- 1Unidad de Bioquímica y Biología Molecular de Plantas, Centro de Investigación Científica de Yucatán, Mérida, Mexico
- 2CONACYT, Instituto Tecnológico Nacional de México, Instituto Tecnológico de Tuxtla Gutiérrez, Tuxtla Gutiérrez, Mexico
- 3CONACYT, Laboratorio de Análisis y Diagnóstico del Patrimonio, Colegio de Michoacán, Zamora, Mexico
- 4Centro de Biotecnología y Genómica de Plantas, Universidad Politécnica de Madrid, Madrid, Spain
High-affinity K+ (HAK) transporters are encoded by a large family of genes and are ubiquitous in the plant kingdom. These HAK-type transporters participate in low- and high-affinity potassium (K+) uptake and are crucial for the maintenance of K+ homeostasis under hostile conditions. In this study, the full-length cDNA of CcHAK1 gene was isolated from roots of the habanero pepper (Capsicum chinense). CcHAK1 expression was positively regulated by K+ starvation in roots and was not inhibited in the presence of NaCl. Phylogenetic analysis placed the CcHAK1 transporter in group I of the HAK K+ transporters, showing that it is closely related to Capsicum annuum CaHAK1 and Solanum lycopersicum LeHAK5. Characterization of the protein in a yeast mutant deficient in high-affinity K+ uptake (WΔ3) suggested that CcHAK1 function is associated with high-affinity K+ uptake, with Km and Vmax for Rb of 50 μM and 0.52 nmol mg−1 min−1, respectively. K+ uptake in yeast expressing the CcHAK1 transporter was inhibited by millimolar concentrations of the cations ammonium () and cesium (Cs+) but not by sodium (Na+). The results presented in this study suggest that the CcHAK1 transporter may contribute to the maintenance of K+ homeostasis in root cells in C. chinense plants undergoing K+-deficiency and salt stress.
Introduction
Plants require a variety of mineral nutrients throughout their ontogeny to complete their growth and development (Marschner, 2012). Potassium (K+) is one of the most important essential macronutrients and the most abundant inorganic cation in plant cells. In plants, K+ represents 2–10% of the dry biomass and has a crucial role in many physiological and developmental processes, including adaptation to hostile conditions (Maathuis, 2009; Wang et al., 2013; Shabala and Pottosin, 2014). Despite the abundance of K+ in the earth's crust (2.1%), its low availability in the soil limits vegetal growth and reduces the productivity of large areas of arable land (Benito et al., 2014; Zörb et al., 2014). As a result, K+ fertilization has become a common and necessary practice in agriculture. However, such fertilization is very expensive, and a great proportion of the added K+ is lost by lixiviation (Römheld and Kirkby, 2010; Zörb et al., 2014).
The typical K+ concentration in the soil solution oscillates between 0.1 and 1 mM (Maathuis, 2009), but the concentration of available K+ is much lower. Consequently, plants have developed a variety of strategies, including symbiosis with microorganisms and specific K+ transport systems, to permit them to survive under conditions of low K+ availability (Garcia and Zimmermann, 2014; Nieves-Cordones et al., 2014). Potassium uptake in roots exhibits biphasic kinetics depending on the external concentration of K+, with components of low and high affinity (Epstein et al., 1963). High-affinity K+ uptake is an active process that is mediated primarily by transporters and passively by AKT1 (Arabidopsis K+ transporter 1) channel (Rubio et al., 2008; Pyo et al., 2010); whereas low-affinity K+ uptake is a passive process that occurs through membrane channels. The proteins that participate in the K+ transport and distribution processes in plants include AKT1 channels, high-affinity K+ transporters 2 (HKT2), cation/proton (H+) antiporters (CHX), and high-affinity K+ (HAK) symporters (Rodríguez-Navarro and Rubio, 2006; Rubio et al., 2010; Alemán et al., 2011; Ahmad and Maathuis, 2014; Chérel et al., 2014; Nieves-Cordones et al., 2014; Véry et al., 2014).
K+ uptake permeases/high-affinity K+ transporters/K+ transporters (KUP/HAK/KT) are represented by a large family of genes present in all biological kingdoms except Animalia; they are even found in viruses (Grabov, 2007; Greiner et al., 2011; Véry et al., 2014). HAK genes are present in all plant genomes studied to date, indicating the likely importance of these transporters in the sessile life style of plants (Rubio et al., 2000; Grabov, 2007; Véry et al., 2014). However, the number of HAK genes varies among species. At present, 13 such genes have been identified in Arabidopsis thaliana (Maser et al., 2001), 27 in Oryza sativa (Gupta et al., 2008; Yang et al., 2009), 27 in Zea mays (Zhang et al., 2012), 31 in Populus trichocarpa (He et al., 2012), 19 in Solanum lycopersicum (Nieves-Cordones et al., 2007; Hyun et al., 2014), and 16 in Prunus persica (Song Z. Z. et al., 2015). In addition, some members of the HAK gene family have been identified in Capsicum annuum (CaHAK1, Martínez-Cordero et al., 2004), Hordeum vulgare (HvHAK1-4, Santa-María et al., 1997; Vallejo et al., 2005; Boscari et al., 2009), Lotus japonicus (LjKUP, Desbrosses et al., 2004), Vitis vinifera (VvKUP1-2, Davies et al., 2006), Gossypium hirsutum (GhKT1, Ruan et al., 2001), in halophytes such as Mesembryanthemum crystallinum (McHAK1-4, Su et al., 2002), Cymodocea nodosa (CnHAK1-2, Garciadeblás et al., 2002), Thellungiella halophila (ThHAK5, Alemán et al., 2009), Phragmites australis (PhaHAK1-2,5, Takahashi et al., 2007a,b), Aeluropus littoralis (AlHAK, Su et al., 2007), and in the Cryptomeria japonica conifer (CjKUP1, Hosoo et al., 2014).
The transporters encoded by genes of the HAK family can be grouped into four (I–IV) phylogenetic groups (Rubio et al., 2000). At the transcriptional level, the members of this family are expressed in different tissues and organs (Su et al., 2002; Ahn et al., 2004; Yang et al., 2014; Song Z. et al., 2015) and are regulated by physiological conditions and environmental factors in a differential manner according to the group to which they belong (Véry et al., 2014; Song Z. et al., 2015). In particular, the genes that encode the HAK transporters of group I are positively regulated in roots under K+ starvation (Santa-María et al., 1997; Bañuelos et al., 2002; Ahn et al., 2004; Martínez-Cordero et al., 2004; Nieves-Cordones et al., 2007; Alemán et al., 2009; Horie et al., 2011; Shen et al., 2015), suggesting an adaptive role of group I transporters under conditions of low K+ availability. The positive regulation of the HAK genes by K+ deficiency can be inhibited by the presence of various ions. It has been reported that the cation sodium (Na+) prevents positive regulation by K+ starvation of LeHAK5, AtHAK5, and ThHAK5 (Nieves-Cordones et al., 2007; Alemán et al., 2009), whereas the expression of HvHAK1, OsHAK5, OsHAK21, and some members of group II such as McHAK1, McHAK3, and PhaHAK2 increases under these conditions (Su et al., 2002; Takahashi et al., 2007a; Fulgenzi et al., 2008; Yang et al., 2014; Shen et al., 2015). Ammonium () and cesium (Cs+) can induce the expression of HAK-type genes, suggesting the participation of HAK-type gene products in their transport and/or an effect of and Cs+ on K+ levels through competitive inhibition (Nieves-Cordones et al., 2007; Qi et al., 2008; ten-Hoopen et al., 2010). Hormones such as abscisic acid (ABA) and ethylene, in addition to other factors such as membrane potential, osmotic stress, reactive oxygen species (ROS), and the processes of growth and stages of vegetal development of the plant, also regulate the expression of the HAK genes (see Véry et al., 2014).
The study of HAK transporters in heterologous systems such as bacteria, yeast and insect cells has provided crucial information on the function, selectivity, and affinity of the transport mediated by these proteins (Haro and Rodríguez-Navarro, 2003; Alemán et al., 2011). For example the expression in yeast mutants has shown that some members of HAK transporters that belong to group I display high affinity for K+ and poor discrimination between K+, Cs+, and rubidium (Rb+), and are inhibited by and Na+ (Rubio et al., 2000; Martínez-Cordero et al., 2004; Alemán et al., 2009), whereas those of group II displayed high or low affinity for K+ and can even show dual affinities. The transporters of groups III and IV have been poorly studied, and their function as transporters is less well understood (Véry et al., 2014). Furthermore, HAK-type proteins mediates the transport of Cs+ under conditions of low K+ availability and to be inhibited by (without transporting this cation), negatively correlating with the K+ uptake and content of cells (Santa-María et al., 2000; Nieves-Cordones et al., 2007; Qi et al., 2008; ten-Hoopen et al., 2010; Adams et al., 2013).
Recently, the participation of HAK transporters of group I in the maintenance of K+ homeostasis under hostile conditions has been reported (Nieves-Cordones et al., 2010, 2014; Alemán et al., 2014; Chérel et al., 2014; Yang et al., 2014; Shen et al., 2015). Salinity can induce K+ deficiency by inhibiting influx and increasing K+ efflux in roots, resulting in decreased K+ content of the plant (Bojórquez-Quintal et al., 2014; Demidchik, 2014; Shabala and Pottosin, 2014). In these adverse environmental conditions, the existence of a Na+-insensitive K+ uptake system in plant roots would undoubtedly be a useful strategy to maintain a high cytosolic K+/Na+ ratio crucial for salt tolerance (Shabala and Cuin, 2008). So far, only few candidates have been described like it is the rice OSHAK5 transporter. Its expression in the bright yellow 2 (BY2) tobacco cell line has demonstrated to increase the salinity tolerance of the cells (Horie et al., 2011), and overexpression of the same transporter in rice increased the K+/Na+ ratio and salt stress tolerance, suggesting the maintenance of high-affinity K+ uptake in the presence of Na+ (Yang et al., 2014). Also, AtHAK5 and OsHAK21 plays an important role in the absorption of K+ under conditions of K+ deficiency and high levels of Na+ (Nieves-Cordones et al., 2010; Shen et al., 2015).
Capsicum chinense (habanero pepper) is a species of pepper that is in great demand in Mexico and other countries due to its flavor, pungency, diversity in shape and fruit color (Bojórquez-Quintal et al., 2014). The production of habanero pepper fruits is directly related to K+ availability, and addition of this nutrient to the soil solution is necessary for their successful cultivation (Monforte-Gonzalez et al., 2010). However, the K+ fertilization seems not required for flowering, possibly due to the existence of efficient transport systems of K+ operating in this plant (Medina-Lara et al., 2008). In general, habanero pepper plants are cultivated in K+-rich soils, but a great proportion of this K+ is not available in the soil solution and cannot be absorbed by the plants (Borges-Gómez et al., 2005). Also, salinity problems could arise in these soils (Delgado et al., 2010) and induce K+ deficiency. Recent studies have suggested the presence of high- and low-affinity K+ transport mechanisms in the roots of the habanero pepper plants (Pacheco-Arjona et al., 2011). However, there is no information on the proteins that participate in K+ uptake in this species under conditions of K+ deficiency. In this work, we report the cDNA cloning of a HAK-type gene that is expressed in C. chinense roots. This gene, CcHAK1, is positively regulated by K+ deficiency and encodes a high-affinity K+ transporter (CcHAK1). Characterization of this transporter in Saccharomyces cerevisiae indicated that it mediates K+ uptake in the micromolar range and is insensitive to Na+.
Materials and Methods
Plant Material and Growth Conditions
Habanero pepper (C. chinense Jacq.) seeds (Chichen-Itza cultivar) (Seminis Vegetable Seeds, Inc., 2700 Camino del Sol, Oxnard, CA 93030, USA) were used in this study. The seeds were surface-sterilized with 80% ethanol (v/v) and sodium hypochlorite (30%, v/v, Cloralex™, North Alen, SA de CV) as described in Celis-Arámburo et al. (2011), washed with sterile water, pre-hydrated for 72 h in the dark at 4°C, and germinated by placing them in Petri dishes containing filter paper moistened with sterile grade milli-Q water. After germination, the seeds were transferred to containers with sterile vermiculite moistened with sterile milli-Q water, and the seedlings were grown for 45 days in a growth room at 25°C under a photoperiod cycle of 16/8 h light/dark and a light intensity of 123 μmol m−2 s−1. The seedlings were watered with sterile milli-Q water until the emergence of cotyledonal leaves (~15 days); then, a modified Hoagland nutrient solution (1/5 of the typical ionic strength) that contained the following micronutrients (μM) was applied: 50 CaCl2, 12.5 H3BO3, 1 MnSO4, 1 ZnSO4, 0.5 CuSO4, 0.1 (NH4)6Mo7O24, 0.1 NiCl, 10 Fe-EDTA, and macronutrients (mM): 1.2 KNO3, 0.8 Ca(NO3)2, 0.2 KH2PO4, 0.2 MgSO4. The nutrient solution was replaced with fresh solution each week until 45 days of plants growth.
Potassium starvation experiments were conducted under hydroponic conditions; 45-day-old seedlings, at which the vermiculite was removed, were transferred to a nutrient solution lacking K+ but otherwise containing the micronutrients described above. The macronutrient concentrations were modified to the following concentrations (in mM): 0.1 Ca(H2PO4)2, 1.4 Ca(NO3)2, 0.2 MgSO4, without K+. The nutrient solution was replaced twice during the experiment. Roots from seedlings growing in the absence of K+ were harvested at 15-days, quickly frozen in liquid nitrogen and stored at −80°C until total RNA extraction was performed.
Isolation, Sequence Analysis of CcHAK1 cDNA, and Phylogenetic Tree
Total RNA was isolated from root tissue using a NucleoSpin RNA Plant Kit (Macherey-Nagel, Neumann-Neander Str. 6–8, Düren, Germany). cDNA was synthesized from 1 μg of total RNA using commercial avian myeloblastosis virus reverse transcriptase (First-strand cDNA Synthesis Kit, Amersahm Biosciences, Freiburg Germany) with an anchored oligo-dT primer according to the manufacturer's instructions. To obtain an initial fragment containing the habanero pepper HAK1 gene, the reverse transcription products were amplified by the polymerase chain reaction (PCR) using Taq high-fidelity polymerase (Roche) and degenerate primers previously described by Martínez-Cordero et al. (2004) to isolate a HAK1 gene from C. annuum. The PCR product was cloned into the pCR2.1-TOPO vector using a TA Cloning Kit (Invitrogen, Carlsbad, CA, USA) and sequenced. The missing portions of the cDNA at the 5′ and 3′ ends were rescued by rapid amplification of 5′ and 3′ cDNA ends (5′/3′ RACE kit 2nd generation, Roche, Mannheim, Germany) following the manufacturer's instructions. The full-length cDNA, which was designated CcHAK1, was obtained after PCR using the sense primer 5′-GTCTAGAAAACAATGGCTAGCTCAGATAGTGAT-3′ and the antisense primer 5′-CGAATTCGTTATACCTCATAAGTCATGCCAACC-3′ that included the initiation codon ATG and a stop codon, respectively. These primers included Xba I and EcoR I restriction sites appropriate for insertion of CcHAK1 in the sense orientation into a yeast expression vector. The sequence of the primer containing the initiation codon ATG was modified according to Hamilton et al. (1987) and Haro et al. (2013) to enhance protein expression in yeast. PCR products were cloned into the pCR2.1-TOPO vector using a TA Cloning Kit (Invitrogen) and verified by sequencing.
Protein and nucleotide alignments were obtained using the Clustal W2 program (http://www.ebi.ac.uk) and BLAST (http://www.ncbi.nlm.nih.gov/BLAST/) in tblastp form. The phylogenetic tree was generated using the neighbor-joining method with MEGA software. The molecular weight was determined by the program Compute Pi/Mw tool (http://expasy.org/). Hydrophobicity parameters were calculated by TopPred-Topology prediction of membrane protein (http://mobyle.pasteur.fr/cgi-bin/portal.py?=toppred), TMpred (http://www.ch.embnet.org/software/TMPRED_form.html), TMHMM (http://www.cbs.dtu.dk/services/TMHMM/). A hypothetical model for the membrane topology of the protein encoded by the amplified cDNA (CcHAK1) was built using the TMHMM server (http://www.cbs.dtu.dk/services/TMHMM/).
Functional Complementation of CcHAK1 in the Yeast Saccharomyces cerevisiae
The pCR2.1-TOPO + CcHAK1 cDNA construct was digested with Xba I and EcoR I restriction enzymes, and a 2421-bp fragment containing the CcHAK1 open reading frame (ORF, 2415 bp in length) plus 5 bp at the 5′-proximal ATG codon and 1 bp at the 3′-terminal STOP codon (AAACA and C, respectively, to enhance protein expression in yeast) was ligated into the yeast expression vector pYPGE15 (Brunelli and Pall, 1993) to generate the construct pYPGE15+CcHAK1. For functional complementation experiments, pYPGE15 (the parent plasmid) and pYPGE15+CcHAK1 were transformed into K+ uptake- deficient yeast (S. cerevisiae) strain WΔ3 (MATa, ade2, ura3, trp1, trk1Δ::LEU2 trk2Δ::HIS3; Haro et al., 1999; Haro and Rodríguez-Navarro, 2003). The yeast strains transformed with the plasmid pYPGE15 containing the CcHAK1 cDNA and with the empty plasmid were designated as WΔ3-CcHAK1 and WΔ3-p, respectively. The WΔ3 strains were maintained in yeast extract-peptone-dextrose (YPD) medium and minimal medium SD (Sherman, 1991) supplemented with uracil (appropriate nutritional requirements according to the auxotrophic markers) and 50 mM K+.
Complementation assays growth of yeast at low K+ were performed in Petri dishes containing solid arginine phosphate medium lacking uracil (AP-U) (Rodríguez-Navarro and Ramos, 1984) supplemented with concentrations of K+ ranging from 0.05 to 5 mM, in the absence or presence of 20 mM NH4Cl, 5 mM CsCl or various concentrations of NaCl (50 and 100 mM), for inhibition studies. For growth, the yeast strains were incubated at 28°C. In some cases, WΔ3-CaHAK1 (WΔ3 strain transformed with CaHAK1, the HAK1 gene of C. annuum (Martínez-Cordero et al., 2004) was used as a positive control for the yeast growth in the micromolar concentrations of K+.
Growth Curves of Yeast Transformants
The WΔ3-CcHAK1strain was grown in liquid AP-U medium at 28°C under continuous agitation and supplemented with various concentrations of K+ (0.05, 0.1, and 5 mM K+) or with 0.1 mM K+ plus 10 mM NaCl. A Bioscreen C Microbiology Reader (OY Growth Curve AB Ltd., Helsinki, Finland) was used to measure the optical densities of the cultures every 3 h for 3 consecutive days of growth at 28°C in continuous agitation. In this assay, the WΔ3-CaHAK1 strain was used as a positive control. The experiment was repeated thrice.
Cation Uptake Experiments in Yeast
To evaluate the K+ transport capacity of CcHAK1 and to determine the values of Km and Vmax, two tests were performed: measurement of K+ depletion from the culture medium and measurement of cation accumulation in the cells. For both assays, yeast cells were grown overnight at 28°C in AP-U medium (supplemented with 50 mM K+ for WΔ3-pYPGE15 and WΔ3-p) and then starved of K+ for 4 h in K+-free AP-U medium. The cells were then suspended in 10 mM MES supplemented with 2% glucose and adjusted to pH 6 with Ca(OH)2. At time zero, the indicated concentrations of cations (KCl or RbCl) were added to the medium and the samples were collected at intervals over a 2-h period. The experiment was repeated thrice.
For K+ depletion experiments, the cells were suspended in AP-U medium containing various micromolar concentrations of K+ plus 1 mM CsCl, 1 mM NH4Cl, or 5 mM NaCl for inhibition studies. Samples of the medium (1 ml) were removed at various time intervals and centrifuged at 5000 rpm for 1 min, and the K+ concentration in the supernatant was measured. For K+ uptake assays, 5- or 10-ml samples were taken at intervals, filtered through an AAWP nitrocellulose membrane filter (0.8-μm pore, Millipore, Molsheim, France) and washed with 20 mM MgCl2. The filters were incubated overnight in 0.1 M HCl and 10 mM MgCl2. The results are expressed on a cell dry weight basis. Data from three independent experiments for each condition were fitted to the Michaelis-Menten equation. Cations were identified and quantified by atomic emission spectrophotometry using a Perkin-Elmer Model 2380 spectrophotometer (Norwalk, CT, USA; Fraile-Escanciano et al., 2010). Control experiments were performed with the WΔ3 strain transformed with plasmid without an insert (WΔ3-p).
Analysis of Transcript Levels of CcHAK1 by RT-PCR
To evaluate CcHAK1 expression, 45-day-old habanero pepper seedlings were transferred to a modified Hoagland nutrient solution containing 1.4 mM K+ (KCl, control) or 50 μM K+ (deficit) with 0, 10, 30, or 50 mM NaCl for 10 days. Semi-quantitative PCR was performed using cDNA synthesized from 1 μg of total RNA isolated as described above. For PCR, we used sense (5′-TACAACAACAAGTGGATTCAAG-3′) and antisense (5′-CGAATTCGTTATACCTCATAAGTCATGCCAACC-3′) primers designed based on the total cDNA sequence from CcHAK1. PCR was conducted after a 5 min denaturation step at 95°C followed by 25, 28, 30, or 35 cycles of 30 s at 95°C, 30 s at 55°C, and 40 s at 72°C. Tubulin served as a positive control in the reaction with primers forward (5′-GACCTTGAATCGGCTTATGG-3′) and reverse (5′ TATCCTGGGTGAACGCTTTG 3′). RT-PCR was performed with two different RNA extracts from leaves and roots tissues of each treatment. PCR was repeated three times using Taq polymerase (Sigma).
Statistical Analysis
To yeast growth curves and cation uptake experiments the results are representative of three independent experiments. Date are subjected to analysis of variance (ANOVA) and mean comparisons were made using Tukey's multiple range test (P ≤ 0.05), using SIGMA STAT v.12.
Results
Isolation and Sequence Analysis of CcHAK1 cDNA
Total RNA was isolated from the roots of C. chinense seedlings exposed to K+ starvation for 15 days to identify putative K+ transporters that can be expressed in this growth conditions. Using degenerated primers designed from HAK transporters by Martínez-Cordero et al. (2004), a fragment an 843-bp region was initially amplified. The full-length cDNA was obtained by extension of the 5′ and 3′ ends using the 5′/3′ RACE Kit. The final clone obtained had an insert of 2415 bp that contained the CcHAK1.
In silico analysis of the CcHAK1 sequence revealed that it encodes a polypeptide of 804 amino acids with a predictive molecular mass of 89.86 kDa (Figures S1A, S2). The CcHAK1 putative protein showed characteristic conserved regions, such as the GVIYGDIGTSPLY sequence (the conserved amino acids are shown in bold), common to transporters of the KUP/HAK/KT family (Figures S1A, S2; Rubio et al., 2000). Hydrophobicity analysis of the CcHAK1 sequence predicted the presence of 12 transmembrane regions and a long carboxyl-terminal tail (Figure S1). Alignment of the CcHAK1 sequence with the sequence of other transporters (ClustalW2) gave 99% similarity with CaHAK1 and 85% with SlHAK5. Furthermore, a difference of only eight amino acids between the CcHAK1 and CaHAK1 sequences was observed (Figure S2). Phylogenetic analysis placed CcHAK1 protein in group I of the K+ transporters, close to CaHAK1 and SlHAK5 (Figure 1). Interestingly, unlike most HAK transporters of group I (Figure 1), the CcHAK1 sequence shows a change in only one amino acid at the N356T position (Figure S2), where there is a threonine (T) instead of an asparagine (N).
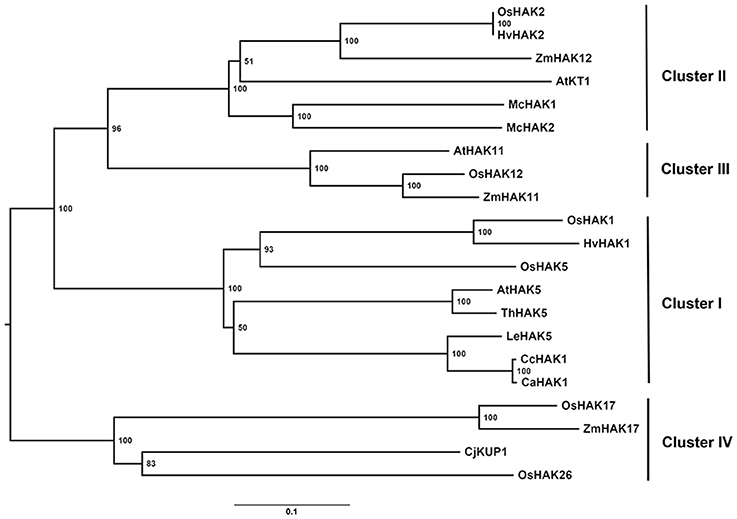
Figure 1. Phylogenetic tree of the proteins of the KUP/HAK/KT transporter family. The most representative members of each group of HAK transporters and the CcHAK1 transporter were used in the phylogenetic study. The tree was constructed using the nearest-neighbor algorithm with MEGA software, and the bookstrap values from 1000 replicates are shown at each node. The accession numbers are as follows: AtHAK5 (AF129478), AtHAK11 (BT002147.1), AtKT1 (AF012656.1), CaHAK1 (AY560009), CcHAK1 (KT202302), CjKUP1 (AB915694), HvHAK1 (AF025292), HvHAK2 (AF129479.1), LeHAK5 (DQ489721), McHAK1 (AF367864.1), McHAK2 (AF367865.1), OsHAK1 (AJ427970), OsHAK2 (AK070575), OsHAK5 (AK241580), OsHAK12 (AJ427981.1), OsHAK17 (AJ427975.1), OsHAK26 (AK072472), ThHAK5 (EF177193), ZmHAK11 (DAA36040.1), ZmHAK12 (AFW56980.1), ZmHAK17 (DAA61709.1).
CcHAK1 Encodes a High-Affinity K+ Transporter
All HAK-type transporters of group I that have been characterized to date are regulated by the absence of K+ in the medium and show a high affinity for the cation, especially when the availability of external K+ is low, with Km values (Rb+) in the micromolar range (Rodríguez-Navarro and Rubio, 2006; Grabov, 2007; Véry et al., 2014). To determine whether CcHAK1 functions as a K+ transporter, the CcHAK1 cDNA was cloned in the yeast expression vector pYPGE15, and the construction was transformed into the WΔ3 yeast strain, which is deficient in high-affinity K+ uptake systems (Haro and Rodríguez-Navarro, 2003). In the presence of 5 mM K+, the growth of the WΔ3-CcHAK1 transformants were similar to that of the yeast strain transformed with the empty vector (WΔ3-p; Figure 2A). However, at 300 and 100 μM, only the expression of CcHAK1 could restored the growth of the WΔ3 mutant. In contrast, growth of the mutant transformed with the empty plasmid (WΔ3-p) under the same conditions was not observed (Figure 2A). These results suggest that CcHAK1 is involved in high-affinity K+ transport.
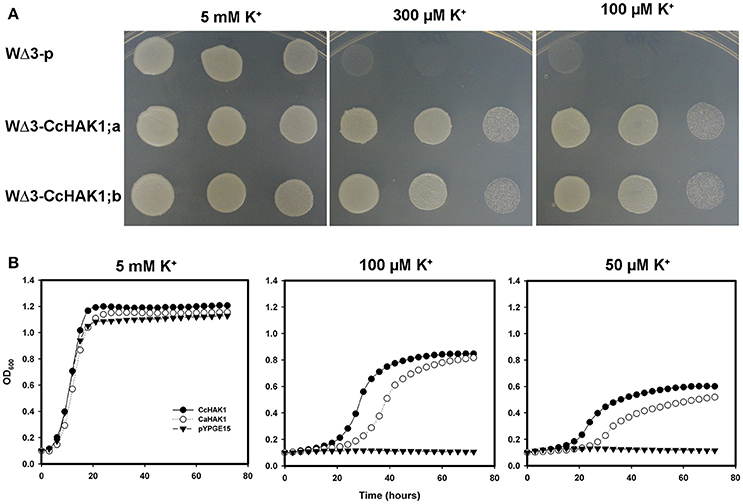
Figure 2. CcHAK1 complementation assay in yeast cells deficient in high-affinity K+ uptake. (A) Growth of the mutant strain of WΔ3 yeast in solid arginine phosphate medium lacking uracil (AP-U) supplemented with various concentrations of K+. The mutant yeast strain was transformed with the empty plasmid pYPGE15 (WΔ3-p) or with the plasmid containing the CcHAK1 cDNA (WΔ3-CcHAK1;a and WΔ3-CcHAK1;b, independent clones). Drop serial dilutions of the cell culture were inoculated on agar plates containing AP-U medium. (B) Growth curves of the WΔ3 strain transformed with the empty plasmid (closed triangles), CcHAK1 (closed circles), or CaHAK1 (open circles) in liquid medium (AP-U) supplemented with 5 mM, 100 μM, or 50 μM K+.
For a more precise study of the growth recovery capacity of the WΔ3 mutants, growth curves of the cells in liquid arginine phosphate lacking uracil (AP-U) medium at various concentrations of K+ were performed (Figure 2B). For this study, the growth of transformants expressing CcHAK1 and its C. annuum CaHAK1 counterpart were compared (Martínez-Cordero et al., 2004). At 5 mM K+, the three transformed strains showed similar growth. However, WΔ3-p failed to grow at micromolar concentrations of K+. On the other hand, the transformants expressing the HAK1 transporter grew even in the presence of 50 μM K+. These results indicate that CcHAK1 expression enables growth of the WΔ3 mutant at micromolar concentrations of K+, suggesting that it confers high-affinity transport of K+ like its previously characterized CaHAK1 counterpart (Martínez-Cordero et al., 2004).
To confirm that CcHAK1 is a K+ transporter, a kinetic study of the depletion of K+ from the medium was performed (Figure 3A). The WΔ3-CcHAK1 yeasts depleted the K+ present in the external medium (25 μM K+) after 60 min. No depletion of K+ was observed in medium containing the WΔ3-p strain, indicating that the observed K+ uptake from the WΔ3-CcHAK1 medium was due to the expression of CcHAK1 (Figure 3A). Kinetic characterization of the transport mediated by CcHAK1 was carried out using Rb+ in the absorption experiments. The Rb+ is a K+ analogue that is commonly used in kinetic analyses; CcHAK1 does not discriminate between these two cations (data not shown). CcHAK1 mediates high-affinity Rb+ uptake with an apparent Km of 50 μM and a Vmax of 0.52 nmol mg−1 min−1 (Figure 3B).
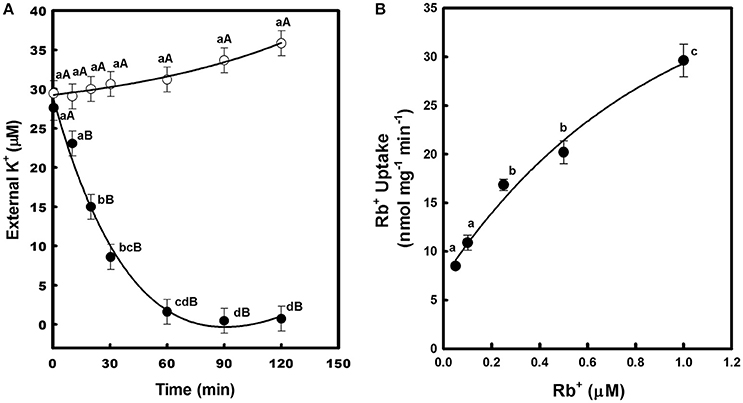
Figure 3. Depletion of external K+ and kinetics of Rb+ uptake in cell suspensions of yeast expressing the CcHAK1 transporter. (A) K+ uptake in yeast. WΔ3 strains transformed with CcHAK1 cDNA (WΔ3-CcHAK1, closed circles) or with the empty plasmid (WΔ3-p, open circles) were subjected to K+ starvation for 4 h. At time zero, 25 μM K+ (KCl) was added to the suspension solution, which consisted of 10 mM MES pH 6, supplemented with glucose at 2%. The concentration of K+ was measured in the medium at intervals over a 2-h period. (B) Rb+ uptake in yeast. Rb+ adsorption values at various external concentrations of Rb+ are shown. The data were fitted to the Michaelis–Menten equation; a Km of 50 μM and a Vmax of 0.52 nmol mg−1 min−1 for Rb+ were calculated. The WΔ3 strain transformed with the CcHAK1 cDNA was subjected to K+ starvation for 4 h and suspended in 10 mM MES at pH 6 for Rb+ uptake experiments. Figures show the data of a representative experiment of three repetitions. Data are mean ± SE of three replicates. Different lowercase letters represent significant differences (p ≤ 0.05; Tukey's test) within a strain between time points (A) or Rb+ concentrations (B), while different capital letters (A) represent significant differences (p ≤ 0.05; Tukey's test) between strains within the same day.
Effects of , Cs+, and Na+ on the Transport of K+
To determine the effect of , Cs+, and Na+ on the transport of high-affinity K+, drop complementation assays and K+ depletion studies were performed. Growth of the WΔ3-CcHAK1 strain was inhibited when and Cs+ were added to the culture medium (AP-U). In contrast, the growth of CcHAK1 transformants was insensitive to Na+ (Figure 4A). WΔ3 yeast cells expressing CcHAK1 cDNA were capable of depleting the external K+ in the culture medium after 90 min under conditions of absence. The presence of 1 mM NH4Cl inhibited the transport of K+ (Figure 4B). Similar results were observed using 1 mM CsCl (Figure 4C). An increase in external K+ concentration was observed for WΔ3-CcHAK1 after 60 min of treatment with (Figure 4A) and Cs+ (Figure 4C).
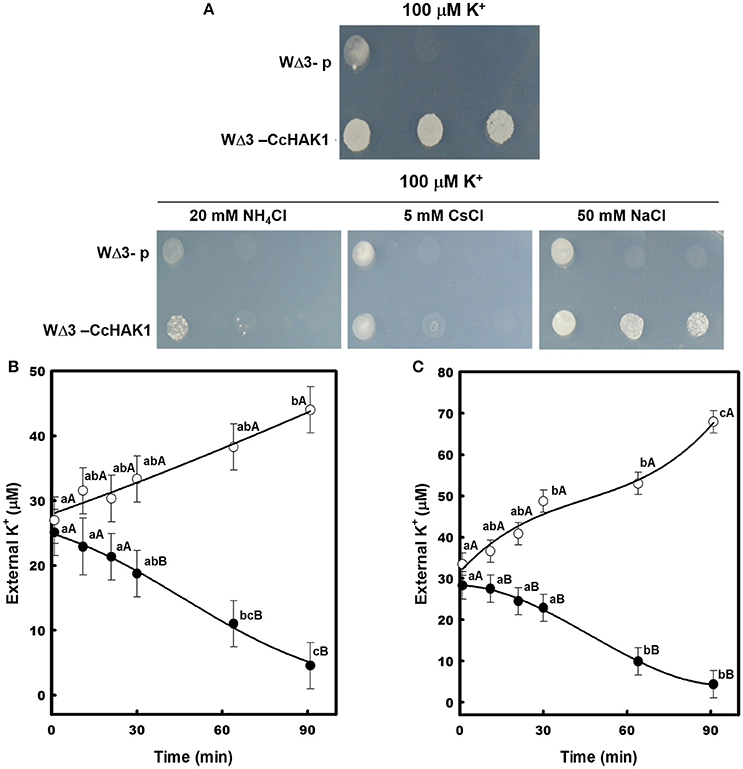
Figure 4. Effects of NH4Cl, CsCl, and NaCl on the high-affinity K+ uptake mediated by CcHAK1 in the yeast mutant. (A) Growth of yeast transformed with CcHAK1 cDNA (WΔ3-CcHAK1) in the presence of NH4Cl, CsCl, and NaCl. WΔ3-p: empty plasmid. (B) K+ uptake in the presence (open circles) and absence (closed circles) of 1 mM NH4Cl. (C) High-affinity K+ uptake in the presence (open circles) and absence (closed circles) of 1 mM CsCl. The WΔ3 strain transformed with CcHAK1 cDNA was subjected to K+ starvation for 4 h prior to the beginning of the experiment. The depletion of K+ was measured at intervals over a 90-min period. One representative experiment (of three) is shown. Figures show the data of a representative experiment of three repetitions. Data are mean ± SE of three replicates. Different lowercase letters represent significant differences (p ≤ 0.05; Tukey's test) within a treatment between times, while different capital letters represent significant differences (p ≤ 0.05; Tukey's test) between treatments within the same day.
To corroborate the insensitivity to Na+ of the K+ transport observed in the WΔ3-CcHAK1 transformants, a drop complementation assay was performed in which the growth of the CaHAK1 transformant sensitive to Na+ (Martínez-Cordero et al., 2004) was compared with that of the CcHAK1 transformant (Figure 5). AP-U medium containing 50 μM KCl was supplemented with various concentrations of NaCl. The WΔ3-CcHAK1 yeast strain grew in the presence of 50 and 100 mM NaCl, whereas growth of the CaHAK1-transformed strains and the empty plasmid were inhibited in the presence of NaCl. The latter strain was incapable of growing at 50 μM K+. All transformants showed similar growth at 5 mM K+ (Figure 5A).
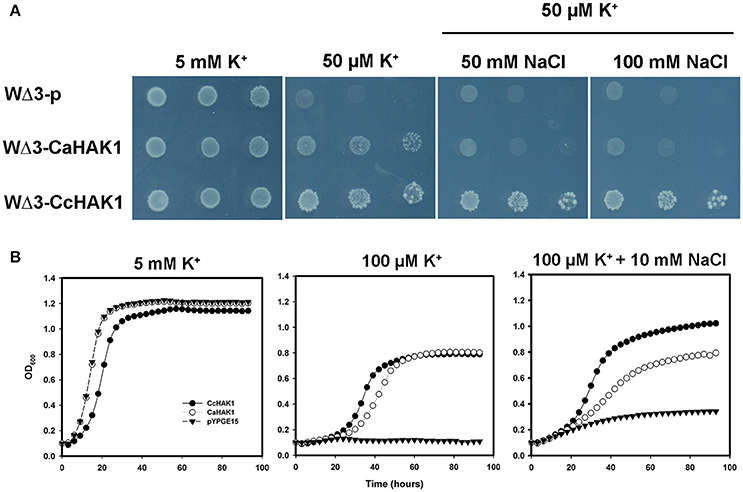
Figure 5. Growth of yeast transformed with CcHAK1 (WΔ3-CcHAK1) or CaHAK1 (WΔ3-CaHAK1) cDNA in the presence of NaCl. (A) Drop complementation assay in solid arginine phosphate medium lacking uracil (AP-U) supplemented with 50 μM K+ and various concentrations of NaCl. WΔ3-p: transformant harboring the empty plasmid. (B) Growth curves of the WΔ3 strain transformed with the empty pYPGE15 plasmid (closed triangles), CcHAK1 (closed circles), or CaHAK1 (open circles) in liquid medium (AP-U) supplemented with 100 μM K+ in the presence or absence of 10 mM NaCl. As a control for optical density, WΔ3 cells were inoculated with 5 mM K+.
The effect of Na+ on the growth of the transformants was evaluated in liquid medium (AP-U) supplemented with 100 μM K+ in the presence or absence of 10 mM NaCl (Figure 5). Unlike the WΔ3-p strain, the growth of the WΔ3-CaHAK1, and WΔ3-CcHAK1 transformants showed no inhibition by Na+ at low concentrations of K+ until 96 h. However, the growth of the yeast mutants expressing CaHAK1 was much slower than that of the WΔ3-CcHAK1 mutant (Figure 5B). The depletion of external K+ by the WΔ3-CcHAK1 strain was not inhibited by the presence of NaCl, but it displayed slower kinetics than were observed in the absence of NaCl (Figure 6).
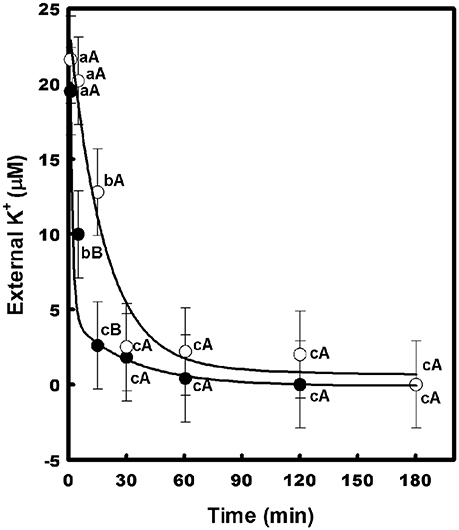
Figure 6. Effect of NaCl on the high-affinity K+ uptake mediated by CcHAK1. High-affinity K+ uptake in the presence (open circles) and absence (closed circles) of 5 mM NaCl is shown. The WΔ3 strain transformed with CcHAK1 cDNA was subjected to K+ starvation for 4 h prior to the beginning of the experiment. The depletion of K+ was measured at intervals over a 2–3 h period. Figure show the data of a representative experiment of three repetitions. Data are mean ± SE of three replicates. Different lowercase letters significant differences (p ≤ 0.05; Tukey's test) within a treatment between time points, while different capital letters represent significant differences (p ≤ 0.05; Tukey's test) between treatments within the same time.
Semi-Quantitative Expression of CcHAK1 in Pepper Roots
The expression of the HAK genes is regulated by environmental conditions, stage of vegetal development and factors such as ions, hormones, and ROS (see Véry et al., 2014). To address this question, an analysis of CcHAK1 expression in habanero pepper roots under various growth conditions was performed using semi-quantitative PCR (Figure 7). CcHAK1 transcripts were detected in normal plant growth conditions (1.4 mM K+ in the growth medium) in leaves and roots, its expression was induced mainly in the roots of seedlings exposed to K+ deprivation, and its expression was maintained even in the presence of 50 mM NaCl (Figure 7 and Figure S3).
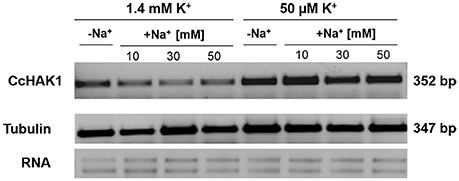
Figure 7. Effects of K+ and NaCl on CcHAK1 transcript levels. Habanero pepper seedlings 45 days old were transferred for 10 days to Hoagland solution [1/5] containing 1.4 mM or 0.05 mM K+ in the absence or presence of 10, 30, or 50 mM NaCl. The transcript levels were evaluated by RT-PCR using the tubulin gene as a loading control.
Discussion
In this study, the cDNA corresponding to the CcHAK1 gene (KT202302) was isolated from the RNA of habanero pepper roots (C. chinense) grown in the absence of K+. The CcHAK1 cDNA was 2415 bp in length. This gene encodes a HAK-type high-affinity K+ transporter of 804 amino acids (CcHAK1) with a predicted molecular mass of 89.86 KDa. CcHAK1 presents all of the structural characteristics that have previously been reported for HAK-type transporters (Figure S1), including 12 transmembrane domains, a long loop between the second and third transmembrane segments and a long carboxyl end (Rodriguez-Navarro, 2000; Gómez-Porras et al., 2012). These transporters belong to a large family of K+ transporter genes known as KUP/HAK/KT transporters that are present in non-animal cells and are ubiquitous in plants (Grabov, 2007; Greiner et al., 2011; Véry et al., 2014). Genome sequencing and molecular cloning projects have resulted in the identification of genes of the HAK family in various plant species (Santa-María et al., 1997; Maser et al., 2001; Garciadeblás et al., 2002; Su et al., 2002; Desbrosses et al., 2004; Davies et al., 2006; Takahashi et al., 2007a,b; Alemán et al., 2009; Yang et al., 2009; He et al., 2012; Zhang et al., 2012; Hosoo et al., 2014; Hyun et al., 2014; Song Z. Z. et al., 2015). In Solanaceae, 19 genes have been identified in tomato, including LeHAK5 (Nieves-Cordones et al., 2007; Hyun et al., 2014) and a member in pepper, CaHAK1 (Martínez-Cordero et al., 2004). The CcHAK1 cDNA is the second HAK-type gene discovered in peppers and the first in the C. chinense species; it shows 99% identity with CaHAK1 and 84% with LeHAK5.
The phylogenetic analysis showed that CcHAK1 belonged to group I of the HAK transporters (Rubio et al., 2000) and that it is closely related to the C. annuum CaHAK1 and the S. lycopersicum LeHAK5 (Figure 1). In addition to the high degree of homology between CcHAK1 and CaHAK1, both proteins consist of 804 amino acids, and they differ in only eight amino acid residues (Figure S2). One of these changes in amino acid residues is of particular interest because is one of the most conserved in group I of HAKs (Figure 1). CcHAK1 has a threonine (T) instead of an asparagine (N) at the 356 position (T356N) (Figure S2). Both T and N are polar, hydrophilic amino acids, but they differ in the lengths of their lateral chains. OsHAK5, which is a group I K+ transporter, has a histidine (H) at the H362N position (Figure S2). It has been reported that this HAK-type transporter shows regulatory characteristics that differ from those of other members of the group (Horie et al., 2011; Yang et al., 2014). Point mutations in the HAK transporters can modify its affinity for K+ and its sensitivity to Na+, as has been reported for HvHAK1 and AtHAK5 (Mangano et al., 2008; Alemán et al., 2014). Recent studies have identified the amino acid residues in transmembrane regions and loops of E. coli HAK transporters that are critical for K+ uptake by these proteins (Sato et al., 2014). These findings suggest that CcHAK1 could possess characteristics that differ from those of other members of group I of the HAK family. In future studies, a directed mutagenesis approach on specific amino acids in CcHAK1 should offer interesting results.
In this study, CcHAK1 function was characterized in the WΔ3 yeast strain, which is defective in high-affinity K+ uptake (Haro and Rodríguez-Navarro, 2003). CcHAK1 expression complemented the growth of the WΔ3 yeast strain and depleted the external K+ (μM) present in the medium (Figures 2, 3A). This result demonstrates that CcHAK1, mediates high-affinity K+ uptake. Expression of CcHAK1 in yeast showed that it has an apparent Km for Rb+ of 50 μM and a Vmax of 0.52 nmol mg−1 min−1 (Figure 3B). It is worth noting that the CcHAK1 Km (Rb+) is ~26-fold higher than that of its CaHAK1 homologous (1.9 μM) (Martínez-Cordero et al., 2004). The difference in the Km values suggests that C. chinense possesses low K+ uptake capacity under low K+ availability conditions. However, cultures of the WΔ3-CcHAK1 strain achieved higher optical density values than the transformants that expresses CaHAK1 at low concentrations of K+ (Figure 2B). In general, CcHAK1 has one of the highest Km (Rb+) values of the HAK-type transporters of group I that have been studied to date. Also, according to the expression analysis carried out in this study, CcHAK1 transcripts were detected in roots of plants grown in K+ normal conditions although its expression was enhanced during K+ deprivation (Figure 7). This expression pattern has been described previously in HvHAK1 (Santa-María et al., 1997) and in AtHAK5 (Rubio et al., 2000).
In roots and heterologous systems that express HAK-type proteins, high-affinity K+ uptake is inhibited by , Cs+, and Na+ (Véry et al., 2014). As has been reported for CaHAK1 (Martínez-Cordero et al., 2004), the growth of the WΔ3-CcHAK1 strain and its high-affinity K+ uptake were inhibited at millimolar concentrations of and Cs+ (Figure 4). These data agree with the results of previous studies of pepper roots in which and Cs+ were found to competitively inhibit K+ uptake at low concentrations (Martínez-Cordero et al., 2005; Pacheco-Arjona et al., 2011). In different plant species, two components of high-affinity K+ uptake have been identified: a component that is sensitive to and is mediated by HAK-type transporters and a component that is insensitive to and is mediated by AKT1-type K+ channels (Spalding et al., 1999; Santa-María et al., 2000; Martínez-Cordero et al., 2005; Nieves-Cordones et al., 2007; Pacheco-Arjona et al., 2011). Cs+ induces K+ deficiency in cells by inhibiting K+ uptake through AKT1 channels and HAK-type transporters under conditions of both high and low K+ availability (Hampton et al., 2004; Qi et al., 2008; Adams et al., 2013). In the current study, the K+ transport mediated by CcHAK1 is inhibited by both and Cs+.
The increase of K in the medium of growing for W3-CcHAK1 strain in the presence of and Cs+ can indicate K leakage from the cells. We suggested that in yeast, the uptake of these cations can causes a membrane depolarization which drives to an activation of outwardly rectifying plasma membrane potassium channel Tork1. Tork1 is the only potassium-specific efflux system described in yeast and its activity is regulated by membrane potential (Yenush, 2016).
Different responses to the Na+ effect have been reported for HAK-type transporters (Véry et al., 2014) but remarkably only a few examples, like OsHAK5, have demonstrated to be Na+-insensitive K+ uptake systems. In our work, CcHAK1 expression complemented the growth of the strain at low concentrations of K+ and in the presence of NaCl (Figure 4A) and in spite of the high sequence homology this is a functional difference between CaHAK1 and CcHAK1 (Figure 5). CaHAK1 like AtHAK5, OsHAK1, and HvHAK1 are group I transporters whose high-affinity K+ uptake is sensitive to Na+, probably due to a competitive inhibition mechanism (Rubio et al., 2000; Bañuelos et al., 2002; Martínez-Cordero et al., 2004; Fulgenzi et al., 2008). In fact, HvHAK1 and other transporters such as PhaHAK5, PhaHAK2, and OsHAK2 can mediate uptake of Na+ present at the millimolar level and inhibit K+ uptake (Santa-María et al., 1997; Takahashi et al., 2007a,b). In this study, the Na+ uptake capacity of the strain that expresses CcHAK1 was not determined, but Na+ did not interfere with K+ uptake (Figure 6). OsHAK5 is an atypical transporter that mediates K+ uptake insensitive to Na+. In E. coli and BY2 cells, the OsHAK5 transporter preferentially accumulates K+ rather than Na+ under NaCl stress (Horie et al., 2011). Similarly, CcHAK1 was shown to be Na+-insensitive; and the presence of Na+ merely made the high-affinity K+ uptake lightly slow (Figure 6). Surprisingly, in both CcHAK1 and OsHAK5, the N residue corresponding to positions 356 and 362 of the respective protein sequences is substituted with another amino acid, suggesting that this amino acid residue may participate in the regulation of the Na+ effect (Figure S2). Also, the HAK transporters of some halophytic species are insensitive to Na+, and this has been related to their tolerance of salinity (Garciadeblás et al., 2002; Su et al., 2007; Takahashi et al., 2007b). There are few studies about the effect of salinity on habanero pepper, which has been classified as a glycophyte (Niu and Rodríguez, 2010; Niu et al., 2010). Nevertheless, this specie is widely cultivated in the Yucatan coast, México, where the saline intrusion has increased, due to an intensification of the agricultural land use during recent years that has caused that soil's electrical conductivity to reach maximum values of 3.21 dS m−1 (Delgado et al., 2010).
Similar to all HAK-type genes of group I and some members of group II (Véry et al., 2014), the expression of CcHAK1 mainly in roots is regulated by K+ deficiency but it was also expressed under control conditions (Figure 7 and Figure S3). This finding suggests that the transporter that encodes CcHAK1 has an adaptive role under conditions of low K+ availability. On the other hand, the presence of Na+ in the medium can affect the expression of the genes that encode HAK-type transporters, especially under K+ deficiency conditions (Véry et al., 2014). Unlike the case with AtHAK5, LeHAK5, and ThHAK5 (Nieves-Cordones et al., 2007; Alemán et al., 2009), Na+ did not decrease CcHAK1 expression under K+ deficiency or control conditions (Figure 7). Considering the insensitivity of CcHAK1 expression to Na+, the results suggest a possible role for this protein in maintaining K+ homeostasis in root cells under saline stress. Other genes that encode HAK transporters of group II show various levels of expression in the presence of high concentrations of Na+. PhaHAK2, McHAK1, and McHAK3, which are genes of halophyte species, are positively regulated by Na+ (Su et al., 2002; Takahashi et al., 2007a). In barley, the accumulation of HvHAK1 transcripts temporarily increases in the presence of Na+ (Fulgenzi et al., 2008). However, K+ uptake through the PhaHAK2 and HvHAK1 transporters is sensitive to high concentrations of Na+ (Takahashi et al., 2007a; Fulgenzi et al., 2008; Véry et al., 2014). In rice roots, hulls and vascular tissues, the levels of OsHAK5 and OsHAK21 transcripts are positively regulated by high concentrations of NaCl under conditions of low and high K+ availability (Yang et al., 2014; Shen et al., 2015).
Leakage of K+ from root cells is a common response that occurs in the presence of NaCl due to depolarization of the plasma membrane (Demidchik et al., 2014). Also, other ROS-activated mechanisms may contribute to this K+-efflux in some species (Bose et al., 2014). This depolarization makes K+ uptake through AKT1 channels thermodynamically impossible and conditions the plants to take up K+ through HAK-type transporters (Anschütz et al., 2014; Demidchik et al., 2014). It has recently been reported that the AtHAK5, OsHAK5, and OsHAK21 transporters are required for growth and K+ uptake under NaCl stress (Nieves-Cordones et al., 2010; Yang et al., 2014; Shen et al., 2015). Overexpression of OsHAK5 in rice and BY2 cells improves the salt tolerance of the cells by increasing the K+/Na+ ratio (Horie et al., 2011; Yang et al., 2014). Capsicum is a genus that is moderately sensitive to saline stress throughout its ontogeny with a significant reduction in fruit production (Bojórquez-Quintal et al., 2012). In habanero pepper, NaCl induces K+ efflux and reduces the K+ content of roots at high salt stress (Bojórquez-Quintal et al., 2014). The results regarding CcHAK1 expression and high-affinity K+ uptake in the presence of Na+ presented in this work suggest that, in habanero peppers, continuous high-affinity K+ uptake may occur to maintain the K+/Na+ ratio under saline stress. In fact, the content of K+ in habanero pepper roots is maintained at low and moderate concentrations of NaCl (Bojórquez-Quintal et al., 2014). The successful cultivation and production of habanero pepper fruits is directly related to K+ availability (Monforte-Gonzalez et al., 2010) and salt stress induces the leakage of K+. Taking into account that maintenance of K+ absorption and decrease in Na+ accumulation represents an important strategy in developing tolerance to saline stress (Shabala and Cuin, 2008) and that the habanero pepper (C. chinense) actually is grown intensively in the southern region of Mexico where salinity problems could arise in the future (Delgado et al., 2010), the overexpression of Na+-insensitive K+ transporters such as CcHAK1 provides an attractive alternative for the improvement of glycophyte species production such as the peppers and to enhance salt tolerance of plants.
Accession Number
The nucleotide sequence reported in this paper has been submitted to GenBank with accession number KT202302.
Author Contributions
MM: Group leader and head of the research project; IE proposed experiments and writing manuscript; EB, NR, LS, BB, MFM, Isolation, sequence analysis of CcHAK1 cDNA, and phylogenetic tree. Functional complementation of CcHAK1 in the yeast Saccharomyces cerevisiae. Analysis of transcript levels of CcHAK1 by RT-PCR. Cation uptake experiments in yeast.
Funding
This work was supported by Consejo Nacional de Ciencia y Tecnología (CONACYT) project # 166621-Z.
Conflict of Interest Statement
The authors declare that the research was conducted in the absence of any commercial or financial relationships that could be construed as a potential conflict of interest.
Acknowledgments
We thank Alonso Rodriguez-Navarro and Centro de Biotecnología and Genómica de Plantas-Universidad Politécnica de Madrid (CBGP-UPM) for the use of equipment and laboratory facilities. We thank Dr. Francisco Rubio (Departamento de Nutrición Vegetal, Centro de Edafología y Biología Aplicada del SEGURA-CSIC, Murcia, Spain) for the donation the yeast strain with the HAK1-transporter of C. annuum (CaHAK1). For their excellent technical assistance we thank Blanca Garciadeblás (Centro de Biotecnología and Genómica de Plantas-Universidad Politécnica de Madrid, CBGP-UPM). We also thank CONACYT for the scholarships awarded to NR (#205076) and EB (#224261) for her doctoral studies and to the grand to CONACYT (166621).
Supplementary Material
The Supplementary Material for this article can be found online at: http://journal.frontiersin.org/article/10.3389/fpls.2016.01980/full#supplementary-material
Abbreviations
AKT1, Arabidopsis K+ transporter 1; AP-U medium, arginine phosphate lacking uracil medium; BY2 cells, bright yellow 2 cells; CHX, cation/proton (H+) antiporters; HAK, high-affinity K+ transporter; HKT2, high-affinity K+ transporters 2; KUP/HAK/KT, K+ uptake permeases/high-affinity K+ transporters/K+ transporter; ORF, open reading frame; YPD medium, yeast extract-peptone-dextrose.
References
Adams, E., Abdollahi, P., and Shin, R. (2013). Cesium inhibits plant growth through Jasmonate Signaling in Arabidopsis thaliana. Int. J. Mol. Sci. 14, 4545–4559. doi: 10.3390/ijms14034545
Ahmad, I., and Maathuis, F. J. M. (2014). Cellular and tissue distribution of potassium: physiological relevance mechanisms and regulation. J. Plant Physiol. 171, 708–714. doi: 10.1016/j.jplph.2013.10.016
Ahn, S. J., Shin, R., and Schachtman, D. P. (2004). Expression of KT/KUP genes in Arabidopsis and the role of root hairs in K+ uptake. Plant Physiol. 134, 1135–1145. doi: 10.1104/pp.103.034660
Alemán, F., Caballero, F., Ródenas, R., Rivero, R. M., Martínez, V., and Rubio, F. (2014). The F130S point mutation in the Arabidopsis high-affinity K+ transporter AtHAK5 increases K+ over Na+ and Cs+ selectivity and confers Na+ and Cs+ tolerance to yeast under heterologous expression. Front. Plant Sci. 5:430. doi: 10.3389/fpls.2014.00430
Alemán, F., Nieves-Cordones, M., Martínez, V., and Rubio, F. (2009). Differential regulation of the HAK5 genes encoding the high-affinity K+ transporters of Thellungiella halophila and Arabidopsis thaliana. Environ. Exp. Bot. 65, 263–269. doi: 10.1016/j.envexpbot.2008.09.011
Alemán, F., Nieves-Cordones, M., Martínez, V., and Rubio, F. (2011). Root K+ acquisition in plants: the Arabidopsis thaliana model. Plant Cell Physiol. 52, 1603–1612. doi: 10.1093/pcp/pcr096
Anschütz, U., Becker, D., and Shabala, S. (2014). Going beyond nutrition: regulation of potassium homoeostasis as a common denominator of plant adaptive responses to environment. J. Plant Physiol. 171, 670–687. doi: 10.1016/j.jplph.2014.01.009
Bañuelos, M. A., Garciadeblás, B., Cubero, B., and Rodríguez-Navarro, A. (2002). Inventory and functional characterization of the HAK potassium transporters of rice. Plant Physiol. 130, 784–795. doi: 10.1104/pp.007781
Benito, B., Haro, R., Amtmann, A., Cuin, T. A., and Dreyer, I. (2014). The twins K+ and Na+ in plants. J. Plant Physiol. 171, 723–731. doi: 10.1016/j.jplph.2013.10.014
Bojórquez-Quintal, J. E., Echevarría-Machado, I., Medina-Lara, F., and Martínez-Estévez, M. (2012). Plants challenges in a salinized world: the case of Capsicum. Afr. J. Biotechnol. 11, 13614–13626. doi: 10.5897/ajb12.2145
Bojórquez-Quintal, J. E., Velarde-Buendía, A., Ku-González, A., Carrillo-Pech, M., Ortega-Camacho, D., Echevarría-Machado, I., et al. (2014). Mechanisms of salt tolerance in habanero pepper plants (Capsicum chinense Jacq.): proline accumulation, ions dynamics and sodium root-shoot partition and compartmentation. Front. Plant Sci. 5:605. doi: 10.3389/fpls.2014.00605
Borges-Gómez, L., Escamilla-Bencomo, A., Soria-Fregoso, M., and Casanova-Villareal, V. (2005). Potasio en suelos de Yucatán. TERRA Latin. 23, 437–445.
Boscari, A., Clement, M., Volkov, V., Golldack, D., Hybiak, J., and Miller, A. J. (2009). Potassium channels in barley: cloning, functional characterization and expression analyses in relation to leaf growth and development. Plant Cell Environ. 32, 1761–1777. doi: 10.1111/j.1365-3040.2009.02033.x
Bose, Y., Shabala, L., Pottosin, I., Zeng, F., Velarde-Buendia, A. M., Massart, A., et al. (2014). Kinetics of xylem loading, membrane potential maintenance, and sensitivity of K+ -permeable channels to reactive oxygen species: physiological traits that differentiate salinity tolerance between pea and barley. Plant Cell Environ. 37, 589–600. doi: 10.1111/pce.12180
Brunelli, J. P., and Pall, M. L. (1993). A series of yeast shuttle vectors for expression of cDNAs and other DNA sequences. Yeast 9, 1299–1308. doi: 10.1002/yea.320091203
Celis-Arámburo, T. J., Carrillo-Pech, M., Castro-Concha, L. A., Miranda-Ham, M. L., Martínez-Estévez, M., and Echevarría-Machado, I. (2011). Exogenous nitrate induces root branching and inhibits primary root growth in Capsicum chinense Jacq. Plant Physiol. Biochem. 49, 1456–1464. doi: 10.1016/j.plaphy.2011.09.003
Chérel, I., Lefoulon, C., Boeglin, M., and Sentenac, H. (2014). Molecular mechanisms involved in plant adaptation to low K+ availability. J. Exp. Bot. 65, 833–848. doi: 10.1093/jxb/ert402
Davies, C., Shin, R., Liu, W., Thomas, M. R., and Schachtman, D. P. (2006). Transporters expressed during grape berry (Vitis vinifera L.) development are associated with an increase in berry size and berry potassium accumulation. J. Exp. Bot. 57, 3209–3216. doi: 10.1093/jxb/erl091
Delgado, C., Pacheco, J., Cabrera, A., Batllori, E., Orellana, R., and Bautista, F. (2010). Quality of groundwater for irrigation in tropical karst enviroment: the case of Yucatan, Mexico. Agr. Water Manage. 97, 1423–1433. doi: 10.1016/j.agwat.2010.04.006
Demidchik, V. (2014). Mechanisms and physiological roles of K+ efflux from roots cells. J. Plant Physiol. 171, 696–707. doi: 10.1016/j.jplph.2014.01.015
Demidchik, V., Straltsova, D., Medvedev, S. S., Pozhvanov, G. A., Sokolik, A., and Yurin, V. (2014). Stress-induced electrolyte leakage: the role of K+-permeable channels and involvement in programmed cell death and metabolic adjustment. J. Exp. Bot. 65, 1259–1270. doi: 10.1093/jxb/eru004
Desbrosses, G., Kopka, C., Ott, T., and Udvardi, M. K. (2004). Lotus japonicus LjKUP is induced late during nodule development and encodes a potassium transporter of the plasma membrane. Mol. Plant Microbe Interact. 17, 789–797. doi: 10.1094/MPMI.2004.17.7.789
Epstein, E., Rains, D. W., and Elzam, O. E. (1963). Resolution of dual mechanisms of potassium absorption by barley roots. Proc. Natl. Acad. Sci. U.S.A. 49, 684–692. doi: 10.1073/pnas.49.5.684
Fraile-Escanciano, A., Kamisugi, Y., Cuming, A. C., Rodríguez-Navarro, A., and Benito, B. (2010). The SOS1 transporter of physcosmitrella patents mediates sodium efflux in Planta. New Phytol. 188, 750–761. doi: 10.1111/j.1469-8137.2010.03405.x
Fulgenzi, F. R., Peralta, M. L., Mangano, S., Danna, C. H., Vallejo, A. J., Puigdomenech, P., et al. (2008). The ionic environment controls the contribution of the barley HvHAK1 transporter to potassium acquisition. Plant Physiol. 147, 252–262. doi: 10.1104/pp.107.114546
Garcia, K., and Zimmermann, S. D. (2014). The role of mycorrhizal associations in plant potassium nutrition. Front. Plant Sci. 5:337. doi: 10.3389/fpls.2014.00337
Garciadeblás, B., Benito, B., and Rodríguez-Navarro, A. (2002). Molecular cloning and functional expression in bacteria of the potassium transporters CnHAK1 and CnHAK2 of the seagrass Cyamodocea nodosa. Plant Mol. Biol. 50, 623–633. doi: 10.1023/A:1019951023362
Gómez-Porras, J. L., Ria-o-Pachón, D. M., Benito, B., Haro, R., Sklodowski, K., Rodríguez-Navarro, A., et al. (2012). Phylogenetic analysis of K+ transporters in bryophytes, lycophytes, and flowering plants indicates a specialization of vascular plants. Front. Plant Sci. 3:167. doi: 10.3389/fpls.2012.00167
Grabov, A. (2007). Plant KT/KUP/HAK potassium transporters: single family-multiple functions. Ann. Bot. 99, 1035–1041. doi: 10.1093/aob/mcm066
Greiner, T., Ramos, J., Alvarez, M. C., Gurnon, J. R., Kang, M., Van Etten, J. L., et al. (2011). Functional HAK/KUP/KT-like potassium transporter encoded by chlorella viruses. Plant J. 68, 977–986. doi: 10.1111/j.1365-313X.2011.04748.x
Gupta, M., Qiu, X., Wang, L., Xie, W., Zhang, C., Xiong, L., et al. (2008). KT/HAK/KUP potassium transporters gene family and their whole-life cycle expression profile in rice (Oryza sativa). Mol. Genet. Genomics 280, 437–452. doi: 10.1007/s00438-008-0377-7
Hamilton, R., Watanabe, C. K., and Boer, H. A. (1987). Compilation and comparison of the sequence context around the AUG start codons in Saccharomyces cerevisiae mRNAs. Nucleic Acids Res. 15, 3581–3593. doi: 10.1093/nar/15.8.3581
Hampton, C. R., Bowen, H. C., Broadley, M. R., Hammond, J. P., Mead, A., Payne, K. A., et al. (2004). Cesium toxicity in Arabidopsis. Plant Physiol. 136, 3824–3837. doi: 10.1104/pp.104.046672
Haro, R., Fraile-Escanciano, A., González-Melendi, P., and Rodríguez-Navarro, A. (2013). The potassium transporters HAK2 and HAK3 localize to endomembranes in Physcomitrella patens. HAK2 is required in some stress conditions. Plant Cell Physiol. 54, 1441–1454. doi: 10.1093/pcp/pct097
Haro, R., and Rodríguez-Navarro, A. (2003). Functional analysis of the M2D helix of the TRK1 potassium transporter of Saccharomyces cervisiae. Biochim. Biophys. Acta 1613, 1–6. doi: 10.1016/S0005-2736(03)00132-9
Haro, R., Sainz, L., Rubio, F., and Rodríguez-Navarro, A. (1999). Cloning of two genes encoding potassium transporters in Neurospora crassa and expression of the corresponding cDNAs in Saccharomyces cerevisiae. Mol. Microbiol. 31, 511–520. doi: 10.1046/j.1365-2958.1999.01192.x
He, C., Cui, K., Duan, A., Zeng, Y., and Zhang, J. (2012). Genome-wide and molecular evolution analysis of the Poplar KT/HAK/KUP potassium transporter gene family. Ecol. Evol. 2, 1996–2004. doi: 10.1002/ece3.299
Horie, T., Sugawara, M., Okada, T., Taira, K., Kaothien-Nakayama, P., Katsuhara, M., et al. (2011). Rice sodium-insensitive potassium transporter, OsHAK5, confers increased salt tolerance in tobacco BY2 cells. J. Biosci. Bioeng. 111, 346–356. doi: 10.1016/j.jbiosc.2010.10.014
Hosoo, Y., Kimura, Y., Nanatani, K., and Uozumi, N. (2014). Molecular cloning and expression analysis of a gene encoding KUP/HAK/KT-type potassium uptake transporter from Cryptomeria japonica. Trees 28, 1527–1537. doi: 10.1007/s00468-014-1059-1
Hyun, T. K., Rim, Y., Kim, E., and Kim, J. S. (2014). Genome-wide and molecular evolution analyses of the KT/HAK/KUP family in tomato (Solanum lycopersicum L.). Genes Genom. 36, 365–374. doi: 10.1007/s13258-014-0174-0
Maathuis, F. J. M. (2009). Physiological functions of mineral macronutrients. Curr. Opin. Plant Biol. 12, 250–258. doi: 10.1016/j.pbi.2009.04.003
Mangano, S., Silberstein, S., and Santa-Maria, G. E. (2008). Point mutations in the barley HvHAK1 potassium transporter lead to improved K+-nutrition and enhanced resistance to salt stress. FEBS Lett. 582, 3922–3928. doi: 10.1016/j.febslet.2008.10.036
Martínez-Cordero, M. A., Martínez, V., and Rubio, F. (2004). Cloning and functional characterization of the high-affinity K+ transporter HAK1 of pepper. Plant Mol. Biol. 56, 413–421. doi: 10.1007/s11103-004-3845-4
Martínez-Cordero, M. A., Martínez, V., and Rubio, F. (2005). High-affinity K+ uptake in pepper plants. J. Exp. Bot. 56, 1553–1562. doi: 10.1093/jxb/eri150
Maser, P., Thomine, S., Schroeder, J. I., Ward, J. M., Hirschi, K., Sze, H., et al. (2001). Phylogenetic relationships within cation transporter families of Arabidopsis. Plant Physiol. 126, 1646–1667. doi: 10.1104/pp.126.4.1646
Medina-Lara, F., Echevarría-Machado, I., Pacheco-Arjona, R., Ruiz-Lau, N., Guzmán-Antonio, A., and Martinez-Estevez, M. (2008). Influence of nitrogen and potassium fertilization on fruiting and capsaicin content in habanero pepper (Capsicum chinense Jacq.). Hortscience 43, 1549–1554.
Monforte-Gonzalez, M., Guzmán-Antonio, A., Uuh-Chim, F., and Vázquez-Flota, F. (2010). Capsaicin accumulation is related to nitrate content in placentas of habanero peppers (Capsicum chinense Jacq.). J. Sci. Food Agric. 90, 764–768. doi: 10.1002/jsfa.3880
Nieves-Cordones, M., Alemán, F., Martínez, V., and Rubio, F. (2010). The Arabidopsis thaliana HAK5 K+ transporter is required for plant growth and K+ acquisition from low K+ solutions under saline conditions. Mol. Plant 3, 326–333. doi: 10.1093/mp/ssp102
Nieves-Cordones, M., Alemán, F., Martínez, V., and Rubio, F. (2014). K+ uptake in plant roots. The systems involved their regulation and parallels in other organisms. J. Plant Physiol. 171, 688–695. doi: 10.1016/j.jplph.2013.09.021
Nieves-Cordones, M., Martínez-Cordero, M., Martínez, V., and Rubio, F. (2007). An NH4+-sensitive component dominates high-affinity K+ uptake in tomato plants. Plant Sci. 172, 273–280. doi: 10.1016/j.plantsci.2006.09.003
Niu, G., and Rodríguez, D. S. (2010). Rapid screening for relative salt tolerance among chili pepper genotypes. Hortscience 45, 1192–1195.
Niu, G., Rodríguez, D. S., Call, E., Bosland, P. W., Ulery, A., and Acosta, E. (2010). Responses of eight chili peppers to saline water irrigation. Sci. Hortic. 126, 215–222. doi: 10.1016/j.scienta.2010.07.016
Pacheco-Arjona, J. R., Ruíz-Lau, N., Medina-Lara, F., Minero-García, Y., Echevarría-Machado, I., De los Santos-Briones, C., et al. (2011). Effects of ammonium nitrate, cesium chloride and tetraethylammonium on high-affinity potassium uptake in habanero pepper plantlets (Capsicum chinense Jacq.). Afr. J. Biotechnol. 10, 13418–13429. doi: 10.5897/AJB10.2097
Pyo, Y. J., Gierth, M., Schroeder, J. I., and Cho, M. H. (2010). High-affinity K+ transport in Arabidopsis: AtHAK5 and AKT1 are vital for seedling establishment and postgermination growth under low-potassium conditions. Plant Physiol. 153, 863–875. doi: 10.1104/pp.110.154369
Qi, Z., Hampton, C. R., Shin, R., Barkla, B. J., White, P. J., and Schachtman, D. P. (2008). The high affinity K+ transporter AtHAK5 plays a physiological role in planta at very low K+ concentrations and provides a cesium uptake pathway in Arabidopsis. J. Exp. Bot. 59, 595–607. doi: 10.1093/jxb/erm330
Rodriguez-Navarro, A. (2000). Potassium transport in fungi and plants. Biochim. Biophys. Acta 1469, 1–30. doi: 10.1016/S0304-4157(99)00013-1
Rodríguez-Navarro, A., and Ramos, J. (1984). Dual system for potassium transport in Saccharomyces cerevisiae. J. Bacteriol. 159, 940–945.
Rodríguez-Navarro, A., and Rubio, F. (2006). High-affinity potassium and sodium transport systems in plants. J. Exp. Bot. 57, 1149–1160. doi: 10.1093/jxb/erj068
Römheld, V., and Kirkby, E. A. (2010). Research on potassium in agriculture: needs and prospects. Plant Soil 335, 155–180. doi: 10.1007/s11104-010-0520-1
Ruan, Y. L., Llewellyn, D. J., and Furbank, R. T. (2001). The control of single-celled cotton fiber elongation by developmentally reversible gating of plasmodesmata and coordinated expression of sucrose and K+ transporters and expansin. Plant Cell 13, 47–60. doi: 10.1105/tpc.13.1.47
Rubio, F., Alemán, F., Nieves-Cordones, M., and Vicente, M. (2010). Studies on Arabidopsis athak5, atakt1 double mutants disclose the range of concentrations at which AtHAK5, AtAKT1 and unknown systems mediate K+ uptake. Physiol. Plant. 139, 220–228. doi: 10.1111/j.1399-3054.2010.01354.x
Rubio, F., Nieves-Cordones, M., Alemán, F., and Martínez, V. (2008). Relative contribution of AtHAK5 and AtAKT1 to K+ uptake in the high-affinity range of concentrations. Physiol. Plant. 134, 598–608. doi: 10.1111/j.1399-3054.2008.01168.x
Rubio, F., Santa-María, G. E., and Rodríguez-Navarro, A. (2000). Cloning of Arabidopsis and barley cDNAs encoding HAK potassium transporters in root and shoot cells. Physiol. Plant. 109, 34–43. doi: 10.1034/j.1399-3054.2000.100106.x
Santa-María, G. E., Danna, C. H., and Czibener, C. (2000). High-affinity potassium transport in barley roots. Ammonium-sensitive and –insensitive pathways. Plant Physiol. 123, 297–306. doi: 10.1104/pp.123.1.297
Santa-María, G. E., Rubio, F., Dubcovsky, J., and Rodríguez-Navarro, A. (1997). The HAK1 gene of barley is a member of a large gene family and encodes a high-affinity potassium transporter. Plant Cell 9, 2281–2289. doi: 10.1105/tpc.9.12.2281
Sato, Y., Nanatani, K., Hamamoto, S., Shimizu, M., Takahashi, M., Tabucho-Kobayashi, M., et al. (2014). Defining membrane spanning domains and crucial membrane-localized acidic amino acid residues for K+ transport of a KUP/HAK/KT-type Escherichia coli potassium transporter. J. Biochem. 155, 315–323. doi: 10.1093/jb/mvu007
Shabala, S., and Cuin, T. A. (2008). Potassium transport and plant salt tolerance. Physiol. Plant. 133, 651–669. doi: 10.1111/j.1399-3054.2007.01008.x
Shabala, S., and Pottosin, I. (2014). Regulation of potassium transport in plants under hostile conditions: implications for abiotic and biotic stress tolerance. Physiol. Plant. 151, 257–279. doi: 10.1111/ppl.12165
Shen, Y., Shen, L., She, Z., Jing, W., Ge, H., Zhao, J., et al. (2015). The potassium transporter OsHAK21 functions in the maintenance of ion homeostasis and tolerance to salt stress in rice. Plant Cell Environ. 38, 2766–2779. doi: 10.1111/pce.12586
Sherman, F. (1991). Getting started with yeast. Methods Enzymol. 194, 3–21. doi: 10.1016/0076-6879(91)94004-V
Song, Z., Guo, S., Zhang, C., Zhang, B., Ma, R., Korir, N. K., et al. (2015). KT/HAK/KUP potassium transporter genes differentially expressed during fruit development, ripening, and postharvest shelf-life of Xiahui6 peaches. Acta Physiol. Plant. 37, 131, doi: 10.1007/s11738-015-1880-1
Song, Z. Z., Ma, R. J., and Yu, M. L. (2015). Genome-wide analysis and identification of KT/HAK/KUP potassium transporter gene family in peach (Prunus persica). Genet. Mol. Res. 14, 774–787. doi: 10.4238/2015.January.30.21
Spalding, E. P., Hirsch, R. E., Lewis, D. R., Qi, Z., Sussman, M. R., and Lewis, B. D. (1999). Potassium uptake supporting plant growth in the absence of AKT1 channel activity. Inhibition by ammonium and stimulation by sodium. J. Gen. Physiol. 113, 909–918. doi: 10.1085/jgp.113.6.909
Su, H., Golldack, D., Zhao, C., and Bohnert, H. J. (2002). The expression of HAK-type K+ transporters is regulated in response to salinity stress in common ice plant. Plant Physiol. 129, 1482–1493. doi: 10.1104/pp.001149
Su, Q., Feng, S., An, L., and Zhang, G. (2007). Cloning and functional expression in Saccharomyces cereviae of a K+ transporter, AlHAK, from the graminaceous halophyte, Aeluropus littoralis. Biotechnol. Lett. 29, 1959–1963. doi: 10.1007/s10529-007-9484-5
Takahashi, R., Nishio, T., Ichizen, N., and Takano, T. (2007a). Cloning and functional analysis of the K+ transporter, PhaHAK2, from salt-sensitive and salt-tolerant reed plants. Biotechnol. Lett. 29, 501–506. doi: 10.1007/s10529-006-9246-9
Takahashi, R., Nishio, T., Ichizen, N., and Takano, T. (2007b). High-affinity K+ transporter PhaHAK5 is expressed only in salt-sensitive reed plants and shows Na+ permeability under NaCl stress. Plant Cell Rep. 26, 1673–1679. doi: 10.1007/s00299-007-0364-1
ten-Hoopen, F., Cuin, T. A., Pedas, P., Hegelund, J. N., Shabala, S., Schjoerring, J. K., et al. (2010). Competition between uptake of ammonium and potassium in barley and Arabidopsis roots: molecular mechanisms and physiological consequences. J. Exp. Bot. 61, 2303–2315. doi: 10.1093/jxb/erq057
Vallejo, A. J., Peralta, M. L., and Santa-María, G. E. (2005). Expression of potassium-transporter coding genes, and kinetics of rubidium uptake, along a longitudinal root axis. Plant Cell Environ. 28, 850–862. doi: 10.1111/j.1365-3040.2005.01334.x
Véry, A. A., Nieves-Cordones, M., Daly, M., Khan, I., Fizames, C., and Sentenac, H. (2014). Molecular biology of K+ transport across the plant cell membrane: what do we learn from comparison between plant species? J. Plant Physiol. 171, 748–769. doi: 10.1016/j.jplph.2014.01.011
Wang, M., Zheng, Q., Shen, Q., and Guo, S. (2013). The critical role of potassium in plant stress response. Int. J. Mol. Sci. 14, 7370–7390. doi: 10.3390/ijms14047370
Yang, T., Zhang, S., Hu, Y., Wu, F., Hu, Q., Chen, G., et al. (2014). The role of a potassium transporter OsHAK5 in potassium acquisition and transport from roots to shoots in rice at low potassium supply levels. Plant Physiol. 166, 945–959. doi: 10.1104/pp.114.246520
Yang, Z. F., Gao, Q. S., Sun, C. S., Li, W. J., Gu, S. L., and Xu, C. W. (2009). Molecular evolution and functional divergence of HAK potassium transporter gene family in rice (Oryza sativa L.). J. Genet. Genomics 36, 161–172. doi: 10.1016/S1673-8527(08)60103-4
Yenush, L. (2016). “Potassium and sodium transport in yeast,” in Yeast Membrane Transport, eds J. Ramos, H. Sychrová, and M. Kschischo (Springer International Publishing), 187–228.
Zhang, Z., Zhang, J., Chen, Y., Li, R., Wang, H., and Wei, J. (2012). Genome-wide analysis and identification of HAK potassium transporter gene family in maize (Zea mays L.). Mol. Biol. Rep. 39, 8465–8473. doi: 10.1007/s11033-012-1700-2
Keywords: Capsicum chinense, HAK-type transporter, K+-starvation, potassium, roots, sodium
Citation: Ruiz-Lau N, Bojórquez-Quintal E, Benito B, Echevarría-Machado I, Sánchez-Cach LA, Medina-Lara MdF and Martínez-Estévez M (2016) Molecular Cloning and Functional Analysis of a Na+-Insensitive K+ Transporter of Capsicum chinense Jacq. Front. Plant Sci. 7:1980. doi: 10.3389/fpls.2016.01980
Received: 14 September 2016; Accepted: 13 December 2016;
Published: 27 December 2016.
Edited by:
Karabi Datta, University of Calcutta, IndiaReviewed by:
Ryoung Shin, Riken Center for Sustainable Resource Science, JapanAlexander Schulz, University of Copenhagen, Denmark
Copyright © 2016 Ruiz-Lau, Bojórquez-Quintal, Benito, Echevarría-Machado, Sánchez-Cach, Medina-Lara and Martínez-Estévez. This is an open-access article distributed under the terms of the Creative Commons Attribution License (CC BY). The use, distribution or reproduction in other forums is permitted, provided the original author(s) or licensor are credited and that the original publication in this journal is cited, in accordance with accepted academic practice. No use, distribution or reproduction is permitted which does not comply with these terms.
*Correspondence: Manuel Martínez-Estévez, luismanh@cicy.mx
†These authors have contributed equally to this work.