligodendrocyte progenitor cells to oligodendrocytes and subsequent myelin formation (17). Although miR-138 expression is also elevated in oligodendrocytes (17), miR-199a-5p and miR-145 play a critical role in their maturation (18).
Synaptic plasticity (SP) is crucial for cognitive function, serving as the foundation for integrated neuronal communication (19). SP is at the core of cognitive function, which includes long-term potentiation and long-term depression, with underlying biochemical mechanisms that support learning and memory (20). miR-134 has been found to play a critical role in modulating SP. For instance, miR-134 inhibits the translation of Lim kinase 1 (LIMK1), which is involved in actin polymerization and dendritic morphology (21). Disruption of miR-134 leads to dysregulation of synaptic plasticity and impaired learning and memory (22). Meanwhile, miR-34a (23) and miR-34c (24) mediate synaptic and memory deficits. The miR-29 family is differentially regulated in the adult hippocampus during learning (25). In addition, miR-466f-3p appears to positively regulate neuronal plasticity by influencing cAMP response element-binding protein (CREB) activity during spatial learning and memory formation in mice (26). Furthermore, studies on miRNAs in brain tissue have shown that circulating miRNAs are also linked to cognitive function (27, 28). miR-212 and miR-484 are essential for synaptic function and neurotransmission (29, 30). Lower expression of miR-484 and miR-197-3p has been associated with accelerated cognitive decline, with downregulation of miR-484 specifically linked to an increased risk of AD development (30). Some studies have found correlations between specific circulating miRNAs and cognitive performance. For instance, Junyi Ma et al. found an association between miR-330-3p in serum and executive function (27). MiR-181a-5p, miR-148-3p, and miR-146a-5p showed a significant negative correlation with cognitive function and similar beta-amyloid protein (Aβ) 42/40 ratio values, suggesting their potential as biomarkers for AD (31). In addition, Aβ can inhibit the expression of miR-15a, thereby inducing the expression of Bag5 and activating the protective mechanism of Bag5 against Aβ-induced apoptosis (32). Moreover, miR-206 and miR-132 were positively correlated with the Montreal Cognitive Assessment (MoCA) score in patients with mild cognitive impairment (MCI). Circulating miR-206 and miR-132, which are upregulated in patients with MCI, are proposed as potential biomarkers for MCI diagnosis (28). Notably, of the 17 miRNAs reported here, 11—miR-9, miR-29, miR-34a, miR-132, miR-138, miR-145, miR-148, miRNA-181a-5p, miR-199a-5p, miR-206, and miR-219—are highly conserved across species. This characteristic often enhances the value of basic research from a translational perspective, particularly in AD, where understanding both pathogenesis and treatment-specific health outcomes is crucial (left panel in Figure 1).
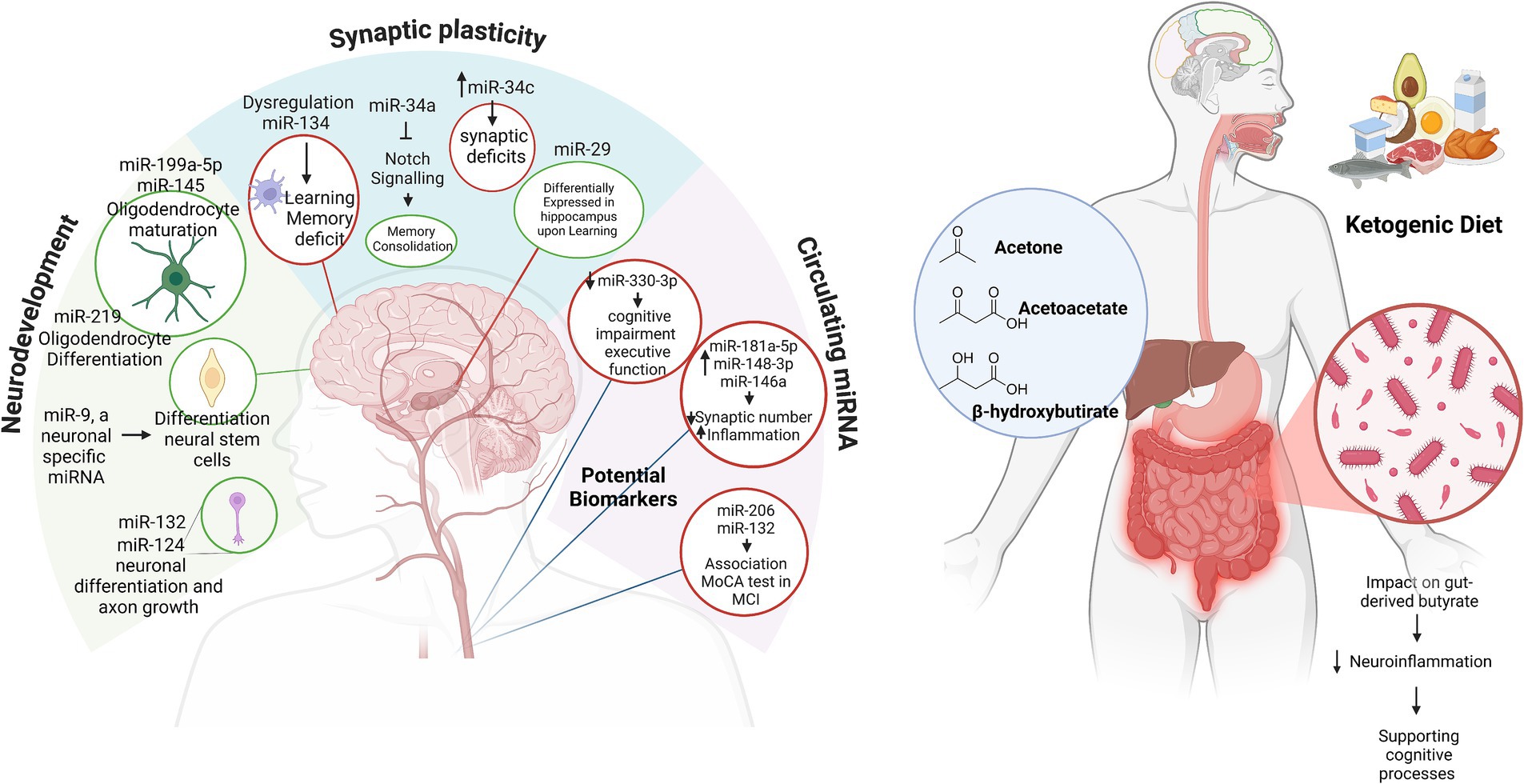
Figure 1. miRNAs in neurodevelopment and cognitive function (on the left). The relationship between miRNAs and their roles in neurodevelopment, synaptic plasticity, and circulating biomarkers in cognitive function and neurological health. Green circles highlight miRNAs associated with positive effects, while red circles represent miRNAs linked to negative effects. MCI, mild cognitive impairment; MoCA, Montreal Cognitive Assessment. The ketogenic diet sustains cognition (on the right). In the blue circle, the ketone bodies produced during the KD; in the dark red circle, the effects of the KD on the microbiome’s synthesis of butyrate. Created in BioRender. Created with BioRender.com.
Biochemical pathways in ketogenesis
The biochemistry of ketone body synthesis begins during fasting. Cellular oxaloacetate levels are insufficient to condense with acetyl-CoA for citrate formation, leading to a delay in the citric acid cycle. The essential biochemical process for the KD occurs primarily in the mitochondria of liver cells and follows these biosynthetic steps: (i) condensation of two molecules of acetyl-CoA to form acetoacetyl-CoA; the reaction is catalyzed by the enzyme 3-ketothiolase. (ii) The acetoacetyl-CoA reacts with another acetyl-CoA in the presence of a water molecule, forming 3-hydroxy-3-methylglutaryl-CoA (HMG-CoA) and free CoA, with the reaction catalyzed by HMG-CoA synthase. It is worth noting that this critical step is facilitated by thioester bond hydrolysis, which offsets the unfavorable formation of acetoacetyl-CoA. HMG-CoA lyase cleaves 3-hydroxy-3-methylglutaryl-CoA into acetyl-CoA and acetoacetate, the first ketone body. Acetoacetate, a β-ketoacid, has two fates: spontaneous decarboxylation to acetone (the second ketone body) or reduction by NADH-dependent 3-hydroxybutyrate dehydrogenase to form 3-hydroxybutyrate (BHB), the third ketone body (33). Ketosis is elicited not only during fasting but can also occur when minimal amounts of carbohydrates are consumed. For instance, 3 days of consuming 20–30 g of carbohydrates or limiting carbohydrate intake to less than 5% of total daily calories can induce ketosis. The use of this nutritional scheme dates back to over 100 years when it was adopted to manage a form of drug-resistant childhood epilepsy. The neuroprotective effect is considered to be directly due to ketone bodies (34). The KD is a valuable nutrition strategy in the management of recurrent migraines (35). In addition to its antioxidant capabilities, the absence of glycemic peaks during ketosis also causes the production of advanced glycation end products (36, 37).
Ketogenic diet and its impact on cognitive function: evidence from human studies
Decline in cognitive function is age-dependent and could be a potential target for extending cognitive health span, in contrast to dementia, including AD (38). The prevalence of dementia worldwide affects more than 55 million people, the majority of whom have AD (39). This situation also leads to onerous burdens on society. In developed countries, the U.S. alone has 6.9 million people aged 65 and older living with AD, according to data updated in 2024 (40). Similarly, developing countries account for 5% of the overall prevalence (41). This disorder affects not only the individual patients but also their families and caregivers. Diagnosing dementia is quite challenging as the MCI test may not always provide a clear indication. Clinical trials have suggested that different types of the KD significantly improve the quality of life and daily function and eased AD-related cognitive impairment, especially in individuals without the epsilon 4 allele of the apolipoprotein E gene (APOE ɛ4). This suggests that ketone body metabolism is related to APOE ɛ4 status (37). Individuals harboring APOE ε4 appear to be at higher risk, as recently reported in a meta-analysis conducted in Italy (42), showing a transitional stage between normal brain aging and dementia. Therefore, it is crucial to find effective prevention therapies to delay the onset of disease and slow cognitive decline. Some studies have explained the mechanisms underlying the protective effect of the KD on AD. By increasing BHB, the KD compensates for the brain’s glucose hypometabolism, providing an alternative and efficient energy source (43). In addition, the KD helps clear amyloid-beta (Aβ) plaques, decrease tau hyperphosphorylation, and improve mitochondrial function. Clinical and preclinical studies have demonstrated cognitive improvements and biomarker modulation, highlighting the KD as a potential therapeutic approach for managing AD (44). It also reduces neuroinflammation by suppressing the NLRP3 inflammasome and lowering the levels of pro-inflammatory cytokines such as IL-1β and TNF-α. These findings suggest that the KD shows promise in delaying and/or mitigating cognitive decline symptoms sustained by neuroinflammation (45).
Ketogenic diet and miRNAs
The modulation of miRNAs by dietary regimens, including the ketogenic diet, is well-documented (46, 47). Micronutrients influence miRNA synthesis epigenetically, with recent evidence suggesting a role for exogenous food-derived miRNAs (48). BHB acts as a signaling molecule, regulating miRNA expression and other epigenetic processes (49). The KD modulates miRNAs that regulate genes involved in metabolic and inflammatory pathways, with normalization of antioxidant and anti-inflammatory miRNAs in obese participants post-diet, suggesting an epigenetic role (37, 50). In fact, miR-34a downregulates SIRT1, a hypothalamic NAD + -dependent deacetylase that regulates energy balance, and enhances mitochondrial biogenesis, fatty acid oxidation, and ketogenesis (51, 52). However, studies on miRNAs as predictors of the KD’s therapeutic effects are scarce, with the majority of them focusing on obesity, where circulating miRNA profiles may indicate the risk of complications and monitor weight loss outcomes (53).
Ketogenic diet and miRNA in neurological conditions
Emerging evidence underscores the complex interplay between miRNAs, the KD, and cognition, with brain-derived neurotrophic factor (BDNF) serving as a pivotal link. BDNF is essential for neurogenesis, synaptogenesis, and memory. It is influenced by miRNAs that regulate its activity and inflammatory pathways (54). The KD promotes neuroprotective effects by modulating miRNA expression (55) and enhancing BDNF function and synaptic activity. Furthermore, the diet’s impact on gut-derived butyrate, which is known to reduce neuroinflammation, highlights its role in supporting cognitive processes (right panel in Figure 1) (56). Recently, the KD, followed for a minimum of 6 months as a treatment for childhood epilepsy, significantly reduced the number of seizures in seven of eight pediatric patients treated with this nutritional regimen. KD-induced modifications in eleven relevant miRNAs were monitored in peripheral blood mononuclear cells (PMBCs). Seven miRNAs were found to be downregulated by the KD in these patients: miR-3978, miR-6726-3p, miR-130a-3p, miR-4758, miR-6745, miR-532, and miR-185-5p. Meanwhile, four were upregulated: miR-4538, miR-602, miR-330-5p, and miR-4673 (57). In a group of patients with pediatric autism, following 4 months of a KD, the levels of miR-134-5p and miR-132-3p were significantly reduced, while the levels of miR-125b-5p remained unchanged and the levels of miR-375-3p increased (56). Finally, our research focused on a 6-week the KD in obese women who self-reported experiencing migraine. We observed a downregulation of miR-211-5p, no changes in brain-enriched miR-382-5p and miR-342-5p, and an upregulation of miR-590-5p, miR-660-3p, miR-34a-5p and, miR-26b-5p (35, 58). Furthermore, it was observed that miRNA-34a, miRNA-132, miRNA-134, and miRNA-330 are commonly associated with neurodevelopment and cognitive functions in animal studies, with miRNA-34a being notably conserved across species.
Critical considerations of the KD
While the KD shows potential in modulating miRNAs and influencing cognitive and neurological conditions, the findings must be approached with caution. It was reported that low-carbohydrate diet regimens were associated with less confusion and faster response during an attention vigilance task, with a positive impact on cognitive behavior even when followed for 1 year (59, 60). Confounding factors must also be considered, such as individual variability due to genetic polymorphism differences, basal metabolism, and adherence to the diet, all of which significantly influence the outcomes. For instance, genetic polymorphisms in pathways related to lipid metabolism or inflammation may alter the miRNA response to the KD, leading to variable effects on cognitive and neuroprotective outcomes (61). In addition, the KD is associated with potential health risks that must not be overlooked. These include nutrient deficiencies, gastrointestinal disturbances, dyslipidemia, and, in some cases, long-term cardiovascular risks (62, 63). In fact, the low-carb pattern is considered to be more beneficial than very low-carbohydrate diets in terms of cardiovascular mortality (64). These adverse effects highlight the need for personalized approaches when considering the KD as a therapeutic intervention.
Conclusion
In conclusion, understanding the complex interplay between metabolism during the KD, miRNAs, and cognitive function is important for calibrating the KD regimen to delay neurodegenerative diseases by activating endogenous miRNAs and, consequently, multiple molecular and cellular pathways. Notably, the network of miRNAs and their influence on the KD and cognitive function is a promising area of research in neuroscience to delay the onset of AD.
Author contributions
DA-G: Visualization, Writing – original draft. EC: Writing – original draft, Writing – review & editing. MC: Conceptualization, Writing – original draft. DB: Writing – review & editing. RC: Conceptualization, Writing – review & editing.
Funding
The author(s) declare that no financial support was received for the research, authorship, and/or publication of this article.
Conflict of interest
The authors declare that the research was conducted in the absence of any commercial or financial relationships that could be construed as a potential conflict of interest.
The author(s) declared that they were an editorial board member of Frontiers, at the time of submission. This had no impact on the peer review process and the final decision.
Generative AI statement
The authors declare that no Gen AI was used in the creation of this manuscript.
Publisher’s note
All claims expressed in this article are solely those of the authors and do not necessarily represent those of their affiliated organizations, or those of the publisher, the editors and the reviewers. Any product that may be evaluated in this article, or claim that may be made by its manufacturer, is not guaranteed or endorsed by the publisher.
References
1. Robinson, M. Encyclopedia of quality of life and well-being research. Ref Rev. (2015) 29:22. doi: 10.1108/RR-06-2015-0143
2. Abrego-Guandique, DM, Bonet, ML, Caroleo, MC, Cannataro, R, Tucci, P, Ribot, J, et al. The effect of Beta-carotene on cognitive function: a systematic review. Brain Sci. (2023) 13:1468. doi: 10.3390/brainsci13101468
3. Elechi, JOG, Guandique, DMA, and Cannataro, R. Creatine in cognitive performance: a commentary. Curr Mol Pharmacol. (2024) 17:e18761429272915–5. doi: 10.2174/0118761429272915231122112748
4. Chinna-Meyyappan, A, Gomes, FA, Koning, E, Fabe, J, Breda, V, and Brietzke, E. Effects of the ketogenic diet on cognition: a systematic review. Nutr Neurosci. (2023) 26:1258–78. doi: 10.1080/1028415X.2022.2143609
5. Melas, K, Talevi, V, Imtiaz, MA, Etteldorf, R, Estrada, S, Krüger, DM, et al. Blood-derived microRNAs are related to cognitive domains in the general population. Alzheimers Dement. (2024) 20:7138–59. doi: 10.1002/alz.14197
6. Cione, E, Abrego Guandique, DM, Caroleo, MC, Luciani, F, Colosimo, M, and Cannataro, R. Liver damage and microRNAs: an update. Curr Issues Mol Biol. (2023) 45:78–91. doi: 10.3390/cimb45010006
7. Peronace, C, Cione, E, Abrego-Guandique, DM, Fazio, MD, Panduri, G, Caroleo, MC, et al. FAM19A4 and hsa-miR124-2 double methylation as screening for ASC-H- and CIN1 HPV-positive women. Pathogens. (2024) 13:312. doi: 10.3390/pathogens13040312
8. Orellana, P, Caviedes, A, Hernandez, H, Lillo, P, Villagra, R, Cerda, M, et al. Differential expression of miRNAs from circulating exosomes in Alzheimer disease and frontotemporal dementia. Alzheimers Dement. (2024) 20:20(S2). doi: 10.1002/alz.091546
9. Tsujimura, K, Shiohama, T, and Takahashi, E. microRNA biology on brain development and neuroimaging approach. Brain Sci. (2022) 12:1366. doi: 10.3390/brainsci12101366
10. Gullett, JM, Chen, Z, O’Shea, A, Akbar, M, Bian, J, Rani, A, et al. MicroRNA predicts cognitive performance in healthy older adults. Neurobiol Aging. (2020) 95:186–94. doi: 10.1016/j.neurobiolaging.2020.07.023
11. Li, YB, Fu, Q, Guo, M, Du, Y, Chen, Y, and Cheng, Y. MicroRNAs: pioneering regulators in Alzheimer’s disease pathogenesis, diagnosis, and therapy. Transl. Psychiatry. (2024) 14. doi: 10.1038/s41398-024-03075-8
12. Cho, KHT, Xu, B, Blenkiron, C, and Fraser, M. Emerging roles of miRNAs in brain development and perinatal brain injury. Front Physiol. (2019):10:227. doi: 10.3389/fphys.2019.00227
13. Makeyev, EV, Zhang, J, Carrasco, MA, and Maniatis, T. The MicroRNA miR-124 promotes neuronal differentiation by triggering brain-specific alternative pre-mRNA splicing. Mol Cell. (2007) 27:435–48. doi: 10.1016/j.molcel.2007.07.015
14. Krichevsky, AM, Sonntag, KC, Isacson, O, and Kosik, KS. Specific MicroRNAs modulate embryonic stem cell–derived neurogenesis. Stem Cells. (2006) 24:857–64. doi: 10.1634/stemcells.2005-0441
15. Roese-Koerner, B, Stappert, L, Berger, T, Braun, NC, Veltel, M, Jungverdorben, J, et al. Reciprocal regulation between bifunctional miR-9/9∗ and its transcriptional modulator notch in human neural stem cell self-renewal and differentiation. Stem Cell Reports. (2016) 7:207–19. doi: 10.1016/j.stemcr.2016.06.008
16. Qian, Y, Song, J, Ouyang, Y, Han, Q, Chen, W, Zhao, X, et al. Advances in roles of miR-132 in the nervous system. Front Pharmacol. (2017) 8:8. doi: 10.3389/fphar.2017.00770
17. Dugas, JC, Cuellar, TL, Scholze, A, Ason, B, Ibrahim, A, Emery, B, et al. Dicer1 and miR-219 are required for Normal oligodendrocyte differentiation and myelination. Neuron. (2010) 65:597–611. doi: 10.1016/j.neuron.2010.01.027
18. Letzen, BS, Liu, C, Thakor, NV, Gearhart, JD, All, AH, and Kerr, CL. MicroRNA expression profiling of oligodendrocyte differentiation from human embryonic stem cells. PLoS One. (2010) 5:e10480. doi: 10.1371/journal.pone.0010480
19. Appelbaum, LG, Shenasa, MA, Stolz, L, and Daskalakis, Z. Synaptic plasticity and mental health: methods, challenges and opportunities. Neuropsychopharmacology. (2023) 48:113–20. doi: 10.1038/s41386-022-01370-w
20. Citri, A, and Malenka, RC. Synaptic plasticity: multiple forms, functions, and mechanisms. Neuropsychopharmacology. (2008) 33:18–41. doi: 10.1038/sj.npp.1301559
21. Fan, C, Zhu, X, Song, Q, Wang, P, Liu, Z, and Yu, SY. MiR-134 modulates chronic stress-induced structural plasticity and depression-like behaviors via downregulation of Limk1/cofilin signaling in rats. Neuropharmacology. (2018) 131:364–76. doi: 10.1016/j.neuropharm.2018.01.009
22. Baby, N, Alagappan, N, Dheen, ST, and Sajikumar, S. MicroRNA-134-5p inhibition rescues long-term plasticity and synaptic tagging/capture in an Aβ(1–42)-induced model of Alzheimer’s disease. Aging Cell. (2020) 19:e13046. doi: 10.1111/acel.13046
23. Dias, BG, Goodman, JV, Ahluwalia, R, Easton, AE, Andero, R, and Ressler, KJ. Amygdala-dependent fear memory consolidation via miR-34a and notch signaling. Neuron. (2014) 83:906–18. doi: 10.1016/j.neuron.2014.07.019
24. Shi, Z, Zhang, K, Zhou, H, Jiang, L, Xie, B, Wang, R, et al. Increased miR-34c mediates synaptic deficits by targeting synaptotagmin 1 through ROS-JNK-p53 pathway in Alzheimer’s disease. Aging Cell. (2020) 19:e13125. doi: 10.1111/acel.13125
25. Kremer, EA, Gaur, N, Lee, MA, Engmann, O, Bohacek, J, and Mansuy, IM. Interplay between TETs and microRNAs in the adult brain for memory formation. Sci Rep. (2018) 8:1678. doi: 10.1038/s41598-018-19806-z
26. Asok, A, Leroy, F, Rayman, JB, and Kandel, ER. Molecular mechanisms of the memory trace. Trends Neurosci. (2019) 42:14–22. doi: 10.1016/j.tins.2018.10.005
27. Ma, J, Cao, X, Chen, F, Ye, Q, Qin, R, Cheng, Y, et al. Exosomal MicroRNAs contribute to cognitive impairment in hypertensive patients by decreasing frontal cerebrovascular reactivity. Front Neurosci. (2021) 15:15. doi: 10.3389/fnins.2021.614220
28. Xie, B, Zhou, H, Zhang, R, Song, M, Yu, L, Wang, L, et al. Serum miR-206 and miR-132 as potential circulating biomarkers for mild cognitive impairment. J Alzheimers Dis. (2015) 45:721–31. doi: 10.3233/JAD-142847
29. Remenyi, J, van, M, Palygin, O, Mistry, RB, McKenzie, C, Macdonald, A, et al. miR-132/212 knockout mice reveal roles for these miRNAs in regulating cortical synaptic transmission and plasticity. PLoS One. (2013) 8:e62509. doi: 10.1371/journal.pone.0062509
30. Wingo, TS, Yang, J, Fan, W, Min Canon, S, Gerasimov, ES, Lori, A, et al. Brain microRNAs associated with late-life depressive symptoms are also associated with cognitive trajectory and dementia. NPJ Genom Med. (2020) 5:6. doi: 10.1038/s41525-019-0113-8
31. Islam, MR, Kaurani, L, Berulava, T, Heilbronner, U, Budde, M, Centeno, TP, et al. A microRNA signature that correlates with cognition and is a target against cognitive decline. EMBO Mol Med. (2021) 13:e13659. doi: 10.15252/emmm.202013659
32. Pan, Q, Hu, X, and Guo, K. Beta-amyloid protein regulates miR-15a and activates Bag5 to influence neuronal apoptosis in Alzheimer’s disease. Zhong Nan Da Xue Xue Bao Yi Xue Ban. (2024) 49:1109–19. doi: 10.11817/j.issn.1672-7347.2024.230439
33. Puchalska, P, and Crawford, PA. Metabolic and signaling roles of ketone bodies in health and disease. Annu Rev Nutr. (2021) 41:49–77. doi: 10.1146/annurev-nutr-111120-111518
34. Dhamija, R, Eckert, S, and Wirrell, E. Ketogenic Diet. Can J Neurol Sci. (2013) 40:158–67. doi: 10.1017/S0317167100013676
35. Gallelli, L, Cione, E, Peltrone, F, Siviglia, S, Verano, A, Chirchiglia, D, et al. Hsa-miR-34a-5p and hsa-miR-375 as biomarkers for monitoring the effects of drug treatment for migraine pain in children and adolescents: a pilot study. J Clin Med. (2019) 8:928. doi: 10.3390/jcm8070928
36. Perrone, A, Giovino, A, Benny, J, and Martinelli, F. Advanced glycation end products (AGEs): biochemistry, signaling, analytical methods, and epigenetic effects. Oxidative Med Cell Longev. (2020) 2020:1–18. doi: 10.1155/2020/3818196
37. Cannataro, R, Caroleo, MC, Fazio, A, la Torre, C, Plastina, P, Gallelli, L, et al. Ketogenic diet and microRNAs linked to antioxidant biochemical homeostasis. Antioxidants (Basel). (2019) 8:269. doi: 10.3390/antiox8080269
38. Isaev, NK, Stelmashook, EV, and Genrikhs, EE. Neurogenesis and brain aging. Rev Neurosci. (2019) 30:573–80. doi: 10.1515/revneuro-2018-0084
39. World Health Organization (WHO). Dementia [Report] (2024). Available at: https://www.who.int/news-room/fact-sheets/detail/dementia (Accessed December 13, 2024).
40. Report of AD association. 2024 Alzheimer’s disease facts and figures. Alzheimers Dement. [Report] (2024) 20:3708–821. doi: 10.1002/alz.13809
41. Abdel-Naseer, M. Epidemiology of dementia in developing countries. J Neurol Sci. (2019) 405:72–3. doi: 10.1016/j.jns.2019.10.202
42. Abrego-Guandique, DM, Saraceno, GF, Cannataro, R, de Burnside, MM, Caroleo, MC, and Cione, E. Apolipoprotein E and Alzheimer’s disease in Italian population: systematic review and Meta-analysis. Brain Sci. (2024) 14:908. doi: 10.3390/brainsci14090908
43. Croteau, E, Castellano, CA, Richard, MA, Fortier, M, Nugent, S, Lepage, M, et al. Ketogenic medium chain triglycerides increase brain energy metabolism in Alzheimer’s disease. J Alzheimers Dis. (2018) 64:551–61. doi: 10.3233/JAD-180202
44. Jiang, Z, Yin, X, Wang, M, Chen, T, Wang, Y, Gao, Z, et al. Effects of ketogenic diet on Neuroinflammation in neurodegenerative diseases. Aging Dis. (2022) 13:1146–65. doi: 10.14336/AD.2021.1217
45. Trotta, MC, Maisto, R, Guida, F, Boccella, S, Luongo, L, Balta, C, et al. The activation of retinal HCA2 receptors by systemic beta-hydroxybutyrate inhibits diabetic retinal damage through reduction of endoplasmic reticulum stress and the NLRP3 inflammasome. PLoS One. (2019) 14:e0211005. doi: 10.1371/journal.pone.0211005
46. DeLucas, M, Sánchez, J, Palou, A, and Serra, F. The impact of diet on miRNA regulation and its implications for health: a systematic review. Nutrients. (2024) 16:770. doi: 10.3390/nu16060770
47. Abrego-Guandique, DM, Galmés, S, García-Rodríguez, A, Cannataro, R, Caroleo, MC, Ribot, J, et al. β-Carotene impacts the liver MicroRNA profile in a sex-specific manner in mouse offspring of Western diet-fed mothers: results from microarray analysis by direct hybridization. Int J Mol Sci. (2024) 25:12899. doi: 10.3390/ijms252312899
48. Cannataro, R, and Cione, E. Diet and miRNA: epigenetic regulator or a new class of supplements? Microrna. (2022) 11:89–90. doi: 10.2174/2211536611666220510111711
49. He, Y, Cheng, X, Zhou, T, Li, D, Peng, J, Xu, Y, et al. Hydroxybutyrate as an epigenetic modifier: underlying mechanisms and implications. Heliyon. (2023) 9:e21098. doi: 10.1016/j.heliyon.2023.e21098
50. Cannataro, R, Perri, M, Gallelli, L, Caroleo, MC, De Sarro, G, and Cione, E. Ketogenic diet acts on body remodeling and microRNAs expression profile. Microrna. (2019) 8:116–26. doi: 10.2174/2211536608666181126093903
51. Choi, SE, Fu, T, Seok, S, Kim, DH, Yu, E, Lee, KW, et al. Elevated microRNA-34a in obesity reduces NAD+ levels and SIRT1 activity by directly targeting NAMPT. Aging Cell. (2013) 12:1062–72. doi: 10.1111/acel.12135
52. Xu, J, Jackson, CW, Khoury, N, Escobar, I, and Perez-Pinzon, MA. Brain SIRT1 mediates metabolic homeostasis and neuroprotection. Front Endocrinol (Lausanne). (2018) 702:9. doi: 10.3389/fendo.2018.00702
53. Ungaro, P, Nettore, IC, Franchini, F, Palatucci, G, Muscogiuri, G, Colao, A, et al. Epigenome modulation induced by ketogenic diets. Nutrients. (2022) 14:3245. doi: 10.3390/nu14153245
54. Kim, HJ, Leeds, P, and Chuang, D. The HDAC inhibitor, sodium butyrate, stimulates neurogenesis in the ischemic brain. J Neurochem. (2009) 110:1226–40. doi: 10.1111/j.1471-4159.2009.06212.x
55. Ahmad, Y, Seo, DS, and Jang, Y. Metabolic effects of ketogenic diets: exploring whole-body metabolism in connection with adipose tissue and other metabolic organs. Int J Mol Sci. (2024) 25:7076. doi: 10.3390/ijms25137076
56. Allan, NP, Yamamoto, BY, Kunihiro, BP, Nunokawa, CKL, Rubas, NC, Wells, RK, et al. Ketogenic diet induced shifts in the gut microbiome associate with changes to inflammatory cytokines and brain-related miRNAs in children with autism Spectrum disorder. Nutrients. (2024) 16:1401. doi: 10.3390/nu16101401
57. Ruiz-Herrero, J, Olaso-Gonzalez, G, Serna, E, Cañedo-Villarroya, E, Correas, AG, Gambini, J, et al. Transcriptomic profile of epileptic children treated with ketogenic therapies. J Integr Neurosci. (2022) 21:31. doi: 10.31083/j.jin2101031
58. Cannataro, R, Perri, M, Caroleo, MC, Gallelli, L, De Sarro, G, and Cione, E. Modulation of MicroRNAs linked to pain-migraine by ketogenic diet (P14-007-19). Curr Dev Nutr. (2019) 3:nzz052.P14-007-19. doi: 10.1093/cdn/nzz052.P14-007-19
59. Brinkworth, GD, Buckley, JD, Noakes, M, Clifton, PM, and Wilson, CJ. Long-term effects of a very low-carbohydrate diet and a low-fat diet on mood and cognitive function. Arch Intern Med. (2009) 169:1873–80. doi: 10.1001/archinternmed.2009.329
60. D’Anci, KE, Watts, KL, Kanarek, RB, and Taylor, HA. Low-carbohydrate weight-loss diets. Effects on cognition and mood. Appetite. (2009) 52:96–103. doi: 10.1016/j.appet.2008.08.009
61. Perdoncin, M, Konrad, A, Wyner, JR, Lohana, S, Pillai, SS, Pereira, DG, et al. A review of miRNAs as biomarkers and effect of dietary modulation in obesity associated cognitive decline and neurodegenerative disorders. Front Mol Neurosci. (2021) 14:14. doi: 10.3389/fnmol.2021.756499
62. O’Neill, B, and Raggi, P. The ketogenic diet: pros and cons. Atherosclerosis. (2020) 292:119–26. doi: 10.1016/j.atherosclerosis.2019.11.021
63. Masood, W, Annamaraju, P, Suheb, MZK, and Uppaluri, KR Ketogenic diet. In: StatPearls. Ed. K. Shumway (Treasure Island Florida: StatPearls Publishing) (2023)
Keywords: microRNAs, cognitive function, ketogenic diet, ketone bodies, biomarker
Citation: Abrego-Guandique DM, Cione E, Caroleo MC, Bonilla DA and Cannataro R (2025) Ketogenic diet and microRNAs: focus on cognitive function. Front. Nutr. 12:1545832. doi: 10.3389/fnut.2025.1545832
Edited by:
Domenico Sergi, University of Ferrara, ItalyReviewed by:
Leila Sadeghi-Reeves, Independent Researcher, Sion, SwitzerlandOrnella Cominetti, Nestlé Research Center, Switzerland
Olha Kostiuchenko, Polish Academy of Sciences, Poland
Copyright © 2025 Abrego-Guandique, Cione, Caroleo, Bonilla and Cannataro. This is an open-access article distributed under the terms of the Creative Commons Attribution License (CC BY). The use, distribution or reproduction in other forums is permitted, provided the original author(s) and the copyright owner(s) are credited and that the original publication in this journal is cited, in accordance with accepted academic practice. No use, distribution or reproduction is permitted which does not comply with these terms.
*Correspondence: Erika Cione, ZXJpa2EuY2lvbmVAdW5pY2FsLml0