- 1International Joint Laboratory on Quantum Sensing and Quantum Metrology, School of Physics, Huazhong University of Science and Technology, Wuhan, China
- 2Hubei Key Laboratory of Gravitation and Quantum Physics, Institute for Quantum Science and Engineering, Huazhong University of Science and Technology, Wuhan, China
Quantum sensing is a quantum technology for ultrasensitive detection, which is particularly useful for sensing weak signals at the nanoscale. Nitrogen vacancy centers in diamond, thanks to their superb quantum coherence under ambient conditions and the stability of the material in extreme and complicated environments, have been demonstrated as promising quantum probes in multi-parameter sensing. Their spin properties make them particularly sensitive to magnetic fields, but they are insensitive to temperature, electric field, pressure, etc., and even immune to some bio-parameters (e.g., pH and glucose concentration). Recently, hybrid quantum sensing has emerged as a promising avenue for further enhancing the capabilities of diamond sensors. Different techniques can potentially improve the sensitivity, range of detectable parameters, and sensing frequencies of diamond sensors. This review provides an overview of hybrid quantum sensing using diamond. We first give a brief introduction to quantum sensing using diamond, and then review various hybrid sensing schemes that have been developed to enhance the sensing capabilities of diamond sensors. Finally, the potential applications and challenges associated with hybrid quantum sensing in diamond are discussed.
1 Background and introduction
Quantum sensing [1] describes the use of a quantum system, quantum properties, or quantum phenomena to perform a measurement of physical quantities. It is a new technology that can detect weak signals beyond the classical limit. It is particularly useful for sensing weak signals at the nanoscale. With unprecedented sensitivity and nanoscale resolution, quantum sensing has become a powerful probe in varieties of applications. For instance, the discovery of new materials with intriguing physical phenomena, such as van der Waals materials [2–4], topological insulators [5, 6], and complex oxide interfaces [7], has increased the need for local and non-perturbative magnetic field sensors. Such a nano-magnetometer would enable the characterization of the growing number of correlated and topological systems, as spins and moving charges generate stray fields in those systems. In addition, temperature fluctuations at the nanometer scale pose a significant role in several scenarios, including temperature-induced control of gene expression [8–10], temperature heterogeneities in living cells [11, 12], and heat dissipation in integrated circuits [13, 14]. Sensitive detection of these fluctuations is highly desirable but challenging. A thermometer with microkelvin temperature resolution, operating across a wide temperature range and offering nanoscale spatial resolution, would greatly benefit researches in physics, biology, and chemistry.
Nitrogen-vacancy (NV) centers in diamond have gained significant attention as promising quantum sensors due to their superb quantum coherence under ambient conditions and nanoscale spatial resolution. NV centers have been demonstrated to be excellent quantum magnetometers [15–17], with the ability to operate across temperatures ranging from 350 mK [18] to 1000 K [19], magnetic fields ranging from ∼10
NV centers in diamond possess spin properties with unprecedented magnetic field sensitives. However, compared with magnetic field sensing, they are insensitive to other parameters, such as temperature [28–31], pressure [26], and electric field [32–34]; and even immune to many biochemical parameters (such as glucose or enzyme concentration, and pH). In order to enhance the sensitivities of parameters with a weak or even no spin response, hybrid quantum sensing has emerged. Insensitive or immune physical or biological parameters can be converted to magnetic signals and then detected by the NV center spin. This approach enables the detection and measurement of a broader range of parameters, extending the capabilities of diamond quantum sensing. Hybrid quantum sensing in diamond has already been demonstrated experimentally in recent years, showcasing the potential in multidisciplinary research.
This review focuses on recent advances on hybrid quantum sensing in diamond. We begin with an introduction to the fundamental properties and working principles of diamond quantum sensing. Hybrid sensing schemes are then discussed, with a particular emphasis on the enhanced sensitivities and broadened ranges of detectable parameters. Finally, the review finishes with outlooks for the potential applications and challenges of hybrid quantum sensing in diamond.
2 Brief introduction to diamond quantum sensing
NV center in diamond consists of a substitutional nitrogen atom and a vacancy [35–37] at the nearest neighborsite, as depicted in Figure 1A. When negatively charged, NV center can be treated as spin-1 system. The positively charged and neutral NV centers, however, are not of interest for quantum sensing due to the absence of magnetic resonance signals. Hereafter, all NV centers mentioned in this article refer to the negatively charged type. The energy levels of the NV center are illustrated in Figure 1B. By utilizing phonon-assisted optical absorption, the NV electron spin can be excited off-resonantly from its spin-triplet ground states to spin-triplet excited states using a 532 nm laser. The
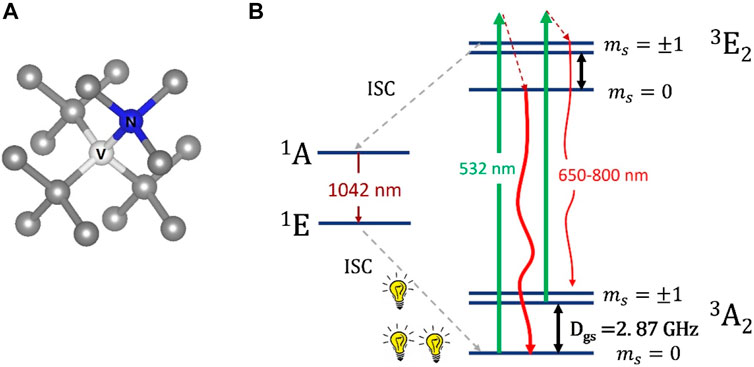
FIGURE 1. Nitrogen-vacancy (NV) center in diamond. (A) The atomic structure of NV center in diamond. The NV center consists of a substitutional nitrogen and a nearby vacancy. (B) Energy levels of an NV center. The spin is pumped into
The Hamiltonian of the ground states of an NV center gives the sensing principle and is written as
where
NV centers are primarily sensitive to magnetic fields, as manifested in the Zeeman term of Eq. 1 with a coupling strength
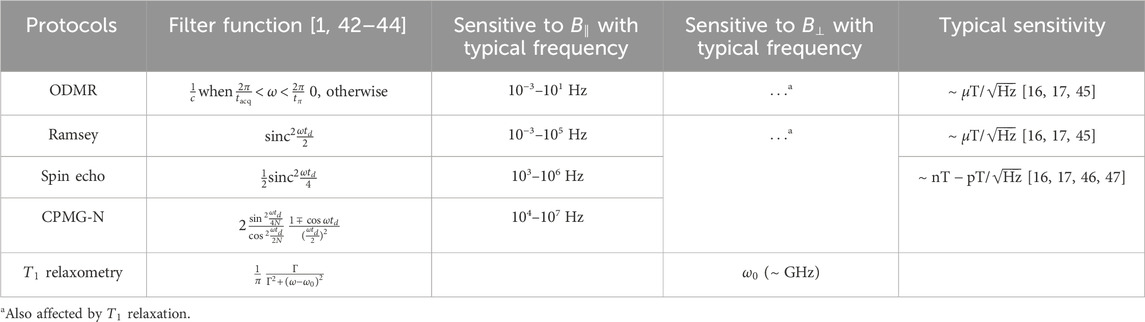
TABLE 1. Summary of the filter functions, sensing frequencies, and sensitivity for the three types of sensing protocols. For the transverse spin relaxation measurements, Ramsey, spin echo and CPMG-N (N is the number of the flip pi pulse) are listed as examples.
NV centers are also responsive to temperature, pressure, and electric fields, although the sensitivity is much lower than magnetic fields. The zero-field splitting
3 Hybrid diamond quantum sensing
Hybrid sensing is a strategy for accurately measuring insensitve parameters by detecting a different parameter to which the NV center is highly sensitive [48]. This section reviews recent advances in hybrid sensing using NV centers in diamond, for measurement of temperatures, pressure, and bio-parameters, as well as further boosted sensitivity to magnetic fields compared with pristine NV centers.
3.1 Temperature sensing
NV thermometry has been attractive for the past 10 years due to its nanoscale spatial resolution, high thermal diffusivity (fast thermal response), and the wide working temperature ranges from cryogenics to 1000 K. In particular, the good bio-compatibility also ensures its application in probing thermal behaviors in biological systems [31, 49–51]. The temperature response of NV centers was first reported in 2010 [28]. The zero-field splitting
Hybrid schemes have been proposed and demonstrated to improve the sensitivity of temperature sensing by using magnetic nanoparticles (MNPs). Two hybrid schemes have been proposed and demonstrated, both of which convert temperature variations into magnetic field changes that can be detected by NV centers. The first scheme is based on magnetic criticality enhancement [54]. In this configuration, a single Cu1-xNix alloy MNP is combined with a nanodiamond containing ensemble NV centers, as illustrated in Figure 2A. The magnetization of the MNP can be used to monitor the local temperature, and this mechanism becomes highly sensitive when the temperature is close to the magnetic phase transition point (i.e., critical point) of the MNP, as shown in Figure 2B. This results in a sensitivity of
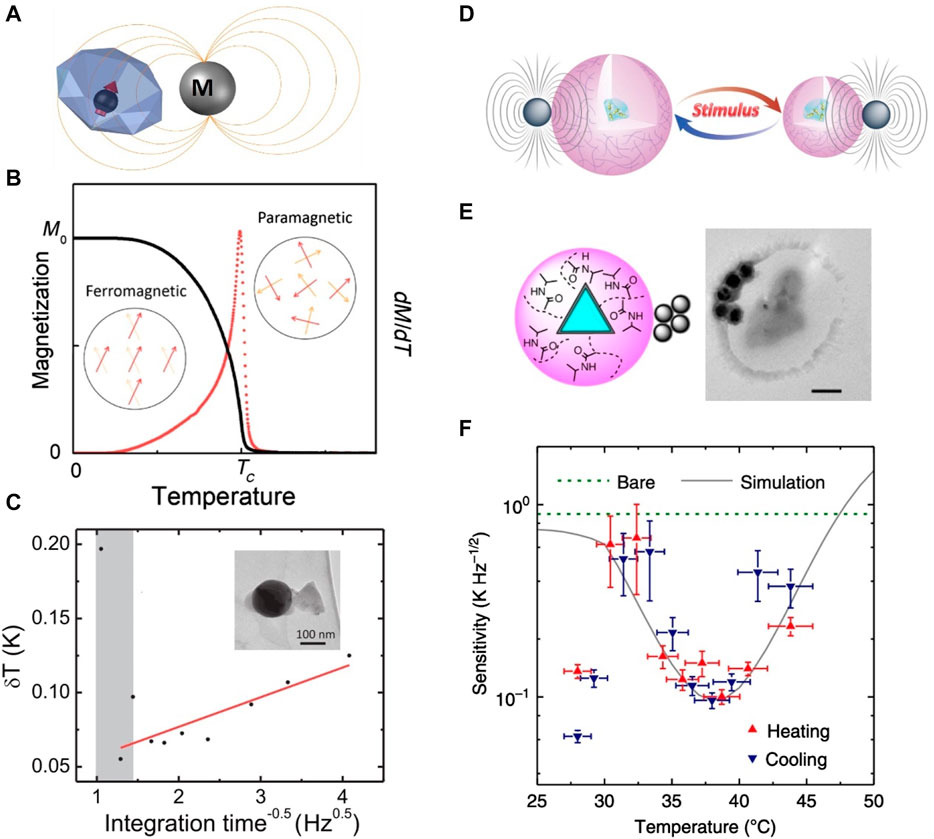
FIGURE 2. Hybrid diamond nano-thermometry. (A) Schematic of a hybrid nanosensor, composed of a fluorescent nanodiamond and a magnetic nanoparticle (MNP). (B) The magnetic moment M (black solid line) of the MNP decreases dramatically with temperature increasing to the ferromagnetic-paramagnetic transition, with the temperature susceptibility dM/dT (red dotted line) peaking at the critical temperature
The second scheme involves the use of both MNPs and a functional hydrogel [55], as depicted in Figures 2D, E. In this configuration, a stimulus-responsive hydrogel acts as a spacing transducer between a nanodiamond with NV centers and MNPs. The volume phase transition of the hydrogel, triggered by temperature stimulation, leads to a sharp variation in the separation distance between the MNPs and nanodiamonds. This results in a magnetic field change that can be detected by NV centers. Using this scheme, a temperature sensitivity of
Both of these methods rely on thermally responsive phase changes to achieve high sensitivity to temperature. However, sensing range of temperature is limited by the phase change point and dynamics. In the case of the magnetic criticality enhanced hybrid nano-thermometer, the working temperature range is within ∼20 K below the Curie temperature. Although the working range can be increased by applying an external magnetic field, it will compromise the temperature sensitivity as the magnetic susceptibility becomes less responsive to temperature under external magnetic field. The limited sensing range can be mitigated by tuning the Curie temperature of magnetic nanoparticles through alloying to meet the requirement of different scenarios. For example, the Curie temperature of the copper-nickel magnetic nanoparticles can be continuously tuned from 4 K to 600 K by manipulating the nickel composition [54]. For hybrid sensing based on volume phase changes of hydrogels, the working range is within only ±7 K near the critical temperature [55]. The utilization of hydrogel also limits the applications in higher temperature sensing due to the solvent evaporation.
3.2 Pressure sensing
Hybrid sensing schemes have also been proposed and demonstrated to improve the sensitivity of pressure and force sensing using diamond. One approach is to deposit a piezomagnetic film, such as Tb0.27Dy0.73Fe2 (Terfenol-D), onto a bulk diamond as a signal transducer, as shown in Figure 3A [58]. The piezomagnetic film exhibits high magnetostrictive effects, allowing the external stress to be converted into a magnetic field change. Simulation results show that the sensitivity for measuring stress (and force) can reach
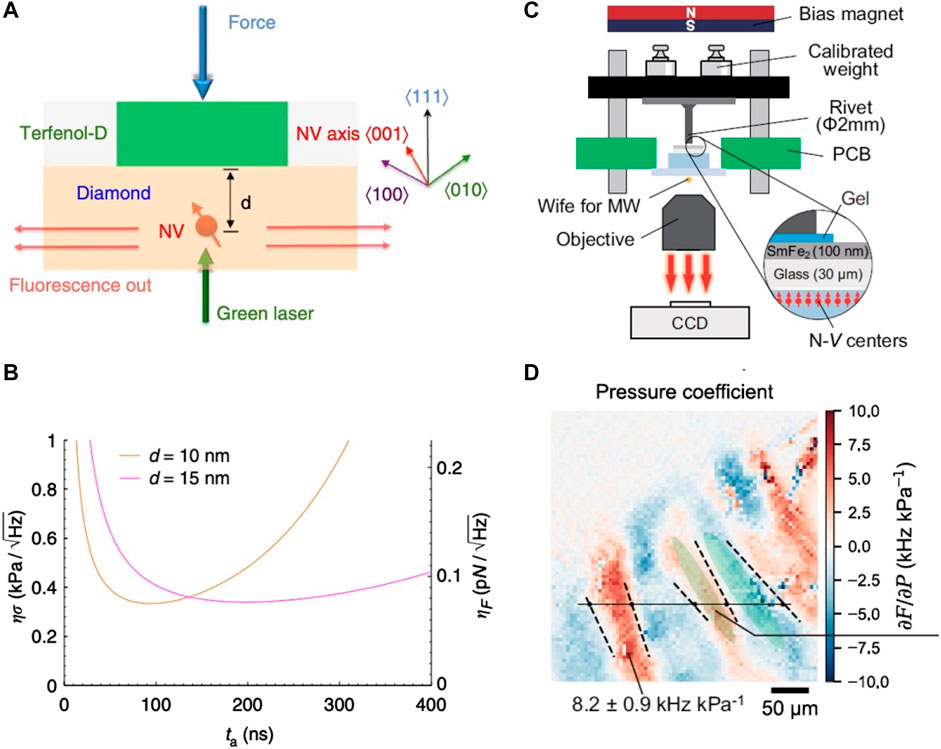
FIGURE 3. Hybrid diamond pressure sensing. (A) The model hybrid system of diamond (brown) and piezomagnetic film (green). (B) Simulation results of the shot-noise-limited sensitivity for the measurement of stress (and force) as a function of the integration time (
However, there remain some issues to be addressed for applications. For instance, the pressure coefficient is correlated with magnetic domain structures, as shown in Ref. [59]. This correlation could lead to an inhomogeneous pressure response in wide-field pressure imaging, which requires complicated calibration efforts. The authors also proposed that using magnetic disk arrays could potentially address these issues, which could be explored in future research [59].
3.3 Bio-parameter sensing
Hybrid sensing can enable accurate detection of bio-parameters which NV centers show no spin response. A recent theoretical work proposed that
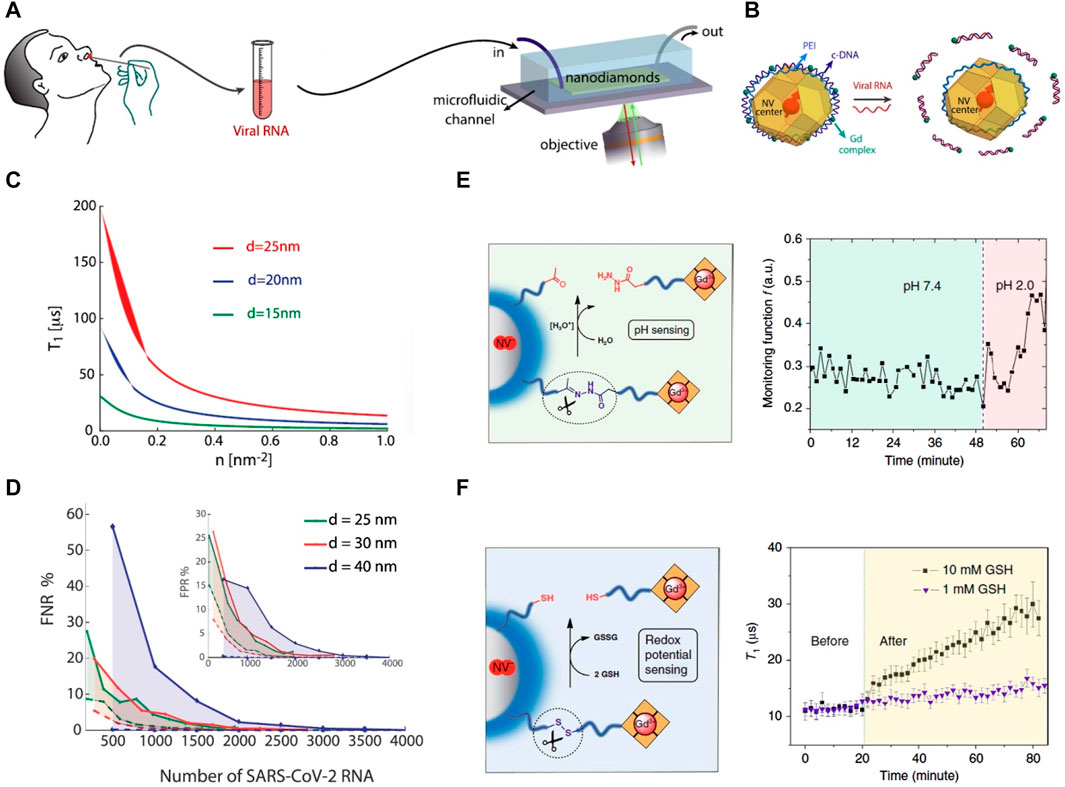
FIGURE 4. Hybrid diamond bio-sensors. (A) Overview of the diagnosis protocols. (B) Mechanism of magnetic noise quenching. In the presence of virus RNA, the base-pair matching of c-DNA and RNA leads to the detachment of c-DNA-DOTA-Gd3+ from the nanodiamond surface, resulting in weaker magnetic interaction between Gd3+ complex and NV centers inside the nanodiamond. (C) Simulation of relaxation time
3.4 Ultrasensitive magnetic field sensing
Hybrid sensing strategies can further enhance the sensitivity to magnetic fields. For example, the sensitivity to magnetic field can be dramatically enhanced by placing a ferromagnetic (FM) particle between the NV center and a target spin. In this theoretically proposed scheme, the target spin resonantly drives magnetization of the FM particle, which can be regarded as a macrospin that amplifies signal detected by the NV center. The magnetic sensitivity is potentially capable of detecting a single nuclear spin with milliseconds of data acquisition [63]. Another approach is to combine NV centers with magnetic flux concentrators (MFCs) with high magnetic permeability to magnify the magnetic fields [64, 65], as shown in Figure 5A. The measured sensitivity can reach 0.9
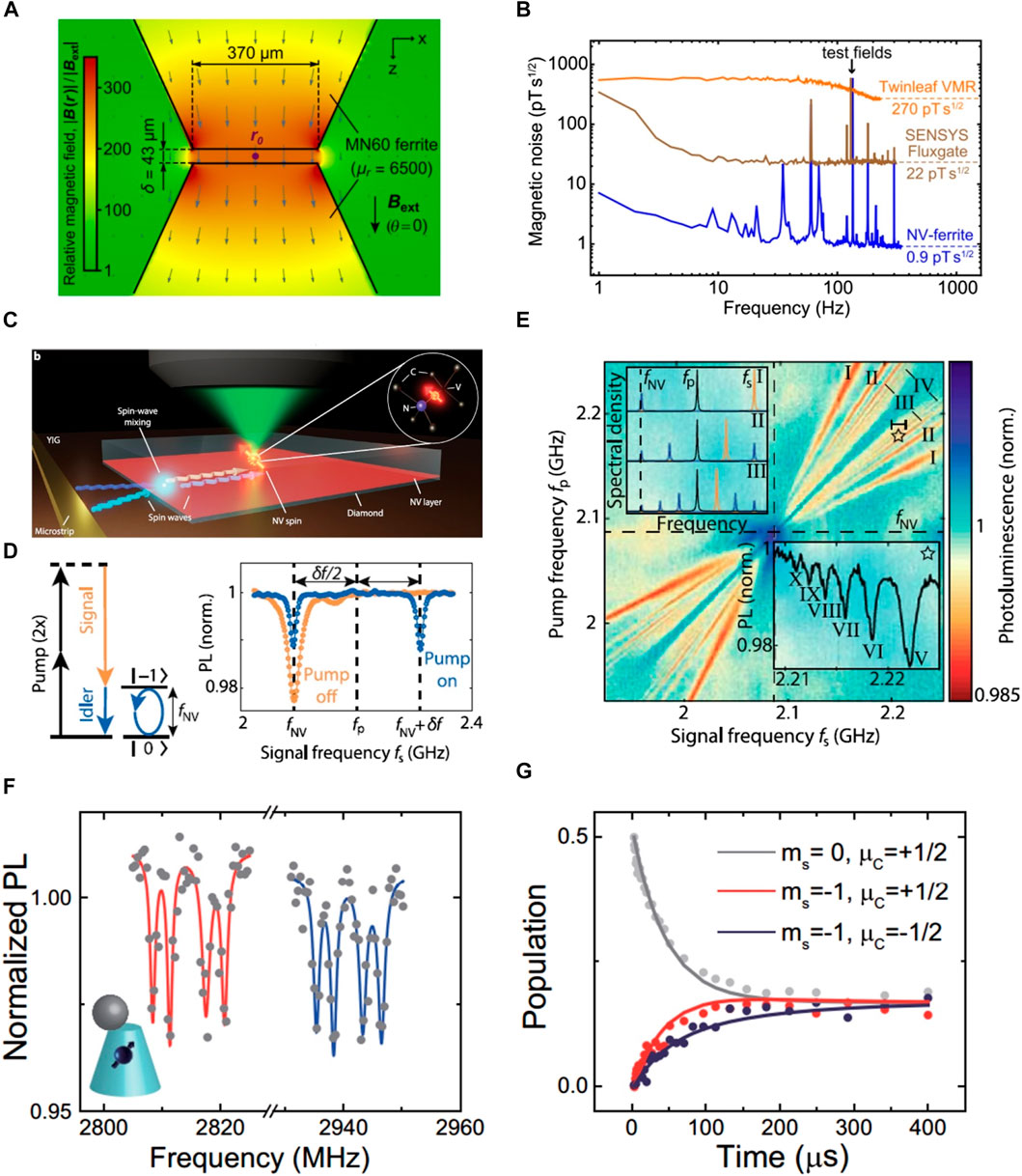
FIGURE 5. Hybrid schemes to enhance magnetic field sensing. (A) MFC structures is combined with ensemble NVs in bulk diamond. The magnetic material here is MN60 ferrite with a permeability of
In addition, the sensing frequency range of NV centers in microwave regimes can be broadened through hybridization with other systems, such as nuclear spins, thin film magnets, and others. Current literature mainly relied on the tuning of electron spin resonance frequencies using a bias field for manipulating the sensing frequency range. However, the bias field can affect the properties such as permeability, magnetic domain structures, and others, of the samples. To address this, one approach is to interface NV centers in diamond with a thin-film magnet [66]. By applying a local pump field, microwave signals can be converted to the NV spin frequency via the non-linear spin-wave dynamics of the magnet, as shown in Figures 5C, D. This allows for the detection of microwave signals that are detuned by several GHz from the NV resonance frequency, enabling the characterization of spin-wave band structures and non-linear spin-wave dynamics, as shown in Figure 5E. Another approach is to couple NV centers with 13C nuclear spins [20]. This coupling can enable multi-frequency sensing at microwave frequencies. By manipulating the states of both the NV center and the 13C nuclear spins, it is possible to probe and measure different frequencies simultaneously, as shown in Figures 5F, G. These hybridization techniques open up new possibilities for NV centers to be used as versatile quantum sensors in a wider range of frequencies and applications.
Finally, hybrid sensing can also be achieved by coupling NV centers with surface quantum excitations. For example, the photoluminescence of NV centers can be largely boosted by coupling NV centers to gold/silver plasmonic nanostructures [67, 68]. The microwave-spin interaction can also be enhanced by coupling NV centers with surface magnons by employing focused electromagnetic fields using a hybrid nanowire-bowtie antenna [69].
4 Opportunities and challenges
Hybrid sensing significantly enhances the sensing capabilities of NV centers in diamond (a comparison between bare NV sensing and hybrid NV sensing is summarized in Table 2), opening up exciting possibilities for advancing diamond quantum sensing applications. Among these, precise nanoscale temperature sensing emerges as a particularly promising avenue. Given the tight thermoregulation of cellular compositions, gaining insights into temperature variations within living cells is imperative for unraveling the mechanisms governing temperature-dependent biological processes. The hybrid nanothermometer, leveraging magnetic criticality enhancements, offers an impressive boost in sensitivity up to two orders of magnitude, which is promising for resolving the long-standing controversies in understanding temperature heterogeneities within living cells. By choosing magnetic nanoparticles with Cuire temperatures around 37°C, the working temperature range of hybrid sensors can be used for in vivo thermal characterizations of living cells, although the calibration can be complicated. One possible approach to solving the aforementioned challenge is to conduct the in vivo measurement and then perform the calibration after the death of the cell, which involves real-time three-dimensional tracking of the sensors [70, 71].
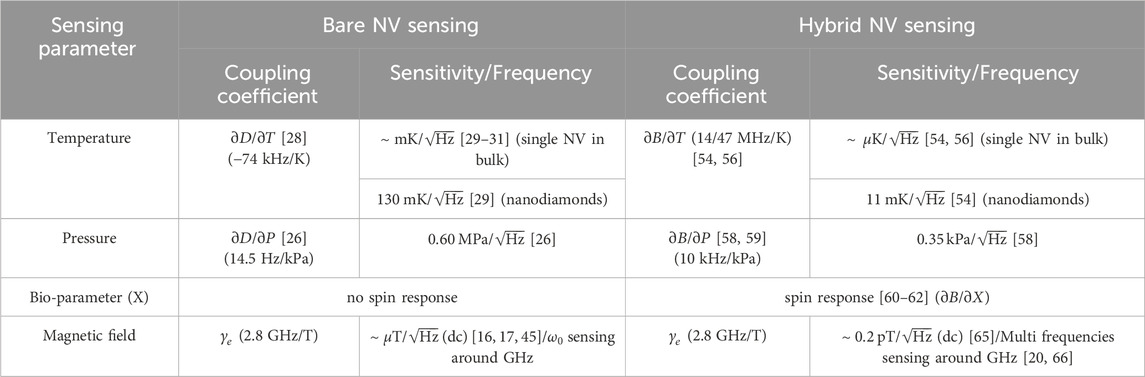
TABLE 2. A comparison between bare NV sensing and hybrid sensing for different sensing parameters. For magnetic field sensing, only sensing frequency around GHz is listed and compared.
Hybrid sensing opens up the possibilities of using quantum probes for understanding bio-processes in the future. In microreactors such as living cells, hybrid quantum sensors can be used to study the nanoscale variations in pH and concentration of species playing a pivotal role in biochemical reactions, such as ATP synthesis and the release or consumption of neurotransmitters. However, many reported hybrid bio-sensors are one-time indicators as discussed above, and developing sensors that can achieve real-time monitoring of bio-signals can be a fruitful direction.
As for sensing magnetic fields, the enhanced sensitivities achieved with NV diamond quantum sensors and MFCs are approaching to those of established technologies like superconducting quantum interference devices and atomic vapor-based sensors. However, the spatial resolution is also reduced at the same time when MFCs are introduced. In principle, miniaturizing the MFC could potentially improve spatial resolution to some extent. However, the enhancement factor depends on the magnetic permeability of the material and the geometry of the MFCs. Therefore, search for magnetic materials with suitable permeability and the optimization of the MFC design geometry should be paid enough attention in future researches. This enhanced magnetic field sensitivity opens up possibilities of future applications in precision navigation, geoscience, and medical imaging, where accurate detection and measurement of magnetic fields are crucial.
Although this article is focused on NV centers in diamond, the hybrid sensing schemes summarized in this review can also be used in other defects in solids as well, such as SiV [72, 73] and GeV [74, 75] in diamond, SiV [76, 77]and divacancy centers [78, 79] in silicon carbide, and defect centers in 2D materials [80–82].
To conclude, past decade witnessed rapid progresses in developing hybrid quantum sensors, and we expect hybrid quantum sensing will impact multidisciplinary research in fields such as non-equilibrium thermodynamics in bio-systems, heat dissipation in nanodevices, and bio-signal detection in disease diagnostics, and many others. Furthermore, future practical implementation and commercialization of quantum hybrid sensors require interdisciplinary efforts, involving techniques in quantum information science, condensed matter physics, and technology for sensor development, as well as expertise from various other fields (biology, chemistry, engineering, etc.).
Author contributions
NW: Conceptualization, Resources, Writing–original draft, Funding acquisition; JC: Conceptualization, Resources, Writing–review and editing, Funding acquisition.
Funding
The author(s) declare financial support was received for the research, authorship, and/or publication of this article. This work was supported by the National Natural Science Foundation of China (12304571, 12161141011).
Conflict of interest
The authors declare that the research was conducted in the absence of any commercial or financial relationships that could be construed as a potential conflict of interest.
Publisher’s note
All claims expressed in this article are solely those of the authors and do not necessarily represent those of their affiliated organizations, or those of the publisher, the editors and the reviewers. Any product that may be evaluated in this article, or claim that may be made by its manufacturer, is not guaranteed or endorsed by the publisher.
References
1. Degen CL, Reinhard F, Cappellaro P. Quantum sensing. Rev Mod Phys (2017) 89:035002. doi:10.1103/RevModPhys.89.035002
2. Gong C, Zhang X. Two-dimensional magnetic crystals and emergent heterostructure devices. Science (2019) 363:eaav4450. doi:10.1126/science.aav4450
3. Huang B, Clark G, Navarro-Moratalla E, Klein DR, Cheng R, Seyler KL, et al. Layer-dependent ferromagnetism in a van Der Waals crystal down to the monolayer limit. Nature (2017) 546:270–3. doi:10.1038/nature22391
4. Gong C, Li L, Li Z, Ji H, Stern A, Xia Y, et al. Discovery of intrinsic ferromagnetism in two-dimensional van Der Waals crystals. Nature (2017) 546:265–9. doi:10.1038/nature22060
5. Hasan MZ, Kane CL. Colloquium: topological insulators. Rev Mod Phys (2010) 82:3045–67. doi:10.1103/revmodphys.82.3045
6. Soumyanarayanan A, Reyren N, Fert A, Panagopoulos C. Emergent phenomena induced by spin–orbit coupling at surfaces and interfaces. Nature (2016) 539:509–17. doi:10.1038/nature19820
7. Hwang HY, Iwasa Y, Kawasaki M, Keimer B, Nagaosa N, Tokura Y. Emergent phenomena at oxide interfaces. Nat Mater (2012) 11:103–13. doi:10.1038/nmat3223
8. Kamei Y, Suzuki M, Watanabe K, Fujimori K, Kawasaki T, Deguchi T, et al. Infrared laser–mediated gene induction in targeted single cells in vivo. Nat Methods (2009) 6:79–81. doi:10.1038/nmeth.1278
9. Lauschke VM, Tsiairis CD, François P, Aulehla A. Scaling of embryonic patterning based on phase-gradient encoding. Nature (2013) 493:101–5. doi:10.1038/nature11804
10. Kumar SV, Wigge PA. H2A.Z-Containing nucleosomes mediate the thermosensory response in arabidopsis. Cell (2010) 140:136–47. doi:10.1016/j.cell.2009.11.006
11. Kiyonaka S, Sakaguchi R, Hamachi I, Morii T, Yoshizaki T, Mori Y. Validating subcellular thermal changes revealed by fluorescent thermosensors. Nat Methods (2015) 12:801–2. doi:10.1038/nmeth.3548
12. Baffou G, Rigneault H, Marguet D, Jullien L. A critique of methods for temperature imaging in single cells. Nat Methods (2014) 11:899–901. doi:10.1038/nmeth.3073
13. Mecklenburg M, Hubbard WA, White ER, Dhall R, Cronin SB, Aloni S, et al. Nanoscale temperature mapping in operating microelectronic devices. Science (2015) 347:629–32. doi:10.1126/science.aaa2433
14. Menges F, Mensch P, Schmid H, Riel H, Stemmer A, Gotsmann B. Temperature mapping of operating nanoscale devices by scanning probe thermometry. Nat Commun (2016) 7:10874. doi:10.1038/ncomms10874
15. Taylor JM, Cappellaro P, Childress L, Jiang L, Budker D, Hemmer PR, et al. High-sensitivity diamond magnetometer with nanoscale resolution. Nat Phys (2008) 4:810–6. doi:10.1038/nphys1075
16. Balasubramanian G, Chan IY, Kolesov R, Al-Hmoud M, Tisler J, Shin C, et al. Nanoscale imaging magnetometry with diamond spins under ambient conditions. Nature (2008) 455:648–51. doi:10.1038/nature07278
17. Maze JR, Stanwix PL, Hodges JS, Hong S, Taylor JM, Cappellaro P, et al. Nanoscale magnetic sensing with an individual electronic spin in diamond. Nature (2008) 455:644–7. doi:10.1038/nature07279
18. Scheidegger PJ, Diesch S, Palm ML, Degen CL. Scanning nitrogen-vacancy magnetometry down to 350 MK. Appl Phys Lett (2022) 120:224001. doi:10.1063/5.0093548
19. Liu G-Q, Feng X, Wang N, Li Q, Liu R-B. Coherent quantum control of nitrogen-vacancy center spins near 1000 kelvin. Nat Commun (2019) 10:1344. doi:10.1038/s41467-019-09327-2
20. Wang N, Liu CF, Fan JW, Feng X, Leong WH, Finkler A, et al. Zero-field magnetometry using hyperfine-biased nitrogen-vacancy centers near diamond surfaces. Phys Rev Res (2022) 4:013098. doi:10.1103/physrevresearch.4.013098
21. Lenz T, Wickenbrock A, Jelezko F, Balasubramanian G, Budker D. Magnetic sensing at zero field with a single nitrogen-vacancy center. Quan Sci. Technol. (2021) 6:034006. doi:10.1088/2058-9565/abffbd
22. Zheng H, Xu J, Iwata GZ, Lenz T, Michl J, Yavkin B, et al. Zero-field magnetometry based on nitrogen-vacancy ensembles in diamond. Phys Rev Appl (2019) 11:064068. doi:10.1103/physrevapplied.11.064068
23. Fortman B, Pena J, Holczer K, Takahashi S. Demonstration of NV-detected ESR spectroscopy at 115 GHz and 4.2 T. Appl Phys Lett (2020) 116:174004. doi:10.1063/5.0006014
24. Stepanov V, Cho FH, Abeywardana C, Takahashi S. High-frequency and high-field optically detected magnetic resonance of nitrogen-vacancy centers in diamond. Appl Phys Lett (2015) 106:063111. doi:10.1063/1.4908528
25. Aslam N, Pfender M, Stöhr R, Neumann P, Scheffler M, Sumiya H, et al. Single spin optically detected magnetic resonance with 60–90 GHz (E-Band) microwave resonators. Rev Sci Instrum (2015) 86:064704. doi:10.1063/1.4922664
26. Doherty MW, Struzhkin VV, Simpson DA, McGuinness LP, Meng Y, Stacey A, et al. Electronic properties and metrology applications of the diamond NV - center under pressure. Phys Rev Lett (2014) 112:047601. doi:10.1103/physrevlett.112.047601
27. Dai J-H, Shang Y-X, Yu Y-H, Xu Y, Yu H, Hong F, et al. Optically detected magnetic resonance of diamond nitrogen-vacancy centers under megabar pressures. Chin Phys. Lett. (2022) 39:117601. doi:10.1088/0256-307x/39/11/117601
28. Acosta VM, Bauch E, Ledbetter MP, Waxman A, Bouchard L-S, Budker D. Temperature dependence of the nitrogen-vacancy magnetic resonance in diamond. Phys Rev Lett (2010) 104:070801. doi:10.1103/physrevlett.104.070801
29. Neumann P, Jakobi I, Dolde F, Burk C, Reuter R, Waldherr G, et al. High-precision nanoscale temperature sensing using single defects in diamond. Nano Lett (2013) 13:2738–42. doi:10.1021/nl401216y
30. Toyli DM, de las Casas CF, Christle DJ, Dobrovitski VV, Awschalom DD. Fluorescence thermometry enhanced by the quantum coherence of single spins in diamond. Proc Natl Acad Sci (2013) 110:8417–21. doi:10.1073/pnas.1306825110
31. Kucsko G, Maurer PC, Yao NY, Kubo M, Noh HJ, Lo PK, et al. Nanometre-scale thermometry in a living cell. Nature (2013) 500:54–8. doi:10.1038/nature12373
32. Dolde F, Fedder H, Doherty MW, Nöbauer T, Rempp F, Balasubramanian G, et al. Electric-field sensing using single diamond spins. Nat Phys (2011) 7:459–63. doi:10.1038/nphys1969
33. Michl J, Steiner J, Denisenko A, Bülau A, Zimmermann A, Nakamura K, et al. Robust and accurate electric field sensing with solid state spin ensembles. Nano Lett (2019) 19:4904–10. doi:10.1021/acs.nanolett.9b00900
34. Bian K, Zheng W, Zeng X, Chen X, Stöhr R, Denisenko A, et al. Nanoscale electric-field imaging based on a quantum sensor and its charge-state control under ambient condition. Nat Commun (2021) 12:2457. doi:10.1038/s41467-021-22709-9
35. Gruber A, Drabenstedt A, Tietz C, Fleury L, Wrachtrup J, von Borczyskowski C. Scanning confocal optical microscopy and magnetic resonance on single defect centers. Science (1997) 276:2012–4. doi:10.1126/science.276.5321.2012
36. Rondin L, Tetienne JP, Hingant T, Roch JF, Maletinsky P, Jacques V. Magnetometry with nitrogen-vacancy defects in diamond. Rep Prog. Phys. (2014) 77:056503. doi:10.1088/0034-4885/77/5/056503
37. Casola F, Van Der Sar T, Yacoby A. Probing condensed matter physics with magnetometry based on nitrogen-vacancy centres in diamond. Nat Rev Mater (2018) 3:17088. doi:10.1038/natrevmats.2017.88
38. Broadway DA, Johnson BC, Barson MSJ, Lillie SE, Dontschuk N, McCloskey DJ, et al. Microscopic imaging of the stress tensor in diamond using in situ quantum sensors. Nano Lett (2019) 19:4543–50. doi:10.1021/acs.nanolett.9b01402
39. Kehayias P, Turner MJ, Trubko R, Schloss JM, Hart CA, Wesson M, et al. Imaging crystal stress in diamond using ensembles of nitrogen-vacancy centers. Phys Rev B (2019) 100:174103. doi:10.1103/physrevb.100.174103
40. Hsieh S, Bhattacharyya P, Zu C, Mittiga T, Smart TJ, Machado F, et al. Imaging stress and magnetism at high pressures using a nanoscale quantum sensor. Science (2019) 366:1349–54. doi:10.1126/science.aaw4352
41. de Lange G, Wang ZH, Ristè D, Dobrovitski VV, Hanson R. Universal dynamical decoupling of a single solid-state spin from a spin bath. Science (2010) 330:60–3. doi:10.1126/science.1192739
42. Zhao N, Ho S-W, Liu R-B. Decoherence and dynamical decoupling control of nitrogen vacancy center electron spins in nuclear spin baths. Phys Rev B (2012) 85:115303. doi:10.1103/physrevb.85.115303
43. Yang W, Ma W-L, Liu R-B. Quantum many-body theory for electron spin decoherence in nanoscale nuclear spin baths. Rep Prog. Phys. (2017) 80:016001. doi:10.1088/0034-4885/80/1/016001
44. Schäfer-Nolte E, Schlipf L, Ternes M, Reinhard F, Kern K, Wrachtrup J. Tracking temperature-dependent relaxation times of ferritin nanomagnets with a wideband quantum spectrometer. Phys Rev Lett (2014) 113:217204. doi:10.1103/physrevlett.113.217204
45. Barry JF, Schloss JM, Bauch E, Turner MJ, Hart CA, Pham LM, et al. Sensitivity optimization for NV-diamond magnetometry. Rev Mod Phys (2020) 92:015004. doi:10.1103/revmodphys.92.015004
46. Wolf T, Neumann P, Nakamura K, Sumiya H, Ohshima T, Isoya J, et al. Subpicotesla diamond magnetometry. Phys Rev X (2015) 5:041001. doi:10.1103/physrevx.5.041001
47. Zhao Z, Ye X, Xu S, Yu P, Yang Z, Kong X, et al. Sub-nanotesla sensitivity at the nanoscale with a single spin. Natl Sci Rev (2023) 10:nwad100. doi:10.1093/nsr/nwad100
48. Wrachtrup J, Finkler A. Hybrid sensors ring the changes. Nature (2014) 512:380–1. doi:10.1038/512380a
49. Choi J, Zhou H, Landig R, Wu HY, Yu X, Von Stetina SE, et al. Probing and manipulating embryogenesis via nanoscale thermometry and temperature control. Proc Natl Acad Sci (2020) 117:14636–41. doi:10.1073/pnas.1922730117
50. Fujiwara M, Sun S, Dohms A, Nishimura Y, Suto K, Takezawa Y, et al. Real-time nanodiamond thermometry probing in vivo thermogenic responses. Sci Adv (2020) 6:eaba9636. doi:10.1126/sciadv.aba9636
51. Simpson DA, Morrisroe E, McCoey JM, Lombard AH, Mendis DC, Treussart F, et al. Non-neurotoxic nanodiamond probes for intraneuronal temperature mapping. ACS Nano (2017) 11:12077–86. doi:10.1021/acsnano.7b04850
52. Plakhotnik T, Doherty MW, Cole JH, Chapman R, Manson NB. All-optical Thermometry and thermal Properties of the optically detected spin Resonances of the NV–Center in nanodiamond. Nano Lett (2014) 14:4989–96. doi:10.1021/nl501841d
53. Fukami M, Yale CG, Andrich P, Liu X, Heremans FJ, Nealey PF, et al. All-optical cryogenic thermometry based on nitrogen-vacancy centers in nanodiamonds. Phys Rev Appl (2019) 12:014042. doi:10.1103/physrevapplied.12.014042
54. Wang N, Liu GQ, Leong WH, Zeng H, Feng X, Li SH, et al. Magnetic criticality enhanced hybrid nanodiamond thermometer under ambient conditions. Phys Rev X (2018) 8:011042. doi:10.1103/physrevx.8.011042
55. Zhang T, Liu G-QQ, Leong W-HH, Liu C-FF, Kwok M-HH, Ngai T, et al. Hybrid nanodiamond quantum sensors enabled by volume phase transitions of hydrogels. Nat Commun (2018) 9:3188. doi:10.1038/s41467-018-05673-9
56. Liu C-F, Leong W-H, Xia K, Feng X, Finkler A, Denisenko A, et al. Ultra-sensitive hybrid diamond nanothermometer. Natl Sci Rev (2021) 8. doi:10.1093/nsr/nwaa194
57. Zhang S-C, Li S, Du B, Dong Y, Zheng Y, Lin H-B, et al. Thermal-demagnetization-enhanced hybrid fiber-based thermometer coupled with nitrogen-vacancy centers. Opt Mater Express (2019) 9:4634. doi:10.1364/OME.9.004634
58. Cai J, Jelezko F, Plenio MB. Hybrid sensors based on colour centres in diamond and piezoactive layers. Nat Commun (2014) 5:4065. doi:10.1038/ncomms5065
59. Kitagawa R, Nagata S, Arai K, Mizuno K, Tsuji T, Fujisaki I, et al. Pressure sensor using a hybrid structure of a magnetostrictive layer and nitrogen-vacancy centers in diamond. Phys Rev Appl (2023) 19:044089. doi:10.1103/physrevapplied.19.044089
60. Li C, Soleyman R, Kohandel M, Cappellaro P. SARS-CoV-2 quantum sensor based on nitrogen-vacancy centers in diamond. Nano Lett (2022) 22:43–9. doi:10.1021/acs.nanolett.1c02868
61. Rendler T, Neburkova J, Zemek O, Kotek J, Zappe A, Chu Z, et al. Optical imaging of localized chemical events using programmable diamond quantum nanosensors. Nat Commun (2017) 8:14701. doi:10.1038/ncomms14701
62. Fujisaku T, Tanabe R, Onoda S, Kubota R, Segawa TF, So FTK, et al. PH nanosensor using electronic spins in diamond. ACS Nano (2019) 13:11726–32. doi:10.1021/acsnano.9b05342
63. Trifunovic L, Pedrocchi FL, Hoffman S, Maletinsky P, Yacoby A, Loss D. High-efficiency resonant amplification of weak magnetic fields for single spin magnetometry at room temperature. Nat Nanotechnol (2015) 10:541–6. doi:10.1038/nnano.2015.74
64. Fescenko I, Jarmola A, Savukov I, Kehayias P, Smits J, Damron J, et al. Diamond magnetometer enhanced by ferrite flux concentrators. Phys Rev Res (2020) 2:023394. doi:10.1103/physrevresearch.2.023394
65. Xie Y, Yu H, Zhu Y, Qin X, Rong X, Duan C-K, et al. A hybrid magnetometer towards femtotesla sensitivity under ambient conditions. Sci Bull (2021) 66:127–32. doi:10.1016/j.scib.2020.08.001
66. Carmiggelt JJ, Bertelli I, Mulder RW, Teepe A, Elyasi M, Simon BG, et al. Broadband microwave detection using electron spins in a hybrid diamond-magnet sensor chip. Nat Commun (2023) 14:490. doi:10.1038/s41467-023-36146-3
67. Kolesov R, Grotz B, Balasubramanian G, Stöhr RJ, Nicolet AAL, Hemmer PR, et al. Wave–particle duality of single surface plasmon polaritons. Nat Phys (2009) 5:470–4. doi:10.1038/nphys1278
68. Bogdanov SI, Shalaginov MY, Lagutchev AS, Chiang CC, Shah D, Baburin AS, et al. Ultrabright room-temperature sub-nanosecond emission from single nitrogen-vacancy centers coupled to nanopatch antennas. Nano Lett (2018) 18:4837–44. doi:10.1021/acs.nanolett.8b01415
69. Chen X-D, Wang E-H, Shan L-K, Feng C, Zheng Y, Dong Y, et al. Focusing the electromagnetic field to 10−6λ for ultra-high enhancement of field-matter interaction. Nat Commun (2021) 12:6389. doi:10.1038/s41467-021-26662-5
70. Feng X, Leong WH, Xia K, Liu CF, Liu GQ, Rendler T, et al. Association of nanodiamond rotation dynamics with cell activities by translation-rotation tracking. Nano Lett (2021) 21:3393–400. doi:10.1021/acs.nanolett.0c04864
71. McGuinness LP, Yan Y, Stacey A, Simpson DA, Hall LT, Maclaurin D, et al. Quantum measurement and orientation tracking of fluorescent nanodiamonds inside living cells. Nat Nanotechnol (2011) 6:358–63. doi:10.1038/nnano.2011.64
72. Rose BC, Huang D, Zhang ZH, Stevenson P, Tyryshkin AM, Sangtawesin S, et al. Observation of an environmentally insensitive solid-state spin defect in diamond. Science (2018) 361:60–3. doi:10.1126/science.aao0290
73. Choi S, Agafonov VN, Davydov VA, Plakhotnik T. Ultrasensitive all-optical thermometry using nanodiamonds with a high concentration of silicon-vacancy centers and multiparametric data analysis. ACS Photon (2019) 6:1387–92. doi:10.1021/acsphotonics.9b00468
74. Bhaskar MK, Sukachev D, Sipahigil A, Evans R, Burek M, Nguyen C, et al. Quantum nonlinear optics with a germanium-vacancy color center in a nanoscale diamond waveguide. Phys Rev Lett (2017) 118:223603. doi:10.1103/physrevlett.118.223603
75. Fan JW, Cojocaru I, Becker J, Fedotov IV, Alkahtani MHA, Alajlan A, et al. Germanium-vacancy color center in diamond as a temperature sensor. ACS Photon (2018) 5:765–70. doi:10.1021/acsphotonics.7b01465
76. Widmann M, Lee SY, Rendler T, Son NT, Fedder H, Paik S, et al. Coherent control of single spins in silicon carbide at room temperature. Nat Mater (2015) 14:164–8. doi:10.1038/nmat4145
77. Anisimov AN, Simin D, Soltamov VA, Lebedev SP, Baranov PG, Astakhov GV, et al. Optical thermometry based on level anticrossing in silicon carbide. Sci Rep (2016) 6:33301. doi:10.1038/srep33301
78. Christle DJ, Klimov PV, de las Casas CF, Szász K, Ivády V, Jokubavicius V, et al. Isolated spin qubits in SiC with a high-fidelity infrared spin-to-photon interface. Phys Rev X (2017) 7:021046. doi:10.1103/physrevx.7.021046
79. Lin WX, Yan FF, Li Q, Wang J, Hao ZH, Zhou JY, et al. Temperature dependence of divacancy spin coherence in implanted silicon carbide. Phys Rev B (2021) 104:125305. doi:10.1103/physrevb.104.125305
80. Gottscholl A, Kianinia M, Soltamov V, Orlinskii S, Mamin G, Bradac C, et al. Initialization and read-out of intrinsic spin defects in a van Der Waals crystal at room temperature. Nat Mater (2020) 19:540–5. doi:10.1038/s41563-020-0619-6
81. Liu W, Li ZP, Yang YZ, Yu S, Meng Y, Wang ZA, et al. Temperature-dependent energy-level shifts of spin defects in hexagonal boron nitride. ACS Photon (2021) 8:1889–95. doi:10.1021/acsphotonics.1c00320
Keywords: quantum sensing, NV center in diamond, hybrid sensing, spin dynamics, multi-modal sensing, nanoscale sensing
Citation: Wang N and Cai J (2024) Hybrid quantum sensing in diamond. Front. Phys. 12:1320108. doi: 10.3389/fphy.2024.1320108
Received: 11 October 2023; Accepted: 04 January 2024;
Published: 14 February 2024.
Edited by:
Panyu Hou, Tsinghua University, ChinaReviewed by:
Lu Yanan, Tsinghua University, ChinaGuanghui He, Washington University in St. Louis, United States, in collaboration with reviewer LY
Copyright © 2024 Wang and Cai. This is an open-access article distributed under the terms of the Creative Commons Attribution License (CC BY). The use, distribution or reproduction in other forums is permitted, provided the original author(s) and the copyright owner(s) are credited and that the original publication in this journal is cited, in accordance with accepted academic practice. No use, distribution or reproduction is permitted which does not comply with these terms.
*Correspondence: Ning Wang, bmluZ3dhbmdAaHVzdC5lZHUuY24=; Jianming Cai, amlhbm1pbmdjYWlAaHVzdC5lZHUuY24=