- 1Central Infrastructure Group Genomics and Transcript Profiling, Max-Planck-Institute of Molecular Plant Physiology, Potsdam, Germany
- 2Institute of Biology, Freie Universität Berlin, Berlin, Germany
- 3Department of Chemistry and Bioscience, Aalborg University, Aalborg East, Denmark
Chromatin regulation ensures stable repression of stress-inducible genes under non-stress conditions and transcriptional activation and memory of stress-related genes after stress exposure. However, there is only limited knowledge on how chromatin genes are regulated at the transcriptional and post-transcriptional level upon stress exposure and relief from stress. We reveal that the repressive modification histone H3 lysine 27 trimethylation (H3K27me3) targets genes which are quickly activated upon cold exposure, however, H3K27me3 is not necessarily lost during a longer time in the cold. In addition, we have set-up a quantitative reverse transcription polymerase chain reaction-based platform for high-throughput transcriptional profiling of a large set of chromatin genes. We find that the expression of many of these genes is regulated by cold. In addition, we reveal an induction of several DNA and histone demethylase genes and certain histone variants after plants have been shifted back to ambient temperature (deacclimation), suggesting a role in the memory of cold acclimation. We also re-analyze large scale transcriptomic datasets for transcriptional regulation and alternative splicing (AS) of chromatin genes, uncovering an unexpected level of regulation of these genes, particularly at the splicing level. This includes several vernalization regulating genes whose AS may result in cold-regulated protein diversity. Overall, we provide a profiling platform for the analysis of chromatin regulatory genes and integrative analyses of their regulation, suggesting a dynamic regulation of key chromatin genes in response to low temperature stress.
Introduction
Plants are exposed to a multitude of abiotic and biotic stresses during their lifetime and have evolved efficient mechanisms to cope with such events. Stress alleviation relies on numerous changes at the biochemical, physiological and molecular level, largely coordinated by a massive and fast reprogramming of the transcriptome. While it is known that the exposure to chilling temperatures results in the up- and downregulation of thousands of genes, as well as extensive post-transcriptional regulation of genes such as alternative splicing, all within minutes of cold exposure (Calixto et al., 2018), the precise mechanisms leading to this reprogramming have not been completely elucidated yet. Changes in the transcriptional activity of cold-stress-responsive genes upon exposure to low temperatures might be partly achieved through remodeling of the chromatin, rendering it more or less accessible for the transcriptional machinery, thereby affecting the expression levels of the genes. After cold stress ends and deacclimation begins, most stress-responsive genes return quickly back to their initial transcriptional levels (Byun et al., 2014; Pagter et al., 2017), suggesting that cold-induced changes to the chromatin might be reversed during deacclimation. Cold induced changes to the chromatin have in particular been studied in the context of vernalization. Vernalization is defined as the acquisition of the competence to flower after prolonged cold treatment, allowing vernalization-responsive plants to flower in spring, under favorable temperature conditions and the appropriate photoperiod. This process relies on epigenetic mechanisms, as cold induces a mitotically stable switch inhibiting the expression of the floral repressor FLOWERING LOCUS C (FLC). The repression of FLC is achieved through the action of regulators of the Polycomb-group (Pc-G), which deposit and maintain the repressive tri-methylation of lysine 27 in histone H3 (H3K27me3) upon cold exposure (Song et al., 2012). Changes in the chromatin state were also previously described for cold-inducible genes not involved in vernalization, suggesting the involvement of dynamic chromatin regulation in the induction and repression of cold stress-responsive genes (Kwon et al., 2009; Park et al., 2018). Hyper-acetylation of histone H3K9 in promoter regions of DREB1, a main regulator of cold response, was observed in rice and was decreased again when plants were returned to control temperatures, suggesting that deacetylation keeps the gene in an off-state in the absence of cold (Roy et al., 2014). Recently, epigenetic changes involved in cold response were reviewed by different authors (Baulcombe and Dean, 2014; Kim et al., 2015; Asensi-Fabado et al., 2017; Banerjee et al., 2017; Luo et al., 2017; Friedrich et al., 2019). Furthermore, epigenetic changes that establish environmental memory in plants have been described (He and Li, 2018). The existence of memory of a cold priming event, including transcriptional memory, resulting in improved freezing tolerance after a subsequent triggering cold treatment was recently shown (Zuther et al., 2019).
In general, chromatin can be distinguished into euchromatin, consisting of mostly active genes and closed inactive heterochromatin, with a preference for repetitive elements (Allis and Jenuwein, 2016). Chromatin is composed of basic repeating units called nucleosomes, consisting of 145 to 147 bp of DNA wrapped around a histone octamer (Luger et al., 1997). The octamer is formed by core histones H2A, H2B, H3, and H4 and the repeating nucleosomal structure is further linked and stabilized by the linker histone H1 (Luger et al., 1997). This results in the arrangement of higher-order helical structures (Widom, 1989). The nucleosome not only helps in the packaging of the DNA, but is also the primary determinant of DNA accessibility (Luger et al., 1997). Histones, particularly their tails, are extensively post-translationally modified and can undergo a variety of covalent modifications such as acetylation, methylation, phosphorylation, and ubiquitination (Zhang and Reinberg, 2001).
Histone acetylation is set by histone acetyltransferases (HAC), which transfer the acetyl group of acetyl Coenzyme A to the ϵ-amino group of lysine side chains (Bannister and Kouzarides, 2011). Histone acetylation is associated with a function as a transcriptional coactivator by neutralizing the positive charge of the lysine. The modification is reversible and the acetyl group can be removed by histone deacetylases to restore the positive charge of the lysine, thus stabilizing the local chromatin architecture. Histone methylation predominantly occurs on the amino acids lysine and arginine. Unlike acetylation, the charge of the histone is not affected (Bannister and Kouzarides, 2011). Lysine residues can be mono-, di-, or trimethylated, while arginine can contain one or two methyl groups on its guanidinyl group (Ng et al., 2009). Histone methylation can lead to an active or repressive chromatin state, depending on the modified residue and the number of added methyl groups. Trithorax group (Trx-G) factors are responsible for the deposition of activating methylations on lysine 4 and 36 of histone 3 (H3K4me3 and H3K36me3, respectively), leading to the transcriptional activation of their target genes (Del Prete et al., 2015). Their action is antagonized by the proteins of the Polycomb Repressive Complex 2 (PRC2), which mediates H3K27me3. This highly conserved methyltransferase complex contains three orthologues of Enhancer of zeste [E(z); CURLY LEAF (CLF), SWINGER (SWN), and MEDEA (MEA)], three orthologues of Suppressor of zeste12 [Su(z)12; EMBRYONIC FLOWER2 (EMF2), VERNALISATION2 (VRN2), and FERTILISATION INDEPENDENT SEED2 (FIS2)], five orthologues of Multicopy Suppressor of Ira (MSI1-5), and a single copy of Extra Sex Combs (ESC) [FERTILIZATION INDEPENDENT ENDOSPERM (FIE) in Arabidopsis thaliana (Kleinmanns and Schubert, 2014). Methylation can be reversed by two different classes of histone demethylases: while LSD1-type demethylases can remove one of two methyl groups, JUMONJI-type histone demethylases can counteract mono-, di- or trimethylation (Shi et al., 2004). The second highly conserved Polycomb-Repressive Complex, PRC1, is a histone ubiquitination complex and monoubiquitylates histone H2A (Kleinmanns and Schubert, 2014).
In addition to histone modifications, the state of chromatin can also be affected by DNA methylation, which was found to be linked to gene repression (Hotchkiss, 1948; Razin and Riggs, 1980). DNA methylation (5-methyl cytosine) can be a heritable epigenetic mark (Jin et al., 2011) and is set by DNA methyltransferases. In plants, cytosine can be methylated symmetrically [CG and CHG methylation (where H is any base except G) as well as asymmetrically (CHH)] and these modifications are predominantly found on transposons and other repetitive DNA elements (Zhang et al., 2006). Small RNAs generated by RNA interference (RNAi) target genomic DNA sequences for cytosine methylation (RNA-directed DNA methylation) (Law and Jacobsen, 2010). DNA methylation is reversible and the methyl groups can be removed by demethylases (Penterman et al., 2007). In general, promoter DNA methylation is associated with the repressive state of chromatin, as it alters the accessibility for transcription factors (Carey et al., 2011).
Dynamic regulation of chromatin is not only achieved by enzymes setting and removing chemical modifications at DNA or histones, but also by replacement of the canonical histones by histone variants, resulting in an immediate loss of histone modifications and a resetting of epigenetic changes (Spiker, 1982). Although nucleosomes are energetically stable, histones can be turned over. Histones H2A and H2B can be exchanged much faster than histones H3 and H4 (Weber and Henikoff, 2014). In A. thaliana, 13 H2A variants (labeled HTA1-13) exist, and are clustered in four groups: H2A, H2A.X, H2A.W, and H2A.Z (Kawashima et al., 2015). Histone H3 exists in 15 variants (labeled HTR1-15) distributed in categories including H3.1, H3.3, and CenH3 (Stroud et al., 2012). Different histone variants carry different functions and are located at different parts of the gene to convey regulation. The H3.3 variants are predominantly located towards the 3′-ends of genes and are generally associated with elevation of gene expression, whereas H2A.W binds to heterochromatin with its C-terminal motif KSPKKA and promotes heterochromatin condensation (Yelagandula et al., 2014; Kawashima et al., 2015). Additionally studies have identified H4 variants, however, protein variants have not been described in A. thaliana (Kawashima et al., 2015). Lastly, three copies of linker histone H1 exist in A. thaliana, H1.1, H1.2, and H1.3 (Kotliński et al., 2016). H1.3 is a stress-inducible histone variant and might be responsible for regulating dynamic DNA methylation (Rutowicz et al., 2015).
While there is ample evidence for a role of chromatin remodeling in the regulation of gene expression in response to cold, relatively little is known about the involvement of specific chromatin regulators. The transcriptional and post-transcriptional regulation of the expression of most of these chromatin modifier genes both during and after cold exposure remains unexplored as well. In the case of vernalization, VERNALIZATION INSENSITIVE 3 (VIN3) is the only VRN gene known to be induced by cold, however, protein level analyses of Pc-G proteins involved in vernalization suggest post-transcriptional regulation of several genes including VRN2, CLF, FIE, and SWN (Wood et al., 2006).
Here, we set out to analyze the transcriptional and post-transcriptional regulation of chromatin regulatory genes in response to cold stress and following deacclimation using both publically available datasets and generation of a quantitative reverse transcription polymerase chain reaction (RT-qPCR) platform. We identify a potential role for Pc-G proteins in repressing stress-inducible genes under non-stress conditions and substantial transcriptional and post-transcriptional regulation of chromatin regulatory genes.
Interestingly, genes involved in vernalization are largely not transcriptionally regulated under short-term (3 days) cold conditions. However, they may be alternatively spliced, resulting in potentially altered protein sequences. Based on data generated with the RT-qPCR platform, we have identified additional cold-inducible chromatin regulatory genes and genes specifically regulated during deacclimation, including DNA demethylases and histone variants.
Methods
Plant Material
A. thaliana accession Col-0 seeds were sown in a single pot and grown on soil in a climate chamber with 20°C day-time temperature and 6°C night-time temperature in a 14-h light cycle with a light intensity of 180 µE m−2 s−1 and a humidity of 60% at day and 70% at night. After 1 week the plants were moved to a short-day climate chamber with the following conditions: 20°C/16°C day/night, 8 h day length, 180 µE m−2 s−1, humidity of 60%/75% day/night. The plants were kept under these short-day conditions for a week before transferring them to new pots (10 plants per 10-cm diameter pot). Afterwards, the plants were kept for another 7 days under short-day conditions before transfer to long-day conditions for another week. The conditions for long-day were 20°C day and 16°C night temperature with a day length of 16 h at a light intensity of 200 µE m−2 s−1. These 4-week-old plants were used in cold acclimation and deacclimation experiments. For cold acclimation, plants were moved for 3 days to a growth chamber with a constant temperature of 4°C and a day length of 16 h with a light intensity of 90 µE m−2 s−1 and a humidity of 70% to 80% (Zuther et al., 2019). For deacclimation, plants were moved back to previous growth conditions for up to 24 h (Pagter et al., 2017). Plant material of 10 individual replicate plants was harvested from non-acclimated (NA) plants (at 8 am), after 3 days of cold acclimation (at 8 am) and after 2, 4, 6, 12, and 24 h of deacclimation (Deacc). The material was immediately frozen in liquid nitrogen and stored at −80°C before being ground into a fine powder in a ball mill (Retsch, Haan, Germany).
Selection of Genes of Interest for the RT-qPCR Platform
We focused our selection on chromatin genes associated with epigenetic changes and selected 135 genes for analyses (see Table 1 for abbreviations and Supplemental Table 2 for primer sequences). These include Pc-G genes and Pc-G associated genes (and their paralogs), Trx-G genes, a selection of histone demethylase genes (putative H3K9 and H3K27 demethylases (JUMONJI-type) and LSD1-like histone demethylases), DNA methyltransferase and demethylase, and canonical histone and histone variant genes.

Table 1 Quantitative reverse transcription polymerase chain reaction (RT-qPCR) platform: Nomenclature of 135 genes encoding epigenetic regulators.
Chromatin Immunoprecipitation and (RT-)qPCR Analysis
Plants were grown for 21 days on ½ MS medium under SD conditions (8 h light, 16 h darkness) and transferred to 4°C for 3 h or 3 days. Seedlings (0.5–1 g) were crosslinked in phosphate-buffered saline (PBS) containing 1% paraformaldehyde for 10 min under vacuum. The crosslink reaction was stopped by the addition of 0.125 M glycine. Samples were rinsed with ice-cold PBS solution and frozen in liquid nitrogen. Chromatin extraction was performed as previously described (Kleinmanns et al., 2017). The extracted chromatin was incubated rotating at 4°C for 16 h with 1 µg of antibodies (anti-H3K27me3, C15410195 Diagenode; anti-H3pan, C15200011 Diagenode; anti-IgG, C15410206 Diagenode). Twenty microliters of Protein A couple beads (Thermo Fisher Scientific) were added and the samples were incubated rotating at 4°C for 4 h. Beads were washed and DNA purified as previously described (Kleinmanns et al., 2017). The qPCR analysis was performed using the Takyon ROX SYBR MasterMix blue dTTP kit and the QuantStudio5 (Applied Biosystems). Primers were designed to amplify loci carrying H3K27me3 according to the Jacobsen Epigenome Browser (Zhang et al., 2007) and their sequences can be found in Supplemental Table 1. Amplification values were normalized to input and to the FUSCA3 (AT3G26790) locus or the ACTIN7 (AT5G09810) locus, depending on the analyzed mark. Statistical significance was tested using one-way ANOVA followed by a Dunnett's multiple comparison test using GraphPad Prism7.
For gene expression analysis, RNA was isolated from 100 mg of seedlings grown as described above, using the innuPREP Plant RNA Kit (Analytik Jena). RNA was quantified using a Nanodrop-1000 spectrophotometer (Thermo Scientific). Genomic DNA was removed using the DNase I kit (Thermo Scientific) and cDNA was synthetized using the RevertAid Reverse Transcriptase (Thermo Scientific). The quantitative PCR was performed as described above. The Ct values were normalized by subtracting the mean of the three housekeeping genes ACTIN2 (AT3G18780), PDF (AT1G13320), and TIP41 (AT4G34270) AT4G34270 and taken as the negative exponent to the base 2. Primer sequences can be found in Supplemental Table 1. Statistical significance was tested using one-way ANOVA followed by a Dunnett's multiple comparison test using GraphPad Prism7.
RT-qPCR Analysis for the Epigenetic Primer Platform
RNA was isolated from a pool of five samples consisting of 10 different plants using Trizol reagent (BioSolve BV). RNA was quantified using a NanoDrop-1000 spectrophotometer (Thermo Scientific) and DNA was removed from the samples using a RapidOut DNA Removal Kit (Thermo Scientific). The absence of genomic DNA was tested by qPCR with intron-specific primers (Intron MAF5 AT5G65080) (Zuther et al., 2012). cDNA was synthesised by SuperScript IV Reverse Transcriptase (Invitrogen) and oligo dT18 primers. The quality of the cDNA was tested using primers amplifying the 3′ and 5′ region of GAPDH (AT1G13440) (Zuther et al., 2012). cDNA of three independent biological replicates for each time point was used for expression analysis (Pagter et al., 2017).
RT-qPCR was performed for 135 genes of interest (Supplemental Table 2) as previously described (Le et al., 2015). Expression of four housekeeping genes, Actin2 (AT3G18780), EXPRS (AT2g32170), GAPDH (AT1G13440), and PDF (AT1G13320) was measured for each sample on each plate (Supplemental Table 3; compare Zuther et al., 2012). The Ct values were normalized by subtracting the mean of the four housekeeping genes from the Ct value of each gene of interest (ΔCt). Transcript abundance was expressed as 2−ΔCt. The log2 fold change of the normalized Ct values was calculated either relative to the values obtained from NA or cold-acclimated (ACC) plants.
Heat maps were constructed in RStudio (R Core Team, 2013; RStudio Team, 2016) using the pheatmap package version 1.0.12.
Primer Design
Primers were either designed in Primer3 or taken from the literature as indicated in Supplemental Table 2. The specifications of the primers were: primer length 20–24 bases, amplicon size 60–150 bp, primer melting temperature 64°C ± 3°C, amplicon melting temperature 75°C–95°C, G/C content 45%–55%, maximum repetition of a nucleotide of 3, and a G/C clamp of 1. Primers with a binding site near the 3′ end of the respective gene were preferred. For highly homologous genes maximum primer length was increased to 30 bp.
Bioinformatic Analyses of the Overlap of Cold-Regulated Genes and H3K27me3 Targets Using Public Data
The dataset of differentially expressed cold-regulated genes was extracted from previously published data (Calixto et al., 2018). Experimental conditions used in this publication were as follows. A. thaliana Col-0 plants were stratified for 4 days at 4°C in the dark and then grown in hydroponic culture for 5 weeks. Growth conditions were 12 h light (150 μE m−2 s−1)/12 h dark with a constant temperature of 20°C. The 4°C cold treatment was started at dusk. Rosette material was harvested and pooled per sampling point.
H3K27me3 targets were extracted from previously published data (Lafos et al., 2011). In this study, H3K27me3 targets genes were identified with whole genome tiling arrays using undifferentiated meristematic cells from the shoot and differentiated leaf tissue from clavata3 mutant plants (Col-0 background). For this analysis the leaf samples were taken from plants grown for 9 weeks under short day conditions (8 h light/16 h dark).
The datasets were compared using the conditional formatting and filter function from Excel.
Bioinformatic Analyses of the Cold Regulation of Chromatin Modifier Genes Using Public Data
For the investigation of the regulation of chromatin genes during cold exposure, a list of chromatin regulator genes was extracted from the Chromatin Database (ChromDB) (version of the 30th of July, 2011) (Gendler et al., 2008). This list was overlapped with the lists of genes either differentially expressed or differentially spliced at any time point during cold exposure (Calixto et al., 2018) using the VennDiagram R package.
The analysis of the cold regulation of vernalization actors was performed by overlapping a list of genes involved in vernalization regulation with the lists of cold-regulated and cold-alternatively spliced genes previously described. The Venn diagram was plotted using the VennDiagram R package. The expression profiles of genes showing differential splicing upon cold exposure were generated and downloaded using the webservice at https://wyguo.shinyapps.io/atrtd2_profile_app/ (Zhang et al., 2017; Calixto et al., 2018). The translations of the differential usage transcripts were extracted from AtRTD2 (Zhang et al., 2017) and aligned to one another using the Needle algorithm (Madeira et al., 2019) to identify potential differences in amino acid sequences caused by cold exposure. The alignments were visualized using Multiple Align Show from the Sequence Manipulation Suite (Stothard, 2000).
Statistics
The statistical significance of overlaps between different groups of genes was calculated using http://nemates.org/MA/progs/overlap_stats.html. The significance of gene expression changes was analysed using an unpaired two-sided t-test, performed in RStudio (R Core Team, 2013; RStudio Team, 2016). The significance levels are presented as followed: *, 0.05 > p > 0.01; **, 0.01 > p > 0.001; and ***, p < 0.001.
Results
Enrichment of H3K27me3 Target Genes in Early, but not Late Cold-Inducible Genes
Expression of stress-inducible genes needs to be tightly controlled to prevent costly induction of plant defense responses in the absence of abiotic and biotic stresses. We hypothesized that stress-inducible genes are epigenetically silenced under non-stress conditions and therefore analyzed the prevalence of the main epigenetic silencing mark H3K27me3 in cold-regulated genes. We bioinformatically compared the H3K27me3 target genes identified in mature leaves (profiled under ambient temperature conditions) with genes regulated quickly after cold exposure (3 h) or after long-term (3 d) cold (Lafos et al., 2011; Calixto et al., 2018) (Figure 1, Supplemental Table 4). H3K27me3 target genes were significantly enriched among the early (3 h) cold-inducible genes, but not in later (3 d) inducible genes. The opposite pattern was revealed for genes downregulated in the cold: H3K27me3 target genes are underrepresented in the early repressed genes, but enriched in the late repressed genes (3 d).
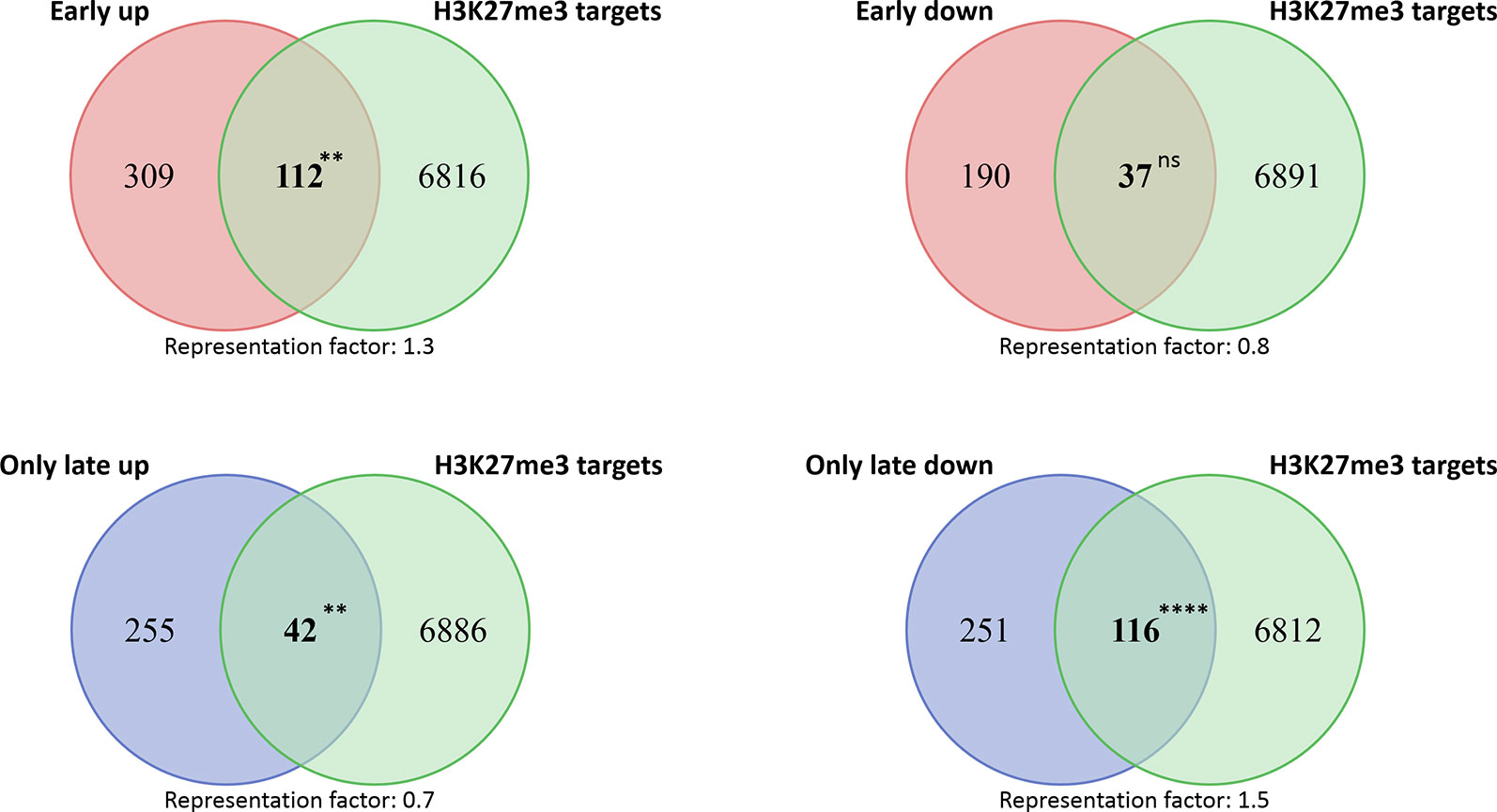
Figure 1 Venn diagrams showing the overlap of cold-regulated genes with H3K27me3 target genes. Four different cold-regulated gene groups, differing in their expression profile were extracted from Calixto et al. (2018). Genes already induced or repressed after 3 h in the cold are found in the group “Early up” or “Early down”, respectively. The “Only late” genes reflect genes which are not differentially expressed at 3 and 6 h and are induced or repressed at least six out of nine time points on day 3 in the cold (72 to 96 h). H3K27me3 targets were extracted from Lafos et al., 2011. Asterisks indicate ****P < 0.0001; **P < 0.01; ns stands for “not significant” and representation factor is given under each Venn diagram. For details see Supplemental Table 4.
To reveal whether early activation or late repression of genes is associated with changes in H3K27me3 or H3 occupancy we analyzed several cold-inducible H3K27me3 target genes for their occupancy in ambient temperature and during cold exposure (3 h and 3 d) using chromatin immunoprecipitation (Figure 2, Supplemental Figure 1). While we confirmed a previous study showing that COR15a displayed a reduction in H3K27me3, possibly due to reduction in general occupancy of H3, after cold exposure (Figure 2; Kwon et al., 2009), the level of H3K27me3 (or H3) was not significantly altered at the cold inducible genes ULTRAPETALA1 (ULT1), WRKY48 (Figure 2), TOLERANT TO CHILLING AND FREEZING1 (TCF1), and the glutaredoxin GRXS4 (Supplemental Figure 1). Similarly, the late repressed gene PINORESINOL REDUCTASE1 (PRR1) showed similar H3K27me3 (and H3) in ambient and cold temperatures (Figure 2). Thus, a change in cold-regulated gene expression is not necessarily associated with changes in H3K27me3 occupancy, at least at the analyzed genic regions.
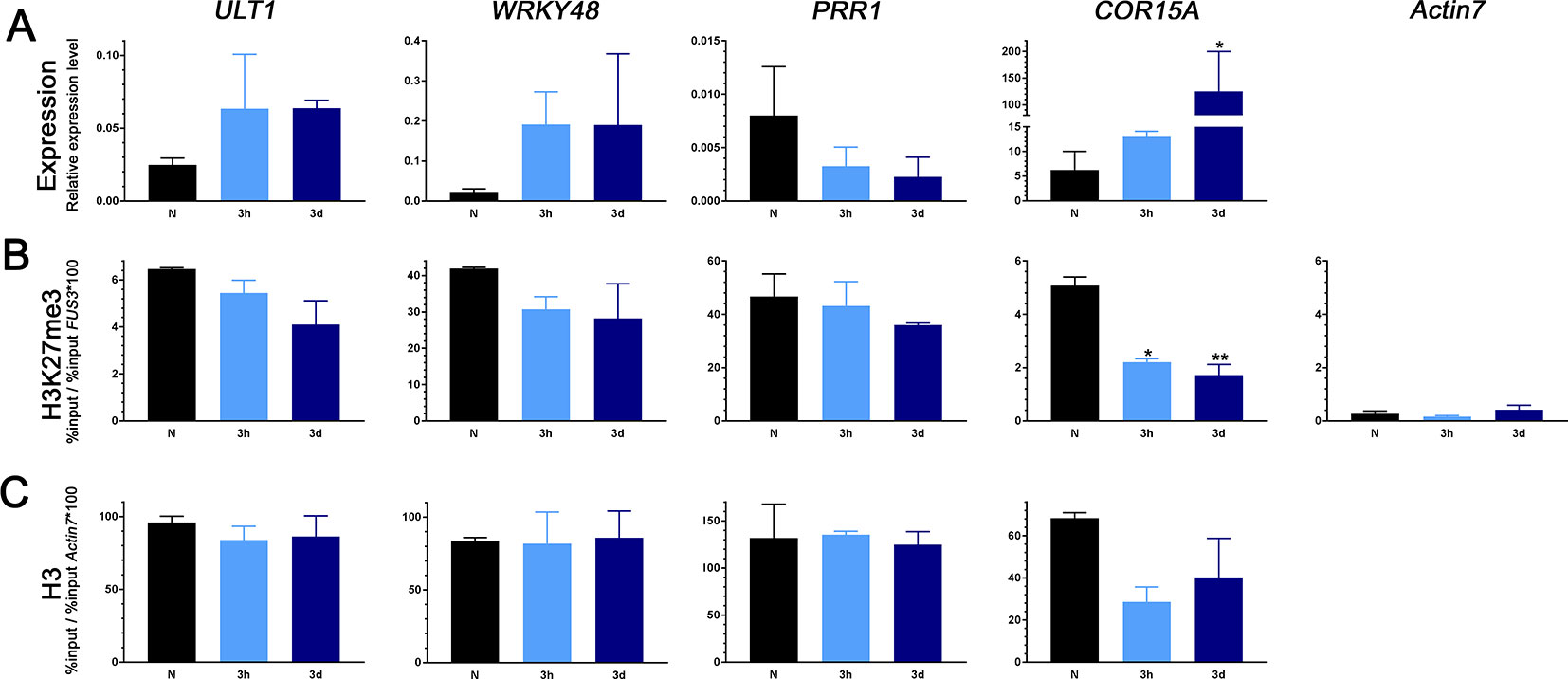
Figure 2 Gene expression changes, H3K27me3, and H3 levels of early upregulated (ULT1, WRKY48) and late downregulated (PRR1) genes as well as a well-characterized cold marker gene (COR15A) in plants exposed to cold. (A) RNA was isolated from 3-week-old seedlings grown at ambient temperature (N) and exposed to 4°C for 3 h or 3 days. Transcript levels for ULT1, WRKY48, PRR1, and COR15A were measured by reverse transcription and quantitative polymerase chain reaction (PCR). ACTIN2, PDF, and AT4G34270 were used as internal control. Error bars indicate ± s.e.m., n = 3 biological replicates. Test for significance by one-way ANOVA followed by a Dunnett's multiple comparison test. Significance levels are indicated relative to N: *P < 0.05. ChIP-PCR analysis of H3K27me3 (B) and H3 (C) occupancy. Chromatin was extracted from the same batch of seedlings used for RT-PCR after cross-linking and precipitated using H3K27me3 and H3 antibodies, respectively. The purified DNA was amplified by quantitative PCR. Results are presented as %input * 100/%input at the FUS3 locus for H3K27me3 and at the ACTIN7 locus for H3. For H3K27me3, ACTIN7 was used as a negative control. Error bars indicate ± s.e.m, n = 2 biological replicates. Test for significance by one-way ANOVA followed by a Dunnett's multiple comparison test. Significance levels are indicated relative to N: *P < 0.05; **P < 0.01. All primer sequences used for this experiment can be found in Supplemental Table 1. Additional analyzed genes and %input values are shown in Supplemental Figure 1.
Transcriptional Regulation and Alternative Splicing of Chromatin Genes During Cold Exposure
Although we did not observe changes in H3K27me3 occupancy at early cold-inducible genes after cold exposure, we wondered whether Pc-G, Pc-G associated and Pc-G antagonist genes and other chromatin genes are subject to transcriptional and/or post-transcriptional regulation upon cold-exposure. We analyzed this in two ways: first, we generated a RT-qPCR platform permitting expression analyses of Pc-G, Pc-G associated and Pc-G antagonist genes, histone and histone variant genes, and DNA methyltransferases and demethylases (in total 135 genes, see Methods and below). In addition, we extracted chromatin genes from the ChromDB (Gendler et al., 2008) and analyzed their transcriptional regulation and alternative splicing using a published dataset (Calixto et al., 2018). While chromatin regulatory genes were not significantly enriched among the genes differentially expressed in the cold (DE genes), they were highly enriched among the differentially alternatively spliced genes (DAS genes) (Figure 3). Only 14 out of 511 genes from ChromDB were both differentially expressed and spliced in the cold.
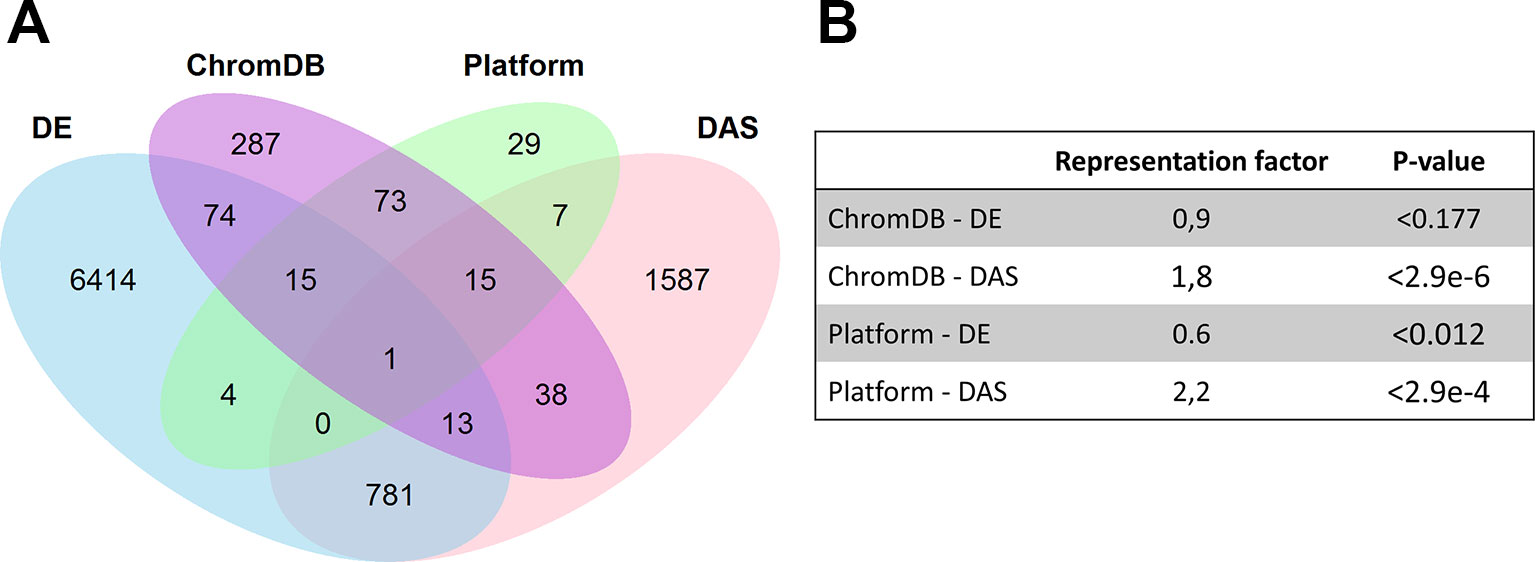
Figure 3 (A) Venn diagrams showing the overlaps of cold-regulated genes with chromatin regulatory genes. The overlaps were generated for genes extracted from the Chromatin Database (ChromDB), genes present on the RT-qPCR platform (Platform), differentially expressed (DE), and differentially alternatively spliced (DAS) genes, based on published data (Calixto et al., 2018). A gene was considered DE or DAS if it was differentially expressed or differentially spliced in at least one time point measured. (B) Significance of the overlap and representation factor for different pairwise comparisons. For details see Supplemental Table 5.
Nevertheless, interesting regulation patterns of several gene families were identified. Among the transcriptionally regulated genes were six histones and histone variants (HTR2, HTR6, HTR11; HTA6, HTA12; H1.3) and all three paralogues of the DNA demethylase DEMETER, DML1/ROS1, DML2, and DML3 (Supplemental Table 5). Genes involved in vernalization were not differentially expressed in response to cold, however, several vernalization regulators showed alternative splicing events (7 out of 15 genes) (Table 2). These include SR45, EMF2, VRN2, VRN5, VEL1, SWN, and HSL1. For most genes, alternative splicing resulted in repression or induction of alternative variants which encode slightly altered proteins, e.g. for VRN2 a cold-induced alternative transcript translates into a protein with an addition of the amino acids QL at position 304 which is within the highly conserved VEFS domain (Supplemental Figure 2). Interestingly, among the genes that were both differentially expressed and spliced was the H3K27me3 demethylase JMJ30. Its close paralogue, JMJ32 was also differentially expressed but not alternatively spliced. Overall, our bioinformatic analyses detected a widespread differential expression and splicing of chromatin regulators. Particularly, cold-induced alternative splicing of vernalization regulators is interesting and consistent with previously reported post-transcriptional regulation of VRN2, FIE, CLF, and SWN (Wood et al., 2006). Whether the proteins generated by alternative splicing exhibit different functional properties or have different interaction partners remains to be discovered.
Expression of Genes Encoding Proteins Involved in Epigenetic Processes During Cold Acclimation and Deacclimation
To allow expression analyses of Pc-G, Trx-G, and associated genes, histone genes, and genes involved in DNA methylation under various conditions, we set up a RT-qPCR platform. The expression of the 144 selected genes was analyzed in samples from NA, ACC, and deacclimated (Deacc) plants after 2, 4, 6, 12, and 24 h of deacclimation. Due to extremely low expression levels throughout all samples, gene expression data for JUMONJI (jmc) domain-containing protein 14 (JMJ14) (AT4G20400), Maternal affect embryo arrest 27/(JMJ15) (AT2G34880), JMJ17 (At1g63490), JMJ18 (AT1G30810), JMJ26 (At1g11950), VP1/ABI3-like 3 (VAL3) (AT4G21550), Probably E3-ubiquitin protein ligase (DRIPH) (AT3G23060), Chromomethylase 1 (CMT1) (AT1G80740), and male-gamete-specific histone H3 (MGH3) (AT1G19890) were not further considered, resulting in 135 investigated genes.
Expression changes of these genes during cold acclimation and subsequent deacclimation are shown in five heat maps compiled according to the function of the respective proteins in epigenetic regulation. The corresponding normalized 2−ΔCt values are shown in Supplemental Table 6.
The expression of 9 out of 19 genes encoding JUMONJI-type and lysine specific 1A-type (LSD1) histone demethylases was significantly upregulated after cold acclimation. Out of these nine genes, JMJ11/ELF6, JMJ19, JMJ22, JMJ27, JMJ28, and JMJ30 showed the highest log2 fold change compared to NA (Figure 4). During deacclimation the expression of these genes decreased over time which is additionally illustrated in the comparison of gene expression levels at all deacclimation time points with the expression at ACC conditions (Supplemental Figure 3, Supplemental Table 7). However, most genes displayed a drop in expression after 4 h Deacc followed by a slight increase at 6 h Deacc. Almost all genes showed a significant downregulation of the expression relative to ACC after 24 h Deacc (Supplemental Figure 3) and no significant differences compared to NA conditions.
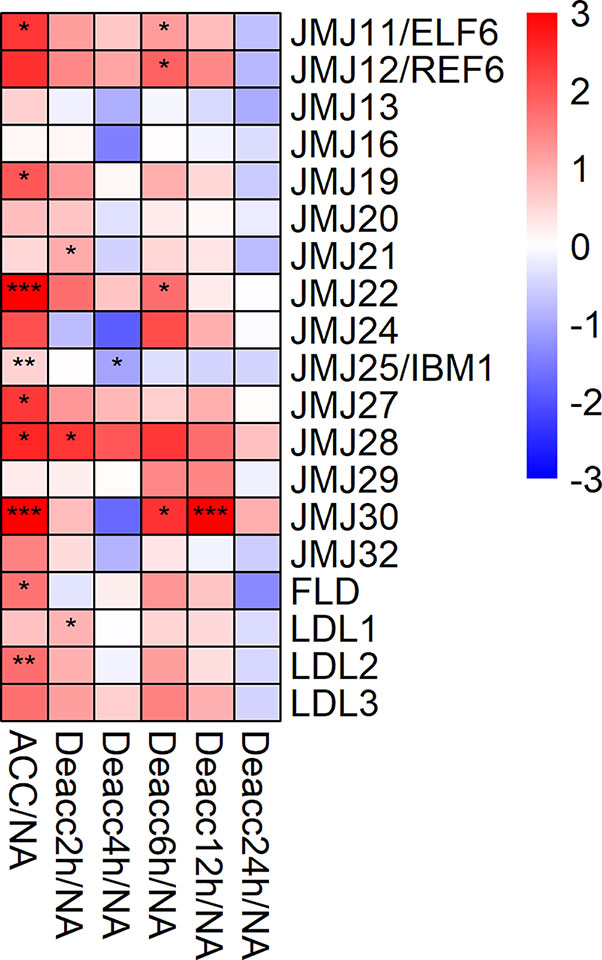
Figure 4 Expression changes of genes encoding JUMONJI-type and LSD1-type histone demethylases after cold acclimation (ACC) and after 2, 4, 6, 12, and 24 h of deacclimation (Deacc). Gene expression is presented as log2 fold change to non-acclimated conditions (NA) (Supplemental Table 7). The scale of log2 fold changes ranges from −3 (blue) to 3 (red) with a median of 0 (white). Significance levels are indicated relative to NA: ***P < 0.001; **P < 0.01; *P < 0.05.
Forty genes encoding members of the Pc-G related protein family were included in the expression analysis (Figure 5, Supplemental Figure 4). Similar to the JUMONJI-type and LSD1-type histone demethylase families, most Pc-G related genes displayed an upregulation during cold acclimation, with the highest upregulation for CLF, four WD40 repeat containing proteins MSI1-4 and VRN1. Altogether, 13 genes encoding Pc-G related proteins were significantly upregulated, while SWN, DRIP2/BMI1A, and VEL3 exhibited a downregulation at ACC. Most genes with a strong upregulation at ACC kept their higher expression levels over 12 h of deacclimation before they were significantly downregulated in comparison to ACC after 24 h (Supplemental Figure 4, Supplemental Table 7). After 6 h Deacc their expression was either increased transiently before returning to the initial NA expression levels or was continuously downregulated during the 24 h of deacclimation (Figure 5). Ten genes displayed a decrease in expression during deacclimation, which was significant in comparison to ACC over several time points, including AL3, CLF, FIE1, MSI1-MSI5, YY1, and VIN3 (Supplemental Figure 4). VEL2 was the only gene with a significant upregulation in comparison to ACC after 24 h Deacc (Supplemental Figure 4). DRIP2/BMI1A, on the other hand, was the only gene of the Pc-G related protein family with a significantly reduced expression at 24 h Deacc compared to NA.
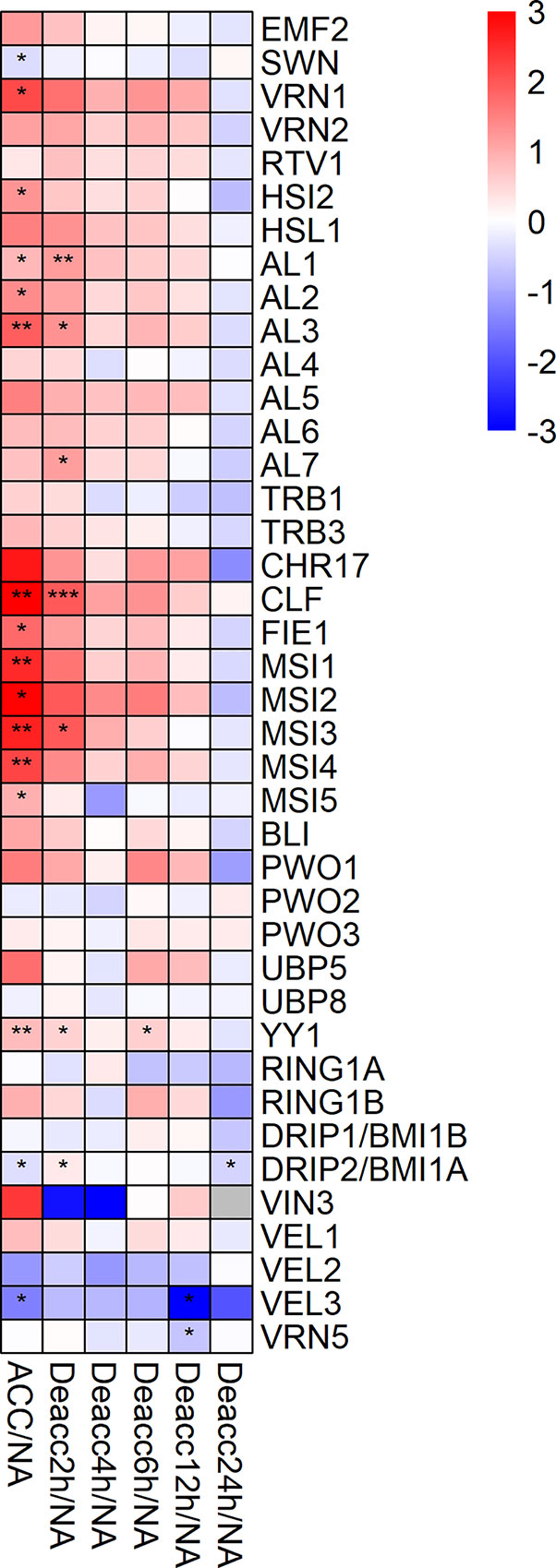
Figure 5 Expression changes of genes encoding proteins of the polycomb group (Pc-G) family after cold acclimation (ACC) and after 2, 4, 6, 12, and 24 h of deacclimation (Deacc). Gene expression is presented as log2 fold change to non-acclimated conditions (NA) (Supplemental Table 7). The scale of log2 fold changes ranges from −3 (blue) to 3 (red) with a median of 0 (white). Significance levels are indicated relative to NA: ***P < 0.001; **P < 0.01; *P < 0.05 *.
The expression of 22 genes encoding Trithorax-group (Trx-G) related proteins and chromatin remodelers was also investigated (Figure 6, Supplemental Table 7). Eight of these genes were significantly induced during cold acclimation (ATX1, ATX4, ULT2, CHR12, FAS1, FAS2, PDS5d, and SWI3A). After 2, 6, and 12 h Deacc only two, four, and two of these genes, respectively, were still significantly induced with only SWI3A showing a stable significant induction over almost all time points. A significant transiently changed expression over two time points during deacclimation compared to NA was only evident for PDS5e at 6 h and 12 h. At 24 h Deacc expression changes caused by cold acclimation were mostly reversed and expression of all genes reached similar levels as under NA conditions, except for ULT1, which was significantly downregulated. ATX5 displayed a transient upregulation in comparison to ACC conditions till 12 h Deacc (Supplemental Figure 5).
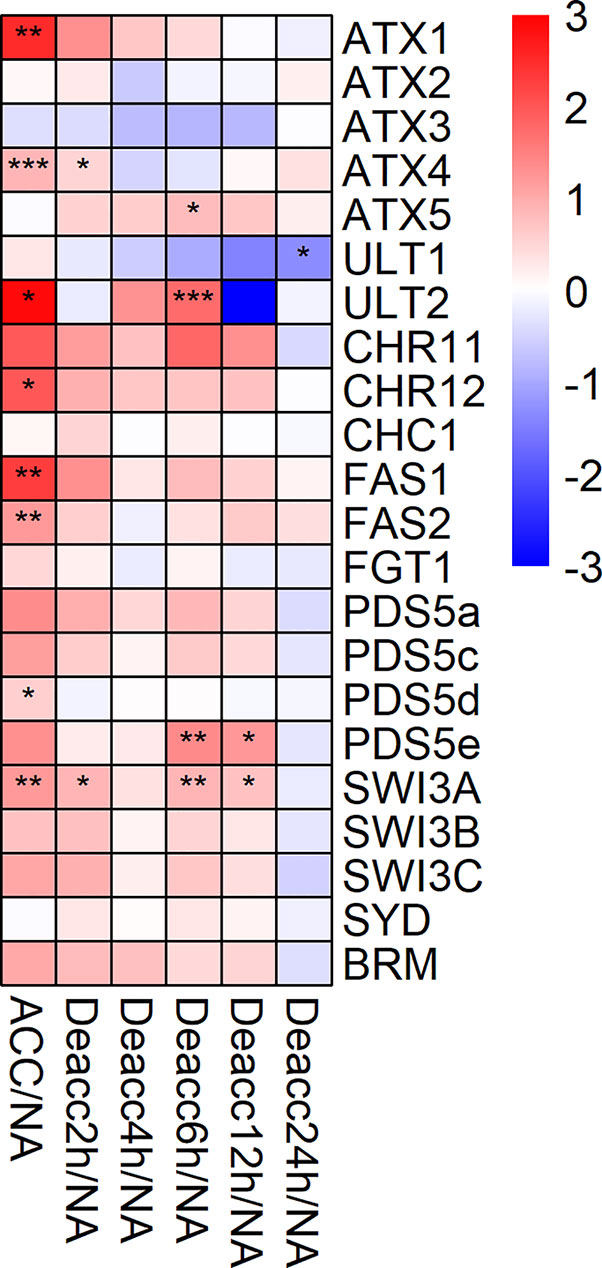
Figure 6 Expression changes of genes encoding proteins of the trithorax group (Trx-G) after cold acclimation (ACC) and after 2, 4, 6, 12, and 24 h of deacclimation (Deacc). Gene expression is presented as log2 fold change to non-acclimated conditions (NA) (Supplemental Table 7). The scale of log2 fold changes ranges from −3 (blue) to 3 (red) with a median of 0 (white). Significance levels are indicated relative to NA: ***P < 0.001; **P < 0.01; *P < 0.05 *.
Further, the expression of 25 genes encoding proteins acting in chromosome-nuclear envelope (Chr-NE) interactions, RNAi and DNA methylation was measured (Figure 7, Supplemental Figure 6). Five genes of this group were significantly differentially expressed at ACC compared to NA (Figure 7), SE, DCL1, ORTH1/VIM3, MET1, and DML3. Only DML3 was significantly reduced in its expression under ACC compared to NA conditions. For seven genes of this group expression decreased significantly at different time points of deacclimation compared to ACC, SUN2, SE, DCL1, ORTH1/VIM3, DRM3, MET1, and DML3 (Supplemental Figure 6, Supplemental Table 7). In contrast, especially CMT2, DRM1, and DME displayed a stable upregulation until 12 h Deacc or 6 h Deacc (Figure 7). DML3 was highly induced at almost all time points of deacclimation compared to ACC and was together with DRM3 and DML1/ROS1 still significantly upregulated compared to ACC at 24 h Deacc (Supplemental Figure 6).
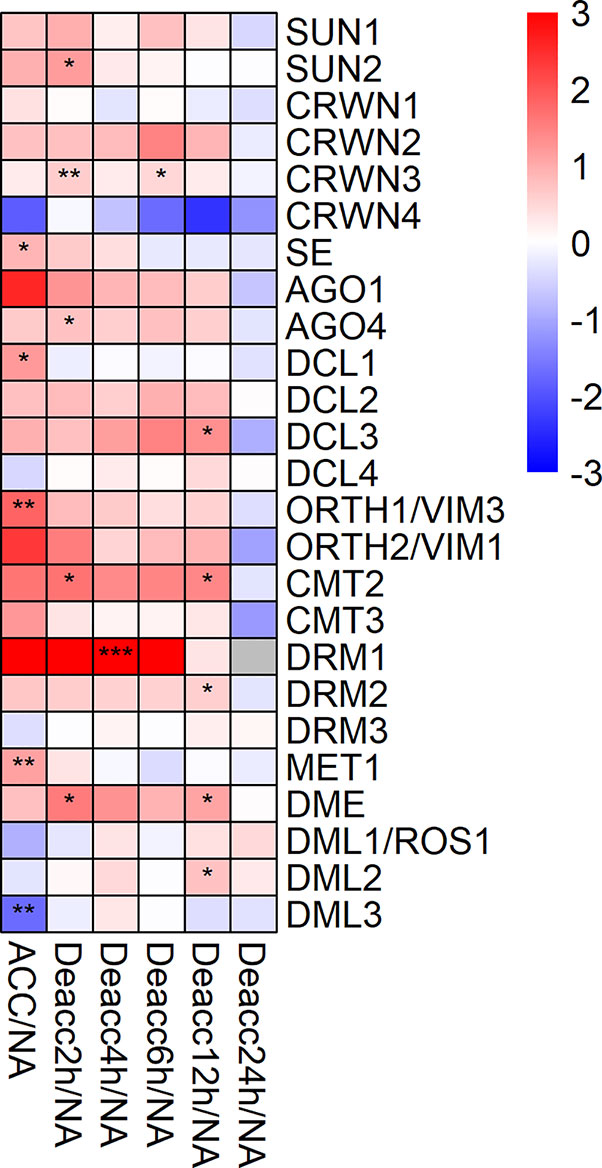
Figure 7 Expression changes of genes encoding proteins acting in chromosome-nuclear envelope (Chr-NE) interactions, RNA interference and methylation after cold acclimation (ACC) and after 2, 4, 6, 12, and 24 h of deacclimation (Deacc). Gene expression is presented as log2 fold change to non-acclimated conditions (NA) (Supplemental Table 7). The scale of log2 fold changes ranges from −3 (blue) to 3 (red) with a median of 0 (white). Significance levels are indicated relative to NA: ***P < 0.001; **P < 0.01; *P < 0.05.
Lastly, changes in expression levels of 29 genes that encode HAC, histone deacetylases (HDAC), or histone variants were investigated (Figure 8, Supplemental Figure 7). Fourteen of the selected genes were significantly upregulated after 3 days of cold acclimation, including HDA3, HTR1 and HTR2, HTR12, and HTR13, which showed the highest induction (Supplemental Table 7). Strikingly, this upregulation was still present after 2 h Deacc for 12 of these genes and became significant for three additional ones, HTA1, HTA10, and HTR5. About half of the genes displayed upregulated expression in comparison to NA over 6 h of deacclimation before they were downregulated after 24 h. Especially for H1.1, HTR1, and HTR5, a more stable upregulation was observed which was still significant after 12 h Deacc. These genes displayed an increase in expression after cold acclimation, followed by a slight decrease up to 4 h of deacclimation compared to NA conditions. From 4 to 12 h Deacc, expression increased again before returning to the NA level after 24 h, similarly to the pattern in most JUMONJI family genes.
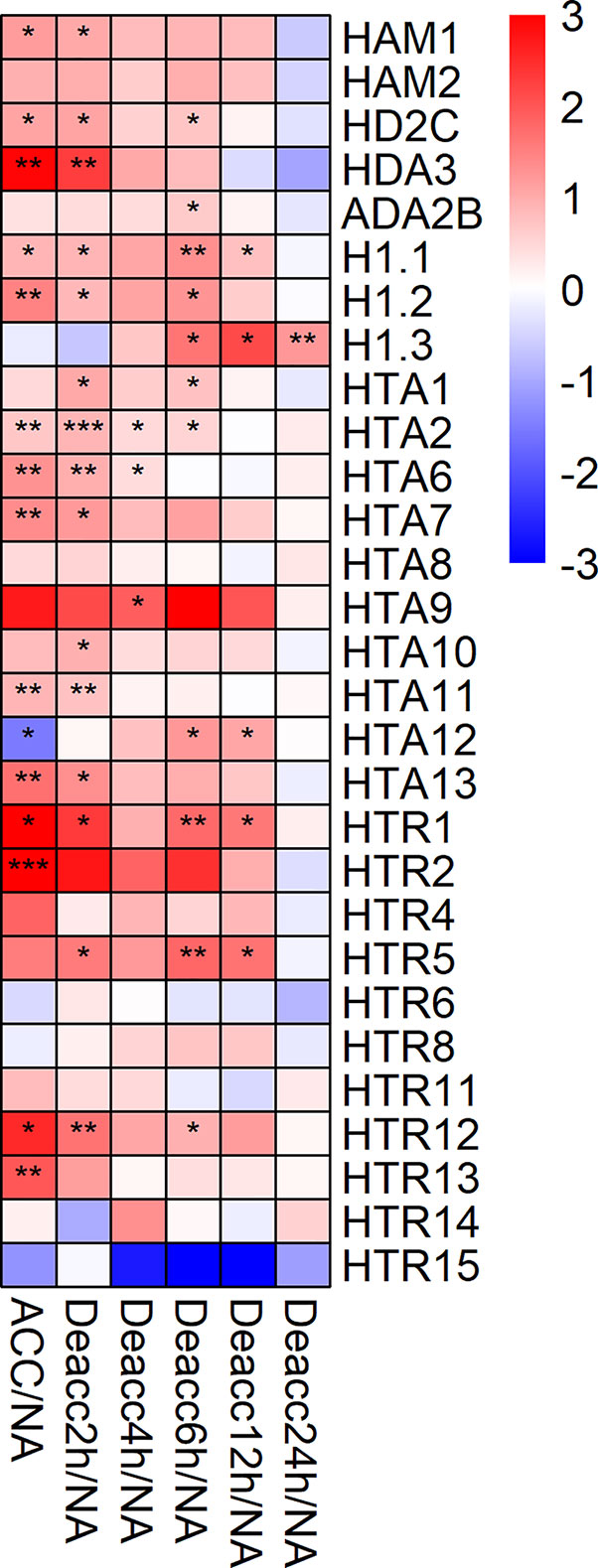
Figure 8 Expression changes of genes encoding histone acetyltransferases (HAC), deacetylases (HDAC), or histone variants after cold acclimation (ACC) and after 2, 4, 6, 12, and 24 h of deacclimation (Deacc). Gene expression is presented as log2 fold change to non-acclimated conditions (NA) (Supplemental Table 7). The scale of log2 fold changes ranges from −3 (blue) to 3 (red) with a median of 0 (white). Significance levels are indicated relative to NA: ***P < 0.001; **P < 0.01; *P < 0.05 *.
Interestingly, HTA12 was upregulated at later time points of deacclimation (6 and 12 h Deacc) after exhibiting the largest decrease in expression after cold acclimation in comparison to NA. Consistently, this gene was significantly induced compared to ACC along the whole deacclimation time course (Supplemental Figure 7). Lastly, H1.3 was the only investigated gene that displayed a decrease after cold acclimation and an increase throughout the 24 h Deacc (Supplemental Figure 7, Supplemental Table 7).
Discussion
H3K27me3 Preferentially Targets Early Cold Inducible and Late Repressed Genes
Chromatin and chromatin modifications contribute to the regulation of stress-regulated genes at various layers: (1) the repression of stress-inducible genes in non-stress conditions (by repressive chromatin), (2) the activation or repression of genes immediately after stress exposure (by the acquisition of active or repressive chromatin, respectively), (3) the sustained activation or repression in non-stress conditions after exposure to the stress, and (4) the transcriptional memory of a stress (in non-stress conditions), permitting primed gene regulation when exposed again to the stress (Friedrich et al., 2019). It is therefore conceivable that chromatin genes and the activity of their gene products are regulated at various layers during different phases of stress exposure and relief. Pc-G-mediated H3K27me3 is one of the key repressive chromatin modifications and targets thousands of genes in non-stress conditions, which are developmental regulators, tissue-specifically regulated genes and stress-responsive genes (Lafos et al., 2011). By bioinformatics comparison of H3K27me3 target genes and a detailed kinetic analysis of cold-regulated genes, we revealed an enrichment of H3K27me3 target genes among the early inducible genes. As the early inducible genes are required to trigger a cascade of gene regulatory networks to permit cold acclimation, it is likely particularly important to control their tight repression in non-stress conditions. Induction of H3K27me3-silenced genes may occur via different mechanisms: the enzymatic removal by histone demethylases, the addition of active modifications leading to a bivalent chromatin state, the exchange of histones by histone variants and the removal or sliding of nucleosomes by chromatin remodeling complexes. Interestingly, several genes encoding proteins potentially regulating dynamic changes in H3K27me3 are induced by cold, including the H3K27me3 demethylase JMJ30 (Gan et al., 2014), the Trx-G proteins ATX1 (a H3K4 methyltransferase) and ULT1 (Alvarez-Venegas et al., 2003; Carles and Fletcher, 2009), and diverse histone variants. At least for the analyzed cold-inducible H3K27me3 genes GRXS4, TCF1, ULT1, and WRKY48, we did not detect changes in H3K27me3 occupancy upon cold exposure, thus it is likely that these genes are activated by acquisition of activating marks or show only depletion of H3K27me3 at specific nucleosomes or in a tissue-specific manner. As activation of COR15A is associated with a reduction in nucleosome occupancy (which we did not detect for other genes), there are likely different, gene specific mechanisms at work. Our analyses also revealed that H3K27me3 target genes (identified in warm conditions) are overrepresented among the late repressed genes in the cold (Figure 1). We did not uncover changes in H3K27me3 occupancy upon cold exposure, at least for PRR1, suggesting that the mode of (further) repression is not accompanied by increased H3K27me3. Also here, H3K27me3 may be increased at specific nucleosomes, which is observed for the H3K27me3 target gene FLOWERING LOCUS C (FLC) which carries H3K27me3 in the warmth but a higher coverage/spreading of H3K27me3 upon cold exposure/vernalization (Schubert et al., 2006; De Lucia et al., 2008). The late repressed H3K27me3 target genes may be marked for long-term repression in the cold, similar to FLC.
Expression Analysis of Genes Mediating Epigenetic Changes
In general, our analysis indicated that cold acclimation had a strong influence on the expression of the selected epigenetics-related genes. This is in agreement with the finding that cold stress enhanced the accessibility of chromatin and bivalent histone modifications of active genes in potato (Zeng et al., 2019). After 24 h of deacclimation, expression of the investigated genes had largely returned to the NA status. This is in agreement with our earlier data on the expression of genes encoding transcription factors, also investigated by RT-qPCR, and global gene expression, investigated by microarray hybridization (Pagter et al., 2017).
Genes Encoding JUMONJI-Type and LSD1 Histone Demethylases are Upregulated During Cold Acclimation
JUMONJI-type and LSD1-type enzymes are histone demethylases. For several years histone methylation was thought to be irreversible until the discovery of human LSD1 (Mosammaparast and Shi, 2010). Although members of both enzyme families target histone methylation, they have different structures as well as targets. The JUMONJI family is defined by a JUMONJI C (JmjC) domain consisting of two histidines and one glutamate residue to chelate the catalytic iron, which is essential for its function (Mosammaparast and Shi, 2010). JmjC proteins are able to demethylate tri-methylated lysines, including those on H3K9, H3K27 and H3K36 (Mosammaparast and Shi, 2010; Gan et al., 2014). JmjC-domain proteins further reverse trimethylated H3K4 to its mono- or dimethylated forms (Iwase et al., 2007). LSD1 histone demethylases contain a SWIRM domain with an amine oxidase domain containing a substrate binding and an FAD-binding part and are only able to demethylate mono- and dimethylated lysines, such as H3K4me1/2 (Stavropoulos et al., 2006; Yang et al., 2006).
It is interesting to note that several members of both families of demethylases displayed an upregulation during cold acclimation before decreasing until 4 h Deacc followed by an increase until 12 h Deacc before returning to the initial NA gene expression levels after 24 h Deacc. This suggests that both demethylase classes are required during cold acclimation and deacclimation. The elevated expression of members of both gene families coincides with previous results, where a reduction in H3K9 methylation during short-term cold stress was described (Hu et al., 2012). Research has further shown that JUMONJI and LSD1 genes are linked to regulation of developmental transitions in A. thaliana. For example, histone demethylation of the FLC gene by JMJ30 and JMJ32 controls flowering at warm temperatures (Yang et al., 2010; Gan et al., 2014). Further, JUMONJI genes are upregulated under drought stress in peanut (Govind et al., 2009; Shen et al., 2014), in agreement with the upregulation found in ACC samples in this experiment. Lastly, JUMONJI proteins have been associated with changes in the circadian clock (Jones et al., 2010; Lu et al., 2011a). As the samples analyzed here were harvested throughout a time period of 24 h during deacclimation, circadian regulation could have contributed to the expression changes of e.g. JMJ30 (Lu et al., 2011b). Additional experiments will be necessary to clarify this contribution. Nevertheless, results for ACC and 24 h Deacc plants are not affected, as these samples were collected at the same time of day as the NA samples. Therefore we conclude that the majority of the investigated histone demethylases of the JUMONJI-type and LSD1 family are upregulated during cold acclimation.
Pc-G Proteins are Involved in Epigenetic Changes During Cold Acclimation and Deacclimation
The Pc-G gene family was discovered in Drosophila, where its members encode proteins able to repress the HOX genes (Lewis, 1978). The Pc-G gene family encodes a diverse set of proteins with a variety of molecular activities (Sauvageau and Sauvageau, 2010; Del Prete et al., 2015). Polycomb proteins act as multiprotein complexes, Polycomb Repressive Complex 1 (PRC1) and PRC2 in plants and PRC3 in humans (Schwartz and Pirrotta, 2007; Kleinmanns and Schubert, 2014). The PRC1 represses genes through mono-ubiquitination of histone H2A and chromatin remodeling (Kleinmanns and Schubert, 2014). Several PRC1 genes were investigated, such as RING1a, RING1b as well as EMBRYONIC FLOWER 1 (EMF1). PRC2 mediates the trimethylation of H3K27 (H3K27me3), which results in the repression of transcription through changes in chromatin organization (Kleinmanns and Schubert, 2014; Del Prete et al., 2015).
Most genes encoding proteins of the Pc-G displayed an upregulation during cold acclimation followed by either a relatively stable expression or decreased expression during deacclimation. Polycomb proteins have been linked to abiotic and biotic stresses in A. thaliana. WD-40 repeat containing protein MSI1 negatively regulates drought-stress responses in A. thaliana and a knockout of this gene confers increased drought tolerance (Alexandre et al., 2009).
Furthermore, H3K27me3 and/or H3 levels decrease at the cold-inducible genes COR15A and GOLS3 during cold stress. Upon transfer to ambient temperatures low H3K27me3 were maintained while COR15A and GOLS3 were repressed again. Thus, H3K27me3 is not sufficient to inhibit transcription, but the gene activation rather leads to H3K27me3/H3 removal (Kwon et al., 2009).
Further research on the regulation of cold-responsive genes by proteins of the Pc-G showed that EMF1 and EMF2 repress several cold-regulated genes such as COR15A and CBF1 (Kim et al., 2010b). Similarly, MSI4/FVE was identified in a screen for repressors of COR15A and loss of FVE leads to higher freezing tolerance in ACC plants (Kim et al., 2004).
As many cold-inducible genes carry the PRC2 mark H3K27me3 in the warmth, these genetic analyses are consistent with an important function for Pc-G proteins in prevention of precocious expression of cold-inducible genes. Although we found an increase in the expression of most PRC2 genes during cold acclimation, PRC2 occupancy analyses during cold acclimation will be required to reveal their presence on the cold-inducible genes. Results collected in this work suggest that a higher expression of genes encoding specific PRC2 subunits, such as WD-40 repeat-containing proteins (MSI1-MSI5), at ACC conditions might have led to an increase or redistribution of H3K27me3, resulting together with other changes in the increased freezing tolerance of A. thaliana. Supporting this hypothesis, induction of Pc-G genes during cold acclimation has also been reported previously. Brassica oleracea displayed induced expression of alfin-like transcription factors, which are interactors of PRC1, during 24 h at 4°C (Kayum et al., 2016) which is consistent with the observed induction of alfin-like genes in our study.
PRC1 and PRC2 proteins are central regulators of vernalization (Song et al., 2012). The only reported Pc-G protein induced by cold is VERNALIZATION INSENSITIVE 3 (VIN3) which is only induced after prolonged cold (at least 10 d) (Sung and Amasino, 2004; Kim et al., 2010a). We did not observe this induction as plants only experienced a 3-day cold period, however, a cold induction of VRN1, CLF, VAL1, and FIE was shown in this work. In addition, several vernalization-related genes were regulated by alternative splicing in the cold, including the PRC2 genes SWN, VRN2, and EMF2 (Table 2). Importantly, all of the alternatively spliced transcripts result in proteins with modified amino acid sequence. Whether these variants have a different function, stability or interaction partners remains to be determined. Wood et al. (2006) revealed that Pc-G proteins are also regulated at the post-translational level as VRN2, CLF, FIE, and SWN showed higher protein abundance after prolonged cold, while no changes in steady-state mRNA levels are detected. In conclusion, our and previous work suggest that Pc-G genes are regulated at the transcriptional, post-transcriptional (alternative splicing), and post-translational level. As the PRC2-mediated H3K27me3 appears to be a major mark repressing cold-regulated genes, tight regulation of PRC2 in the cold is important. Whether PRC2 regulation relates to cold acclimation and chilling/freezing tolerance in addition to vernalization remains to be determined.
The Stress-Responsive ATX1 Gene of the Trx Group is Cold Induced
Trx-G proteins act as antagonists to Pc-G and activate Pc-G target gene transcription by depositing H3K4me3 (Del Prete et al., 2015). Consequently, the activity of these genes must be finely tuned by opposing actions of these protein complexes (Del Prete et al., 2015). Further Trx-G proteins can also trimethylate H3K36 to activate transcription of target genes and act as an ATP-dependent chromatin remodeling complex, such as proteins containing a SWITCH or Brahma domain (Schuettengruber et al., 2011; Del Prete et al., 2015). Trx-G proteins such as ATX1 (an H3K4 methyltransferase), which plays a role in drought tolerance, have been linked to abiotic and biotic stresses in A. thaliana (Ding et al., 2011). This gene, together with ATX4, was also highly induced after cold acclimation. A loss of ATX1 expression results in decreased germination rates, larger stomatal apertures and thus higher transpiration rates, as well as lower drought tolerance (Ding et al., 2011). Further, binding of ATX1 to the gene 9-cis-epoxycarotenoid dioxygenase 3 (NCED3), encoding a protein catalyzing the limiting step in ABA synthesis, was observed, and a loss of ATX1 resulted in decreased NCED3 levels under drought stress (Ding et al., 2011). ATX1 has also been linked to the regulation of the salicylic acid and jasmonic acid pathways via WRKY70, which is a regulator of the plants defense pathway (Alvarez-Venegas et al., 2007).
The gene ATP-dependent helicase BRAHMA (BRM), encoding an ATP-dependent chromatin remodeling complex, displayed opposite effects to ATX1 under drought stress, resulting in increased drought tolerance when the gene was non-functional, through repression of ABA INSENSITIVE5 (Han et al., 2012). While BRM expression is not altered by cold, its paralog CHR12 is induced after cold acclimation. CHR12 is required to arrest growth after the plant is exposed to a stress (drought, heat, salt) (Mlynarova et al., 2007). Thus, its induction may be directly linking growth and stress responses. Three other genes that are highly induced during cold acclimation, FAS1, FAS2, and MSI1 encode proteins which form subunits of CHROMATIN ASSEMBLY FACTOR 1 (CAF-1) and are involved in maintaining the cellular organization of the shoot apical meristem (Kaya et al., 2001). SWI3A was the only gene of this group which was highly expressed during the first 12 h of deacclimation. It plays an essential role for plant growth and development (Zhou et al., 2003). These results show that there is a finely tuned regulation of several Trx-G genes and chromatin remodelers involved in abiotic stress response and stress release. Regulatory functions of Trx-G during cold stress have not been reported yet. Nevertheless, the involvement of many of these genes in stress responses in plants suggests a possible participation. Furthermore results of this work suggest that genes encoding Trx-G proteins play a role in cold acclimation in A. thaliana. Some proteins of Trx-G may also be involved in deacclimation, but further experiments would be required to investigate this.
DNA Methylation May Play a Role in Deacclimation
One group of DNA methylases adds a methyl group onto cytosine residues in higher eukaryotes and have been proposed to control gene expression in plants during development and regulate transposable elements and heterochromatin (Finnegan et al., 1996; Finnegan et al., 1998; Pavlopoulou and Kossida, 2007). Chromomethylases (CMT) are plant-specific and have been linked to symmetric and asymmetric methylation of DNA (Bartee et al., 2001). Changes in the levels of DNA methylation are known to occur during abiotic stresses; however their exact functions and effects are still unclear. For example, it has been observed that a hypermethylation of DNA occurs during salt stress in wheat (Peng and Zhang, 2009). Some of the investigated genes might be involved in deacclimation, e.g. CMT2 displayed a continued increase in expression up to 12 h Deacc. Furthermore, DNA demethylases such as DEMETER have been previously linked to plant stress responses and DME also showed increased expression during deacclimation. In addition, deletion of three DNA demethylases (DML1, DML2, DML3) resulted in increased susceptibility to fungal pathogens and therefore a participation in the biotic stress response of plants was proposed (Le et al., 2014). Interestingly, DML3 was significantly increased in comparison to ACC at all deacclimation time points and three out of four demethylases (DML1, DML2, DML3) were still upregulated at 12 and/or 24 h Deacc, suggesting that deacclimation is accompanied by a resetting of DNA methylation.
RNAi-related proteins are commonly found in the nucleus and cytoplasm and are well-known to act in post-transcriptional gene silencing in the cytoplasm (Castel and Martienssen, 2013). Dicer and DICER-LIKE (DCL) proteins are key regulators of small RNA biogenesis (RNAi). Only DCL1 was induced by cold acclimation whereas DCL3 was significantly upregulated only after 12 h Deacc. Expression analyses on DCL genes in rice showed differential responses comparing drought, cold, and salt stress (Liu et al., 2009). However, the cold response of DCL genes in rice differed compared to Arabidopsis, which may be due to the fact that rice, in contrast to Arabidopsis, is a chilling sensitive plants.
Histone Variants Respond Differentially and Strongly to Cold Acclimation and Deacclimation
Histone acetylation can occur on 26 potential lysine residues in a nucleosome (Lusser et al., 2001) and is a reversible process. Acetylation can alter the surface of nucleosomes and destabilize it to enhance binding of proteins to transcribed regions (Berger, 2007). Our results indicate an induction of both HDACs and HAC during cold acclimation and deacclimation. HDACs have been previously linked to responses to drought and salt stress, but not cold, in young rice seedlings (Hu et al., 2009). In Zea mays HDACs were induced during cold treatment, resulting in deacetylation of histone subunits H3 and H4. In addition, a direct activation of ZmDREB1 expression by ZmHDACs was suggested (Hu et al., 2011). In Arabidopsis, HDA6 is involved in cold acclimation through the regulation of cold-responsive genes (To et al., 2011; Kim et al., 2012). Similarly, the expression of HDA3 was highly induced during cold acclimation, and after 2 h Deacc, whereas HDA6 was not investigated. A similar expression pattern as for HDA3 was observed for HD2C. HD2A, HD2C, and HD2D interact with HDA6 and HDA19 in multiprotein complexes (Luo et al., 2017), and HD2C also interacts with BRAHMA, a chromatin remodeler involved in negative regulation of heat-responsive genes (Buszewicz et al., 2016). HD2C and the WD-40-repeat containing protein HOS15 interact before binding to the promoters of the cold-responsive genes COR15A and COR47. The cold induction of HOS15-mediated chromatin changes promotes HD2C degradation and is correlated with higher histone acetylation levels on the chromatin of COR genes. Additionally, HOS15 recruits CBF transcription factors to COR gene promoters (Park et al., 2018). The reported HD2C degradation seems to be contradictory to a higher HD2C gene expression under cold conditions, but an analysis of proteins levels will be necessary to compare these studies. Furthermore, the HAC Gcn5 (not investigated in this work) interacts with transcriptional adapter ADA2B, a transcriptional activator of HACs, and T-DNA insertions of GCN5 lower the induction of COR genes during cold acclimation (Stockinger et al., 2001; Vlachonasios et al., 2003). The ADA2B gene was also induced during 6 h Deacc in this work, pointing to a possible activation of HACs.
Additionally, the expression of several genes encoding histone variants has been investigated. Variants of H2A and H3 are incorporated into the chromatin during the interphase of the cell cycle to confer unique properties to the nucleosome (Deal and Henikoff, 2011). Histone variants of the canonical H2A (HTA2, HTA10, HTA13), H2A.Z (HTA8, HTA9, and HTA11) and H2A.W (HTA6, HTA7, HTA12) were included in the analysis, as well as the histone H1 variants H1.1, H1.2, H1.3, and HTR1 to HTR15 from the histone H3 (Jiang and Berger, 2017). The expression of most genes encoding histone variants was induced during cold acclimation and stayed upregulated during deacclimation. Studies on temperature stress have discovered that H2A.Z variants are regulated by a mild increase in ambient temperatures (Kumar and Wigge, 2010). A recent model stresses the importance of the H2A.Z status for the transcriptional regulation (Asensi-Fabado et al., 2017). Under cold conditions, H2A.Z deposition is increased resulting in higher plant sensitivity to changes in temperature.
An interesting expression pattern was detected for histone variant H1.3, which was not changed during cold acclimation, but was the only gene displaying a significant and stable upregulation after 6 to 24 h Deacc, indicating that H1.3 is not induced by cold, but specifically by deacclimation. H1.3 was also found to be upregulated after 24 h Deacc compared to ACC conditions in a comparison of three publicly available data sets using microarray and RNA-Seq data and was considered to be part of a core set of 25 common upregulated genes during deacclimation (Vyse et al., 2019). H1.3 is drought stress-induced in A. thaliana, and also responds to ABA treatment (Ascenzi and Gantt, 1997). A model for the action of histone 1 variants suggests that the small and mobile histone H1.3 replaces the canonical histone variants (H1.1 and H1.2) under stress conditions causing hypermethylation, but the influence of this process on transcriptional regulation and physiological responses is not clear yet (Asensi-Fabado et al., 2017). A similar pattern as for H1.3, but without induction at 24 h Deacc was found for HTA12. An induction of H1.3 and HTA12 during later deacclimation time points indicates a possible role of these genes in memorizing a previous stress event.
Overall, this study shows that many chromatin genes are dynamically transcriptionally and post-transcriptionally regulated during the plant cold response and deacclimation. Further work, especially genetic analyses, will be needed to investigate the function of these genes for both processes in more detail. In addition, the modifications set or removed by chromatin enzymes will require a detailed analysis. As hundreds of genes which are stably repressed in non-stress conditions and are targeted by H3K27me3 are activated within minutes after cold exposure, resetting of epigenetic information can be studied during the cold stress response. How this resetting results in memory of stress and/or induces vernalization, is an exciting question to address in the future.
Data Availability Statement
All datasets generated for this study are included in the article/Supplementary Material.
Author Contributions
KV, DS, and EZ designed the qRT-PCR platform. KV performed primer design, qRT-PCR experiments, and data analysis with input from EZ and DH. MP performed deacclimation experiments and prepared RNA. LF performed ChIP-qPCR and data analyses. LF and MR performed bioinformatic analyses. KV, DS, DH, and EZ wrote the manuscript.
Funding
This work was supported through funds for project A3 to DH and C7 to DS from the Collaborative Research Center 973 funded by the German Research Foundation (DFG, SFB 973). We gratefully acknowledge support to MP through the People Program (Marie‐Curie Actions) of the European Union's Seventh Framework Programme (FP7 People: Marie‐Curie Actions FP7‐MC‐IEF) under REA grant agreement 328,713. We acknowledge support by the German Research Foundation and the Open Access Publication Fund of the Freie Universität Berlin.
Conflict of Interest
The authors declare that the research was conducted in the absence of any commercial or financial relationships that could be construed as a potential conflict of interest.
Supplementary Material
The Supplementary Material for this article can be found online at: https://www.frontiersin.org/articles/10.3389/fpls.2020.00039/full#supplementary-material
References
Alexandre, C., Möller-Steinbach, Y., Schönrock, N., Gruissem, W., Hennig, L. (2009). Arabidopsis MSI1 is required for negative regulation of the response to drought stress. Mol. Plant 2, 675–687. doi: 10.1093/mp/ssp012
Allis, C. D., Jenuwein, T. (2016). The molecular hallmarks of epigenetic control. Nat. Rev. Genet. 17, 487–500. doi: 10.1038/nrg.2016.59
Alvarez-Venegas, R., Pien, S., Sadder, M., Witmer, X., Grossniklaus, U., Avramova, Z. (2003). ATX-1, an Arabidopsis homolog of trithorax, activates flower homeotic genes. Curr. Biol. 13, 627–637. doi: 10.1016/S0960-9822(03)00243-4
Alvarez-Venegas, R., Abdallat, A. A., Guo, M., Alfano, J. R., Avramova, Z. (2007). Epigenetic control of a transcription factor at the cross section of two antagonistic pathways. Epigenetics 2, 106–113. doi: 10.4161/epi.2.2.4404
Ascenzi, R., Gantt, J. S. (1997). A drought-stress-inducible histone gene in Arabidopsis thaliana is a member of a distinct class of plant linker histone variants. Plant Mol. Biol. 34, 629–641. doi: 10.1023/A:1005886011722
Asensi-Fabado, M.-A., Amtmann, A., Perrella, G. (2017). Plant responses to abiotic stress: the chromatin context of transcriptional regulation. Biochim. Biophys. Acta (BBA) - Gene Regul. Mech. 1860, 106–122. doi: 10.1016/j.bbagrm.2016.07.015
Banerjee, A., Wani, S. H., Roychoudhury, A. (2017). Epigenetic control of plant cold responses. Front. In Plant Sci. 8, 1643–1643. doi: 10.3389/fpls.2017.01643
Bannister, A. J., Kouzarides, T. (2011). Regulation of chromatin by histone modifications. Cell Res. 21, 381–395. doi: 10.1038/cr.2011.22
Bartee, L., Malagnac, F., Bender, J. (2001). Arabidopsis cmt3 chromomethylase mutations block non-CG methylation and silencing of an endogenous gene. Genes Dev. 15, 1753–1758. doi: 10.1101/gad.905701
Baulcombe, D. C., Dean, C. (2014). Epigenetic regulation in plant responses to the environment. Cold Spring Harbor Perspect. Biol. 6, a019471. doi: 10.1101/cshperspect.a019471
Berger, S. L. (2007). The complex language of chromatin regulation during transcription. Nature 447, 407–412. doi: 10.1038/nature05915
Buszewicz, D., Archacki, R., Palusinski, A., Kotlinski, M., Fogtman, A., Iwanicka-Nowicka, R., et al. (2016). HD2C histone deacetylase and a SWI/SNF chromatin remodelling complex interact and both are involved in mediating the heat stress response in Arabidopsis. Plant Cell Environ. 39, 2108–2122. doi: 10.1111/pce.12756
Byun, Y. J., Koo, M. Y., Joo, H. J., Ha-Lee, Y. M., Lee, D. H. (2014). Comparative analysis of gene expression under cold acclimation, deacclimation and reacclimation in Arabidopsis. Physiol. Plant. 152, 256–274. doi: 10.1111/ppl.12163
Calixto, C. P. G., Guo, W., James, A. B., Tzioutziou, N. A., Entizne, J. C., Panter, P. E., et al. (2018). Rapid and dynamic alternative splicing impacts the Arabidopsis cold response transcriptome. Plant Cell 30, 1424–1444. doi: 10.1105/tpc.18.00177
Carey, N., Marques, C. J., Reik, W. (2011). DNA demethylases: a new epigenetic frontier in drug discovery. Drug Discovery Today 16, 683–690. doi: 10.1016/j.drudis.2011.05.004
Carles, C. C., Fletcher, J. C. (2009). The SAND domain protein ULTRAPETALA1 acts as a trithorax group factor to regulate cell fate in plants. Genes Dev. 23, 2723–2728. doi: 10.1101/gad.1812609
Castel, S. E., Martienssen, R. A. (2013). RNA interference (RNAi) in the nucleus: roles for small RNA in transcription, epigenetics and beyond. Nat. Rev. Genet. 14, 100–112. doi: 10.1038/nrg3355
De Lucia, F., Crevillen, P., Jones, A. M. E., Greb, T., Dean, C. (2008). A PHD-polycomb repressive complex 2 triggers the epigenetic silencing of FLC during vernalization. Proc. Natl. Acad. Sci. U.S.A. 105, 16831–16836. doi: 10.1073/pnas.0808687105
Deal, R. B., Henikoff, S. (2011). Histone variants and modifications in plant gene regulation. Curr. Opin. Plant Biol. 14, 116–122. doi: 10.1016/j.pbi.2010.11.005
Del Prete, S., Mikulski, P., Schubert, D., Gaudin, V. (2015). One, two, three: polycomb proteins hit all dimensions of gene regulation. Genes 6, 520–542. doi: 10.3390/genes6030520
Ding, Y., Avramova, Z., Fromm, M. (2011). The Arabidopsis trithorax-like factor ATX1 functions in dehydration stress responses via ABA-dependent and ABA-independent pathways. Plant J. 66, 735–744. doi: 10.1111/j.1365-313X.2011.04534.x
Finnegan, E. J., Peacock, W. J., Dennis, E. S. (1996). Reduced DNA methylation in Arabidopsis thaliana results in abnormal plant development. Proc. Natl. Acad. Sci. U.S.A. 93, 8449–8454. doi: 10.1073/pnas.93.16.8449
Finnegan, E. J., Genger, R. K., Peacock, W. J., Dennis, E. S. (1998). DNA methylation in plants. Annu. Rev. Plant Physiol. Plant Mol. Biol. 49, 223–247. doi: 10.1146/annurev.arplant.49.1.223
Friedrich, T., Faivre, L., Baurle, I., Schubert, D. (2019). Chromatin-based mechanisms of temperature memory in plants. Plant Cell Environ. 42, 762–770. doi: 10.1111/pce.13373
Gan, E.-S., Xu, Y., Wong, J.-Y., Goh, J. G., Sun, B., Wee, W.-Y., et al. (2014). Jumonji demethylases moderate precocious flowering at elevated temperature via regulation of FLC in Arabidopsis. Nat. Commun. 5, 5098. doi: 10.1038/ncomms6098
Gendler, K., Paulsen, T., Napoli, C. (2008). ChromDB: the chromatin database. Nucleic Acids Res. 36, D298–D302. doi: 10.1093/nar/gkm768
Govind, G., Harshavardhan, V. T., Kalaiarasi, P. J., Iyer, D. R., Muthappa, S. K., Nese, S., et al. (2009). Identification and functional validation of a unique set of drought induced genes preferentially expressed in response to gradual water stress in peanut. Mol. Genet. Genomics 281, 591–605. doi: 10.1007/s00438-009-0432-z
Han, S.-K., Sang, Y., Rodrigues, A., Wu, M.-F., Rodriguez, P. L., Wagner, D. (2012). The SWI2/SNF2 chromatin remodeling ATPase BRAHMA represses abscisic acid responses in the absence of the stress stimulus in Arabidopsis. Plant Cell 24, 4892–4906. doi: 10.1105/tpc.112.105114
He, Y., Li, Z. (2018). Epigenetic environmental memories in plants: establishment, maintenance, and reprogramming. Trends Genet. 34, 856–866. doi: 10.1016/j.tig.2018.07.006
Hotchkiss, R. D. (1948). The quantitative separation of purines, pyrimidines, and nucleosides by paper chromatography. J. Biol. Chem. 175, 315–332.
Hu, Y., Qin, F., Huang, L., Sun, Q., Li, C., Zhao, Y., et al. (2009). Rice histone deacetylase genes display specific expression patterns and developmental functions. Biochem. Biophys. Res. Commun. 388, 266–271. doi: 10.1016/j.bbrc.2009.07.162
Hu, Y., Zhang, L., Zhao, L., Li, J., He, S., Zhou, K., et al. (2011). Trichostatin A selectively suppresses the cold-induced transcription of the ZmDREB1 gene in maize. PloS One 6, e22132. doi: 10.1371/journal.pone.0022132
Hu, Y., Zhang, L., He, S., Huang, M., Tan, J., Zhao, L., et al. (2012). Cold stress selectively unsilences tandem repeats in heterochromatin associated with accumulation of H3K9ac. Plant Cell Environ. 35, 2130–2142. doi: 10.1111/j.1365-3040.2012.02541.x
Iwase, S., Lan, F., Bayliss, P., De La Torre-Ubieta, L., Huarte, M., Qi, H. H., et al. (2007). The X-linked mental retardation gene SMCX/JARID1C defines a family of histone H3 lysine 4 demethylases. Cell 128, 1077–1088. doi: 10.1016/j.cell.2007.02.017
Jiang, D., Berger, F. (2017). Histone variants in plant transcriptional regulation. Biochim. Biophys. Acta - Gene Regul. Mech. 1860, 123–130. doi: 10.1016/j.bbagrm.2016.07.002
Jin, B., Li, Y., Robertson, K. D. (2011). DNA methylation: superior or subordinate in the epigenetic hierarchy? Genes Cancer 2, 607–617. doi: 10.1177/1947601910393957
Jones, M. A., Covington, M. F., Ditacchio, L., Vollmers, C., Panda, S., Harmer, S. L. (2010). Jumonji domain protein JMJD5 functions in both the plant and human circadian systems. Proc. Natl. Acad. Sci. U.S.A. 107, 21623–21628. doi: 10.1073/pnas.1014204108
Kawashima, T., Lorković, Z. J., Nishihama, R., Ishizaki, K., Axelsson, E., Yelagandula, R., et al. (2015). Diversification of histone H2A variants during plant evolution. Trends Plant Sci. 20, 419–425. doi: 10.1016/j.tplants.2015.04.005
Kaya, H., Shibahara, K. I., Taoka, K. I., Iwabuchi, M., Stillman, B., Araki, T. (2001). FASCIATA genes for chromatin assembly factor-1 in Arabidopsis maintain the cellular organization of apical meristems. Cell 104, 131–142. doi: 10.1016/S0092-8674(01)00197-0
Kayum, M. A., Park, J.-I., Ahmed, N. U., Saha, G., Chung, M.-Y., Kang, J.-G., et al. (2016). Alfin-like transcription factor family: characterization and expression profiling against stresses in Brassica oleracea. Acta Physiol. Plant. 38, 127. doi: 10.1007/s11738-016-2139-1
Kim, H. J., Hyun, Y., Park, J. Y., Park, M. J., Park, M. K., Kim, M. D., et al. (2004). A genetic link between cold responses and flowering time through FVE in Arabidopsis thaliana. Nat. Genet. 36, 167–171. doi: 10.1038/ng1298
Kim, D.-H., Zografos, B. R., Sung, S. (2010a). Vernalization-mediated VIN3 induction overcomes the LIKE-HETEROCHROMATIN PROTEIN1/POLYCOMB REPRESSION COMPLEX2-mediated epigenetic repression. Plant Physiol. 154, 949–957. doi: 10.1104/pp.110.161083
Kim, S. Y., Zhu, T., Sung, Z. R. (2010b). Epigenetic regulation of gene programs by EMF1 and EMF2 in Arabidopsis. Plant Physiol. 152, 516–528. doi: 10.1104/pp.109.143495
Kim, J. M., To, T. K., Seki, M. (2012). An epigenetic integrator: new insights into genome regulation, environmental stress responses and developmental controls by histone deacetylase 6. Plant Cell Physiol. 53, 794–800. doi: 10.1093/pcp/pcs004
Kim, J. M., Sasaki, T., Ueda, M., Sako, K., Seki, M. (2015). Chromatin changes in response to drought, salinity, heat, and cold stresses in plants. Front. In Plant Sci. 6, 114. doi: 10.3389/fpls.2015.00114
Kleinmanns, J. A., Schubert, D. (2014). Polycomb and Trithorax group protein-mediated control of stress responses in plants. Biol. Chem. 395, 1291–1300. doi: 10.1515/hsz-2014-0197
Kleinmanns, J. A., Schatlowski, N., Heckmann, D., Schubert, D. (2017). BLISTER regulates Polycomb-target genes, represses stress-regulated genes and promotes stress responses in Arabidopsis thaliana. Front. Plant Sci. 11, 1530. doi: 10.3389/fpls.2017.01530
Kotliński, M., Rutowicz, K., Kniżewski, Ł., Palusiński, A., Olędzki, J., Fogtman, A., et al. (2016). Histone H1 variants in Arabidopsis are subject to numerous post-translational modifications, both conserved and previously unknown in histones, suggesting complex functions of H1 in plants. PloS One 11, e0147908. doi: 10.1371/journal.pone.0147908
Kumar, S. V., Wigge, P. A. (2010). H2A.Z-containing nucleosomes mediate the thermosensory response in Arabidopsis. Cell 140, 136–147. doi: 10.1016/j.cell.2009.11.006
Kwon, C. S., Lee, D., Choi, G., Chung, W.-I. (2009). Histone occupancy-dependent and -independent removal of H3K27 trimethylation at cold-responsive genes in Arabidopsis. Plant J. 60, 112–121. doi: 10.1111/j.1365-313X.2009.03938.x
Lafos, M., Kroll, P., Hohenstatt, M. L., Thorpe, F. L., Clarenz, O., Schubert, D. (2011). Dynamic regulation of H3K27 trimethylation during Arabidopsis differentiation. PloS Genet. 7, e1002040. doi: 10.1371/journal.pgen.1002040
Law, J. A., Jacobsen, S. E. (2010). Establishing, maintaining and modifying DNA methylation patterns in plants and animals. Nat. Rev. Genet. 11, 204–220. doi: 10.1038/nrg2719
Le, T.-N., Schumann, U., Smith, N. A., Tiwari, S., Au, P. C. K., Zhu, Q.-H., et al. (2014). DNA demethylases target promoter transposable elements to positively regulate stress responsive genes in Arabidopsis. Genome Biol. 15, 458. doi: 10.1186/s13059-014-0458-3
Le, M. Q., Pagter, M., Hincha, D. K. (2015). Global changes in gene expression, assayed by microarray hybridization and quantitative RT-PCR, during acclimation of three Arabidopsis thaliana accessions to sub-zero temperatures after cold acclimation. Plant Mol. Biol. 87, 1–15. doi: 10.1007/s11103-014-0256-z
Lewis, E. B. (1978). A gene complex controlling segmentation in Drosophila. Nature 276, 565–570. doi: 10.1038/276565a0
Liu, Q., Feng, Y., Zhu, Z. (2009). Dicer-like (DCL) proteins in plants. Funct. Integr. Genomics 9, 277–286. doi: 10.1007/s10142-009-0111-5
Lu, F., Cui, X., Zhang, S., Jenuwein, T., Cao, X. (2011a). Arabidopsis REF6 is a histone H3 lysine 27 demethylase. Nat. Genet. 43, 715. doi: 10.1038/ng.854
Lu, S. X., Knowles, S. M., Webb, C. J., Celaya, R. B., Cha, C., Siu, J. P., et al. (2011b). The Jumonji C domain-containing protein JMJ30 regulates period length in the Arabidopsis circadian clock. Plant Physiol. 155, 906–915. doi: 10.1104/pp.110.167015
Luger, K., Mader, A. W., Richmond, R. K., Sargent, D. F., Richmond, T. J. (1997). Crystal structure of the nucleosome core particle at 2.8 Å resolution. Nature 389, 251–260. doi: 10.1038/38444
Luo, M., Cheng, K., Xu, Y., Yang, S., Wu, K. (2017). Plant responses to abiotic stress regulated by histone deacetylases. Front. In Plant Sci. 8, 2147. doi: 10.3389/fpls.2017.02147
Lusser, A., Kölle, D., Loidl, P. (2001). Histone acetylation: lessons from the plant kingdom. Trends Plant Sci. 6, 59–65. doi: 10.1016/S1360-1385(00)01839-2
Madeira, F., Park, Y. M., Lee, J., Buso, N., Gur, T., Madhusoodanan, N., et al. (2019). The EMBL-EBI search and sequence analysis tools APIs in 2019. Nucleic Acids Res. 47, W636–w641. doi: 10.1093/nar/gkz268
Mlynarova, L., Nap, J. P., Bisseling, T. (2007). The SWI/SNF chromatin-remodeling gene AtCHR12 mediates temporary growth arrest in Arabidopsis thaliana upon perceiving environmental stress. Plant J. 51, 874–885. doi: 10.1111/j.1365-313X.2007.03185.x
Mosammaparast, N., Shi, Y. (2010). Reversal of histone methylation: biochemical and molecular mechanisms of histone demethylases. Annu. Rev. Biochem. 79, 155–179. doi: 10.1146/annurev.biochem.78.070907.103946
Ng, S. S., Yue, W. W., Oppermann, U., Klose, R. J. (2009). Dynamic protein methylation in chromatin biology. Cell. Mol. Life Sci. 66, 407–422. doi: 10.1007/s00018-008-8303-z
Pagter, M., Alpers, J., Erban, A., Kopka, J., Zuther, E., Hincha, D. K. (2017). Rapid transcriptional and metabolic regulation of the deacclimation process in cold acclimated Arabidopsis thaliana. BMC Genomics 18, 731. doi: 10.1186/s12864-017-4126-3
Park, J., Lim, C. J., Shen, M., Park, H. J., Cha, J.-Y., Iniesto, E., et al. (2018). Epigenetic switch from repressive to permissive chromatin in response to cold stress. Proc. Natl. Acad. Sci. 115, E5400–E5409. doi: 10.1073/pnas.1721241115
Pavlopoulou, A., Kossida, S. (2007). Plant cytosine-5 DNA methyltransferases: structure, function, and molecular evolution. Genomics 90, 530–541. doi: 10.1016/j.ygeno.2007.06.011
Peng, H., Zhang, J. (2009). Plant genomic DNA methylation in response to stresses: potential applications and challenges in plant breeding. Prog. Natural Sci. 19, 1037–1045. doi: 10.1016/j.pnsc.2008.10.014
Penterman, J., Zilberman, D., Huh, J. H., Ballinger, T., Henikoff, S., Fischer, R. L. (2007). DNA demethylation in the Arabidopsis genome. Proc. Natl. Acad. Sci. U.S.A. 104, 6752–6757. doi: 10.1073/pnas.0701861104
R Core Team. (2013). R: A language and environment for statistical computing. R-3.4.3 ed. (Vienna, Austria: R Foundation for Statistical Computing).
Razin, A., Riggs, A. (1980). DNA methylation and gene function. Science 210, 604–610. doi: 10.1126/science.6254144
Roy, D., Paul, A., Roy, A., Ghosh, R., Ganguly, P., Chaudhuri, S. (2014). Differential acetylation of histone H3 at the regulatory region of OsDREB1b promoter facilitates chromatin remodelling and transcription activation during cold stress. PloS One 9, e100343. doi: 10.1371/journal.pone.0100343
RStudio Team (2016). RStudio: Integrated Development Environment for R. 1.1.423 ed. (Boston, MA: RStudio, Inc.).
Rutowicz, K., Puzio, M., Halibart-Puzio, J., Lirski, M., Kotlinski, M., Kroten, M. A., et al. (2015). A specialized histone H1 variant is required for adaptive responses to complex abiotic stress and related DNA methylation in Arabidopsis. Plant Physiol. 169, 2080–2101. doi: 10.1104/pp.15.00493
Sauvageau, M., Sauvageau, G. (2010). Polycomb group proteins: multi-faceted regulators of somatic stem cells and cancer. Cell Stem Cell 7, 299–313. doi: 10.1016/j.stem.2010.08.002
Schubert, D., Primavesi, L., Bishopp, A., Roberts, G., Doonan, J., Jenuwein, T., et al. (2006). Silencing by plant Polycomb-group genes requires dispersed trimethylation of histone H3 at lysine 27. EMBO J. 25, 4638–4649. doi: 10.1038/sj.emboj.7601311
Schuettengruber, B., Martinez, A.-M., Iovino, N., Cavalli, G. (2011). Trithorax group proteins: switching genes on and keeping them active. Nat. Rev. Mol. Cell Biol. 12, 799–814. doi: 10.1038/nrm3230
Schwartz, Y. B., Pirrotta, V. (2007). Polycomb silencing mechanisms and the management of genomic programmes. Nat. Rev. Genet. 8, 9–22. doi: 10.1038/nrg1981
Shen, Y., Conde E Silva, N., Audonnet, L., Servet, C., Wei, W., Zhou, D.-X. (2014). Over-expression of histone H3K4 demethylase gene JMJ15 enhances salt tolerance in Arabidopsis. Front. Plant Sci. 5, 290. doi: 10.3389/fpls.2014.00290
Shi, Y., Lan, F., Matson, C., Mulligan, P., Whetstine, J. R., Cole, P. A., et al. (2004). Histone demethylation mediated by the nuclear amine oxidase homolog LSD1. Cell 119, 941–953. doi: 10.1016/j.cell.2004.12.012
Song, J., Angel, A., Howard, M., Dean, C. (2012). Vernalization - a cold-induced epigenetic switch. J. Cell Sci. 125, 3723–3731. doi: 10.1242/jcs.084764
Spiker, S. (1982). Histone variants in plants. Evidence for primary structure variants differing in molecular weight. J. Biol. Chem. 257, 14250–14255.
Stavropoulos, P., Blobel, G., Hoelz, A. (2006). Crystal structure and mechanism of human lysine-specific demethylase-1. Nat. Struct. Mol. Biol. 13, 626–632. doi: 10.1038/nsmb1113
Stockinger, E. J., Mao, Y., Regier, M. K., Triezenberg, S. J., Thomashow, M. F. (2001). Transcriptional adaptor and histone acetyltransferase proteins in Arabidopsis and their interactions with CBF1, a transcriptional activator involved in cold-regulated gene expression. Nucleic Acids Res. 29, 1524–1533. doi: 10.1093/nar/29.7.1524
Stothard, P. (2000). The sequence manipulation suite: JavaScript programs for analyzing and formatting protein and DNA sequences. Biotechniques 28, 1102–1104. doi: 10.2144/00286ir01
Stroud, H., Otero, S., Desvoyes, B., Ramírez-Parra, E., Jacobsen, S. E., Gutierrez, C. (2012). Genome-wide analysis of histone H3.1 and H3.3 variants in Arabidopsis thaliana. Proc. Natl. Acad. Sci. U.S.A. 109, 5370–5375. doi: 10.1073/pnas.1203145109
Sung, S., Amasino, R. M. (2004). Vernalization in Arabidopsis thaliana is mediated by the PHD finger protein VIN3. Nature 427, 159–164. doi: 10.1038/nature02195
To, T. K., Nakaminami, K., Kim, J.-M., Morosawa, T., Ishida, J., Tanaka, M., et al. (2011). Arabidopsis HDA6 is required for freezing tolerance. Biochem. Biophys. Res. Commun. 406, 414–419. doi: 10.1016/j.bbrc.2011.02.058
Vlachonasios, K. E., Thomashow, M. F., Triezenberg, S. J. (2003). Disruption mutations of ADA2b and GCN5 transcriptional adaptor genes dramatically affect Arabidopsis growth, development, and gene expression. Plant Cell 15, 626–638. doi: 10.1105/tpc.007922
Vyse, K., Pagter, M., Zuther, E., Hincha, D. K. (2019). Deacclimation after cold acclimation - a crucial, but widely neglected part of plant winter survival. J. Exp. Bot. 70, 4595–4604. doi: 10.1093/jxb/erz229
Weber, C. M., Henikoff, S. (2014). Histone variants: dynamic punctuation in transcription. Genes Dev. 28, 672–682. doi: 10.1101/gad.238873.114
Widom, J. (1989). Toward a unified model of chromatin folding. Annu. Rev. Biophys. Biophys. Chem. 18, 365–395. doi: 10.1146/annurev.bb.18.060189.002053
Wood, C. C., Robertson, M., Tanner, G., Peacock, W. J., Dennis, E. S., Helliwell, C. A. (2006). The Arabidopsis thaliana vernalization response requires a polycomb-like protein complex that also includes VERNALIZATION INSENSITIVE 3. Proc. Natl. Acad. Sci. U.S.A. 103, 14631–14636. doi: 10.1073/pnas.0606385103
Yang, M., Gocke, C. B., Luo, X., Borek, D., Tomchick, D. R., Machius, M., et al. (2006). Structural basis for CoREST-dependent demethylation of nucleosomes by the human LSD1 histone demethylase. Mol. Cell 23, 377–387. doi: 10.1016/j.molcel.2006.07.012
Yang, W., Jiang, D., Jiang, J., He, Y. (2010). A plant-specific histone H3 lysine 4 demethylase represses the floral transition in Arabidopsis. Plant J. 62, 663–673. doi: 10.1111/j.1365-313X.2010.04182.x
Yelagandula, R., Stroud, H., Holec, S., Zhou, K., Feng, S., Zhong, X., et al. (2014). The histone variant H2A.W defines heterochromatin and promotes chromatin condensation in Arabidopsis. Cell 158, 98–109. doi: 10.1016/j.cell.2014.06.006
Zeng, Z., Zhang, W., Marand, A. P., Zhu, B., Buell, C. R., Jiang, J. (2019). Cold stress induces enhanced chromatin accessibility and bivalent histone modifications H3K4me3 and H3K27me3 of active genes in potato. Genome Biol. 20, 123. doi: 10.1186/s13059-019-1731-2
Zhang, Y., Reinberg, D. (2001). Transcription regulation by histone methylation: interplay between different covalent modifications of the core histone tails. Genes Dev. 15, 2343–2360. doi: 10.1101/gad.927301
Zhang, X., Yazaki, J., Sundaresan, A., Cokus, S., Chan, S. W. L., Chen, H., et al. (2006). Genome-wide high-resolution mapping and functional analysis of DNA methylation in Arabidopsis. Cell 126, 1189–1201. doi: 10.1016/j.cell.2006.08.003
Zhang, X., Clarenz, O., Cokus, S., Bernatavichute, Y. V., Pellegrini, M., Goodrich, J., et al. (2007). Whole-genome analysis of histone H3 lysine 27 trimethylation in Arabidopsis. PloS Biol. 5, 1026–1035. doi: 10.1371/journal.pbio.0050129
Zhang, R., Calixto, C. P. G., Marquez, Y., Venhuizen, P., Tzioutziou, N. A., Guo, W., et al. (2017). A high quality Arabidopsis transcriptome for accurate transcript-level analysis of alternative splicing. Nucleic Acids Res. 45, 5061–5073. doi: 10.1093/nar/gkx267
Zhou, C., Miki, B., Wu, K. (2003). CHB2, a member of the SWI3 gene family, is a global regulator in Arabidopsis. Plant Mol. Biol. 52, 1125–1134. doi: 10.1023/B:PLAN.0000004305.60407.8b
Zuther, E., Schulz, E., Childs, L. H., Hincha, D. K. (2012). Clinal variation in the non-acclimated and cold-acclimated freezing tolerance of Arabidopsis thaliana accessions. Plant Cell Environ. 35, 1860–1878. doi: 10.1111/j.1365-3040.2012.02522.x
Keywords: chromatin regulators, quantitative reverse transcription polymerase chain reaction platform, histones, cold acclimation, deacclimation
Citation: Vyse K, Faivre L, Romich M, Pagter M, Schubert D, Hincha DK and Zuther E (2020) Transcriptional and Post-Transcriptional Regulation and Transcriptional Memory of Chromatin Regulators in Response to Low Temperature. Front. Plant Sci. 11:39. doi: 10.3389/fpls.2020.00039
Received: 29 August 2019; Accepted: 13 January 2020;
Published: 07 February 2020.
Edited by:
Paula Casati, CONICET Center for Photosynthetic and Biochemical Studies (CEFOBI), ArgentinaReviewed by:
Federico Damian Ariel, Instituto de Agrobiotecnología del Litoral (IAL), ArgentinaElizabeth Finnegan, Commonwealth Scientific and Industrial Research Organisation (CSIRO), Australia
Julia Qüesta, Centre for Research in Agricultural Genomics (CRAG), Spain
Copyright © 2020 Vyse, Faivre, Romich, Pagter, Schubert, Hincha and Zuther. This is an open-access article distributed under the terms of the Creative Commons Attribution License (CC BY). The use, distribution or reproduction in other forums is permitted, provided the original author(s) and the copyright owner(s) are credited and that the original publication in this journal is cited, in accordance with accepted academic practice. No use, distribution or reproduction is permitted which does not comply with these terms.
*Correspondence: Daniel Schubert, dan.schubert@fu-berlin.de; Ellen Zuther, Zuther@mpimp-golm.mpg.de
†These authors have contributed equally to this work