- 1Instituto de Fisiología Vegetal (INFIVE), CONICET-UNLP, La Plata, Argentina
- 2Facultad de Ciencias Agrarias y Forestales, Universidad Nacional de La Plata, La Plata, Argentina
Phosphate (P) is characterized by its low availability and restricted mobility in soils, and also by a high redistribution capacity inside plants. In order to maintain P homeostasis in nutrient restricted conditions, plants have developed mechanisms which enable P acquisition from the soil solution, and an efficient reutilization of P already present in plant cells. Nitric oxide (NO) is a bioactive molecule with a plethora of functions in plants. Its endogenous synthesis depends on internal and environmental factors, and is closely tied with nitrogen (N) metabolism. Furthermore, there is evidence demonstrating that N supply affects P homeostasis and that P deficiency impacts on N assimilation. This review will provide an overview on how NO levels in planta are affected by P deficiency, the interrelationship with N metabolism, and a summary of the current understanding about the influence of this reactive N species over the processes triggered by P starvation, which could modify P use efficiency.
Introduction
It is not uncommon for plant roots to be exposed to temporary changes in local P availability. Considering the pivotal roles of this macronutrient in energy dynamics and metabolic regulation, P fluxes coordinately adjust to balance growth and development at the level of the whole plant. In the same way as it occurs with other mineral nutrients, both local signals acting on the cellular level, and long-distance or systemic signaling pathways, communicating internal nutrient status across different tissues and plant organs, must act coordinately to improve nutrient acquisition and internal utilization (Giehl et al., 2009; Lin et al., 2014; Ham et al., 2018; Wang et al., 2018; Ueda and Yanagisawa, 2019). The signaling compounds, such as NO and hormones, are involved in regulatory pathways when availability of nutrients is scarce (Giehl et al., 2009; Lei et al., 2011). In the case of P starvation, other signaling compounds come into play, including P itself, inositol polyphosphate, miRNAs, photosynthates, and calcium (Ruffel, 2018). Recently, a red-light signaling in the regulation of nutrient uptake and use was suggested, as it was found that expression levels of P starvation-responsive genes in Arabidopsis were modulated by PIF4/PIF5 and HY5 transcription factors, which activity is under the control of phytochromes (Sakuraba et al., 2018).
Reactive oxygen species (ROS) and NO have been recognized as early components in several mineral nutrient signaling events (Baxter et al., 2014; Kolbert, 2016). Even though there is a specific localization, timing and intensity of response depending on the depleted nutrient, it has been proposed that ROS and NO may be frequent elements in plant signal transduction cascades in response to nutrient imbalance (Xu et al., 2010; Zhu et al., 2019).
Sensing, signaling and the elaboration of acclimation responses will determine plant survival and performance in conditions of spatial and temporal variability of soil nutrient concentrations. To that end, in this review, we will discuss the current state of knowledge regarding NO functions in plants specifically under P restriction. We will focus on NO levels (and sources) in plants suffering from P deficiency, and its influence over the processes triggered by P starvation, which could modify P acquisition and use efficiency.
Phosphate in Soil and Plants
Plants are often exposed to growth-limiting levels of P during their life cycle. As this is also true in crops, in order to maintain yields in low P soils, chemical P fertilizers obtained from mineral deposits are applied in each crop cycle (Baker et al., 2015). Each year around 148 million tonnes of phosphoric rock are mined, and 90% of that is used for food production (Cordell et al., 2009). A fraction of the applied fertilizer is lost to run-off, consequently reaching seas and lakes and resulting in eutrophication processes (Childers et al., 2011). Thus, current agriculture generates massive P mobilization from mineral deposits to water bodies: a one-way flux of a resource considered “non-renewable” (Cordell et al., 2009; Elser and Bennett, 2011). Throughout the whole process of P extraction and use, two main critical points can be identified: depletion of P reserves from mines, affecting future global food production’s sustainability, and eutrophication of water reserves, recently reviewed by Schoumans et al. (2014). It is clear that the understanding of diverse processes involved in soil-plant P dynamics constitutes a key point, to face not only an agronomic problem, but also worldwide environmental and economic ones, with a direct impact on water-food-energy security (Jarvie et al., 2015).
The fact that P in soil can be found in a variety of chemical forms – as organic compounds, in the mineral pool as poorly soluble salts, and adsorbed to particle surfaces – turns this nutrient into one of the least available for plant nutrition in the rhizosphere (Raghothama and Karthikeyan, 2005; McLaughlin et al., 2011; Shen et al., 2011). P from organic sources needs to be released through mineralization processes carried out by soil microorganisms and enzymes present in root exudates. Roots are able to uptake P from the soil solution in the form of H2PO4– and HPO42–, and whereas the dynamic balance of available and non-available P is determined by diverse soil and climate conditions (Marschner, 2011), it is also modified by plant roots (Wang et al., 2015), microorganisms (Khan et al., 2016), and other physiological traits (Pang et al., 2018). Therefore in this complex matrix only a small portion of total P in soil is available for plants (Pierre and Parker, 1927). As a consequence only 10–20% of P-containing fertilizers applied are available in the short term (reviewed by Chien et al., 2012). Plants with limited P supply induce changes in pH, organic acids (carboxylates) concentration, and activity of enzymes in the rhizosphere (Hallama et al., 2019), improving P solubility and availability (Figure 1).
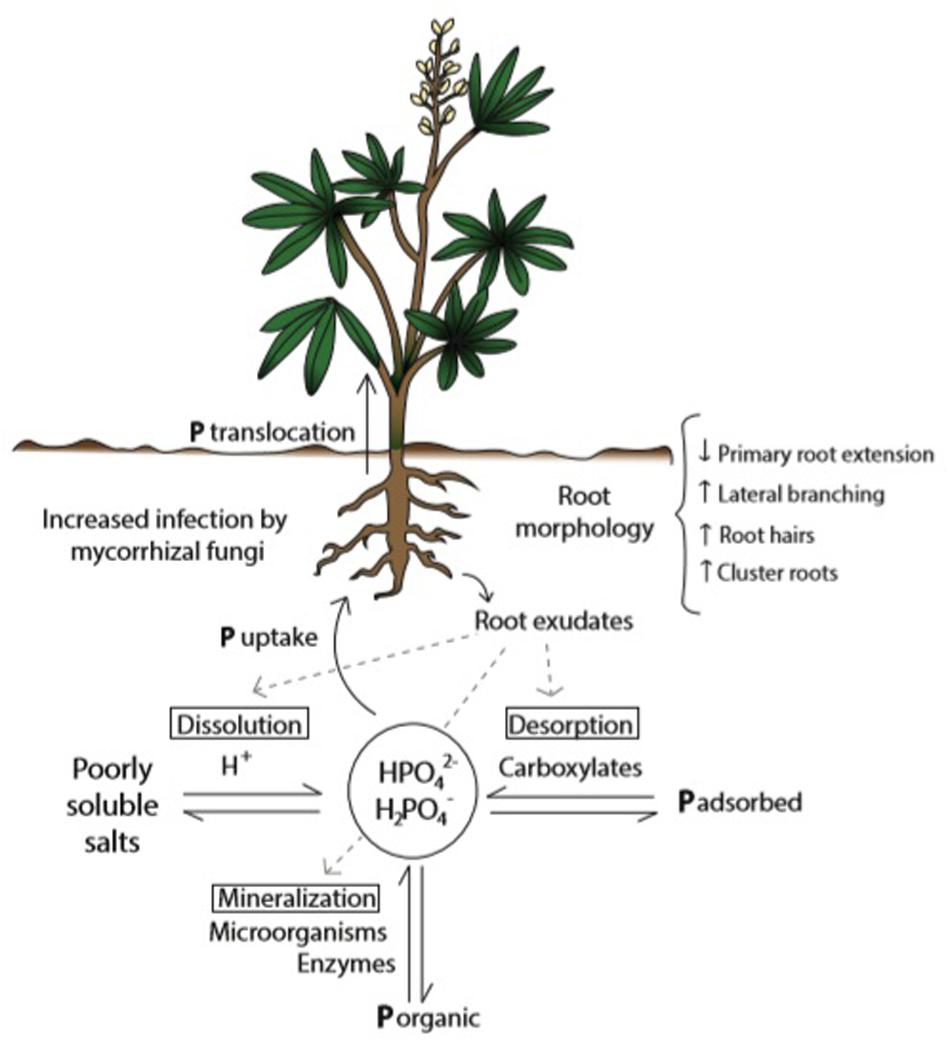
Figure 1. Plant’s strategies to improve P availability and uptake from soil solution in conditions of P scarcity. Acclimation responses tend to increase soil exploration (through morphological changes in roots), P desorption, dissolution, and interaction with microorganisms, as well as P internal remobilization (reallocation and interaction between P pools). P from the soil solution is taken up by the roots in the form of HPO42– and H2PO4–. Figure shows the dynamic equilibrium between available and not available P forms in soil, influenced by root exudations.
Phosphorus is easily remobilized internally, and this is of great agronomic importance. According to some authors, P use-efficiency in crops is determined not only by uptake efficiency but also other factors including utilization inside the plant, once the P is taken up, and the production of economically relevant plant tissues per unit of incorporated P (Schröder et al., 2011). P remobilization increases under external P restriction or during senescence. Remobilization occurs, in general, from leaves but also from proteoid roots as is the case of the harsh hakea where ∼85% of P can be reallocated (Shane et al., 2014). Vacuoles are the primary intracellular compartments for inorganic P (Pi) storage, with active participation in remobilization (Yang et al., 2017). In vacuoles at pH 5.0, monoanion H2PO4– predominates as the Pi species occurring in the efflux with a concentration in the millimolar range (Pratt et al., 2009). Using in vivo 31P-NMR, which allows for the discrimination between cytosolic-Pi and organelles-Pi pools, it has been found that, following the onset of P starvation, the Pi efflux from vacuoles is insufficient to compensate for a rapid decrease in the cytosolic Pi concentration. The sudden drop of cytosolic-Pi could be the first endogenous consequence to P starvation, triggering a signal transduction pathway which activates the P starvation rescue metabolism (Pratt et al., 2009). In addition to vacuole stock, when plants are exposed to P restriction, cell walls, membranes, RNA, and available organic compounds containing P, become important sources for the delivery of P to the actively growing tissues.
To better understand P fluxes in order to improve P use-efficiency by plants, a holistic interpretation of agroecosystems needs to be developed. This would identify the impacts of anthropic intervention through fertilization and diverse agricultural practices, soil P dynamics in connection with climate and microbiota, and plant physiological traits which modulate P acquisition and resulting internal reutilization (Macintosh et al., 2019).
Investigation of plant traits associated with agricultural P management is on the rise. New approaches are emerging such as the global-scale ecosystem services connected to soil fertility management (Macintosh et al., 2019) which is intended to overcome traditional agricultural perspectives, mainly focused on crop yield and short-term economic profits. In close connection with a global focus, P dynamic should also be studied at plant cellular and physiological levels. In this context, NO has influence on mechanisms which could increase soil exploration (Wu et al., 2014) and P availability (Ramos-Artuso et al., 2018), as well as mechanisms which could improve internal reutilization (Zhu et al., 2017). Thus, NO could be a key component in agro-ecosystem P flux interpretation, through modulation of P dynamics on plant physiological and plant-soil relationship levels.
Nitric Oxide in Soil and Plants
Nitric Oxide Synthesis in Plant Cells
Nitric Oxide can be endogenously produced by plant cells, but it can also be incorporated from the environment where it is generated as a result of activity of soil microorganisms. NO is a direct intermediate of nitrification (biological oxidation of ammonium, NH4+, to nitrate, NO3–) and denitrification processes (reduction of NO3–, to nitrogen gas, N2) carried out by microorganisms (Payne, 1976; Skiba et al., 1993; Feelisch and Martin, 1995). Soils are an important source of NO, and environmental conditions such as the presence of inorganic fertilizers can affect NO emissions. In this context, questions arise as to whether increased NO fluxes derived from N fertilization may affect growth and development, influence plant nutrition, and also affect a range of other plant responses (Lamattina et al., 2003). On the other hand, associative or symbiotic relationships between roots and microorganisms may contribute to NO production, and could also influence NO synthesis on each other (Molina-Favero et al., 2007).
Regarding NO synthesis, in mammals, nitric oxide synthase (NOS) enzymes use L-arginine, O2, and NADPH, to produce NO (Stuehr, 1999). In plants, different enzymatic and non-enzymatic sources can contribute to the generation of NO (Moreau et al., 2010; Fröhlich and Durner, 2011; Gupta et al., 2011; Mur et al., 2013; Astier et al., 2017), which have been classified as either oxidative or reductive pathways depending on the substrate involved (Gupta et al., 2011). NO production associated with NR, plasma membrane-associated nitrite:NO reductase (NiNOR) and other molibdo-enzymes (such as xanthine oxidoreductase, XOR), in addition to mitochondrial and chloroplastic electron transport chains, are all reductive pathways and depend on NO2– as a primary substrate. Meanwhile, NO production from arginine, polyamines or hydroxylamine, belongs to the oxidative pathways.
Progress has recently been made concerning the two main sources of NO, arginine-dependent (known as NOS-like activity) and NR in plant tissues. Jeandroz et al. (2016) searched for the presence of transcripts encoding NOS proteins in over 1000 species of land plants and did not find typical NOS sequences. In photosynthetic organisms, only a few algae species contained NOS orthologs (Jeandroz et al., 2016; Santolini et al., 2017), such as the green alga Ostreococcus tauri (Foresi et al., 2010). However, the presence of proteins structurally unrelated to known NOS, or the cooperation between proteins or peptides, which combined can form a complex with similar NOS activity, cannot be discarded in higher plants (Fröhlich and Durner, 2011; Corpas and Barroso, 2017). In this scenario, NO production from NR seems to gain more relevance, considering the importance of NO3– reduction and assimilation in plants. According to Chamizo-Ampudia et al. (2016), in Chlamydomonas reinhardtii, NR can supply electrons from NAD(P)H, through its diaphorase/dehydrogenase activity, to the molybdoenzyme NOFNiR (NO-forming nitrite reductase, also known as Amidoxime Reducing Component, ARC), which is in fact responsible for NO synthesis from NO2–, even in the presence of NO3–, condition under which NR is unable to do that. In addition, NR participates in the control of NO levels in cell by supplying electrons to the truncated hemoglobin THB1, which has dioxygenase activity (it can dioxygenate NO to produce NO3–). THB1 would then act by removing the very reactive NO and simultaneously inhibiting NR by uncoupling the electron transfer from NAD(P)H to NO3– (redirecting the electrons from FAD to THB1). THB1 then plays a dual role in NO detoxification and in the modulation of NR activity (Sanz-Luque et al., 2015). Further research on the conditions that regulate NO or NO2– production (such as the factors which favor the activity of NOFNiR and hemoglobins over NR) is required (Chamizo-Ampudia et al., 2017).
Over the last few years, research in the field of NO generation in plants has advanced. However, knowledge of the regulation of the multiple proposed pathways, especially under stress conditions, and in particular under mineral nutrient deprivation, is still limited. Different sources may come together, depending on stress conditions, substrate availability, species, and plant’s organs. Knowledge of the sources (and fates) of NO which operate under nutrient deficiency, in addition to the understanding of the specific roles of this molecule, are key tools to unraveling the mechanisms which trigger acclimation responses, where the modulation of endogenous levels of this molecule could be involved.
Nitric Oxide Targets in Plants
Nitric oxide may exert its biological functions through protein modifications, such as tyrosine nitration or S-nitrosation (also termed as S-nitrosylation), or through interaction with metalloproteins (metal-nitrosylation) (Figure 2), besides performing a broad spectrum of biochemical events through the interaction with hormones, and ROS among others (Gupta et al., 2020).
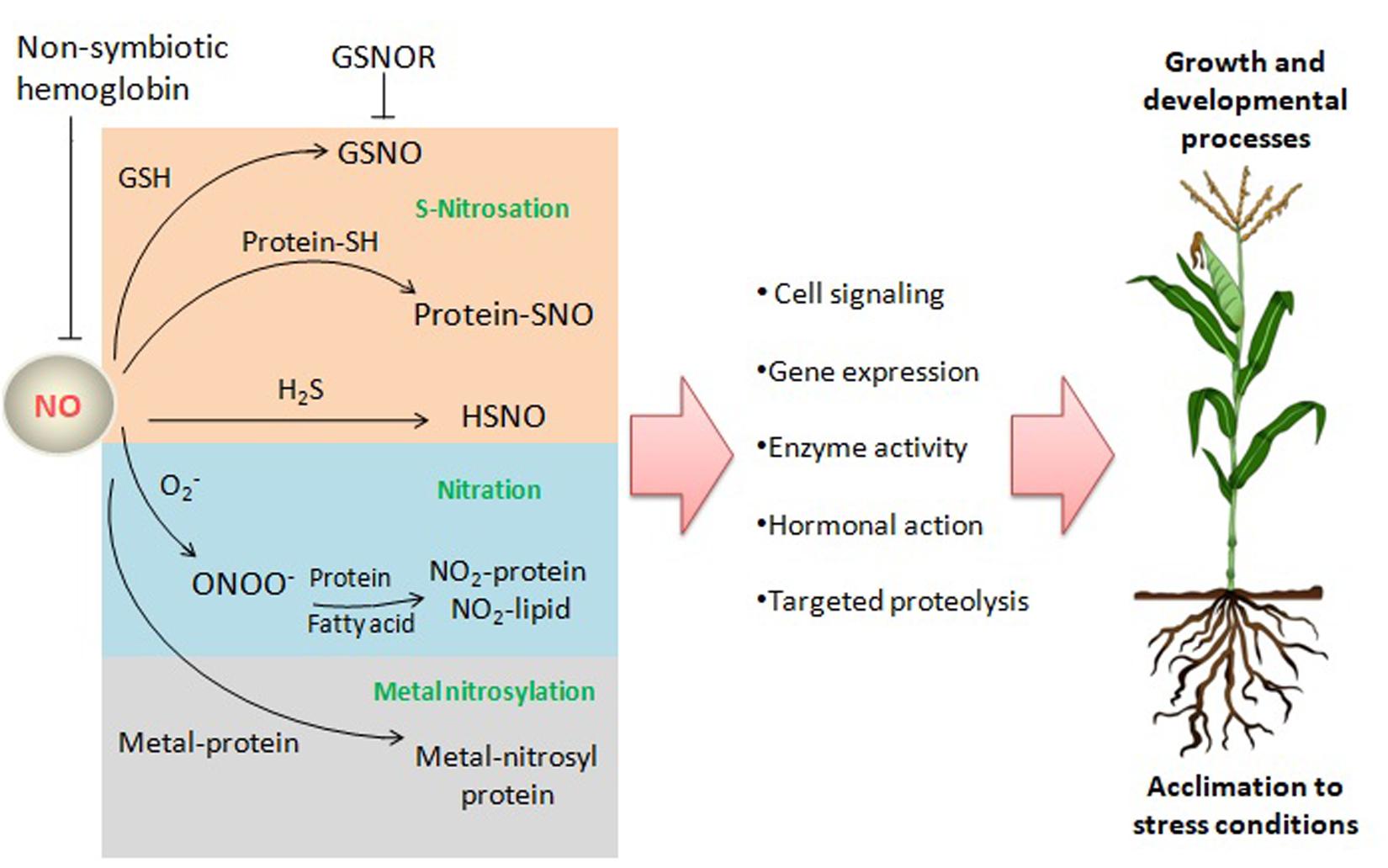
Figure 2. NO reactions and targets in plants. NO may exert its biological functions through interaction with ROS, the gasotransmitter H2S, proteins, and lipids leading to a broad range of biochemical events affecting signaling pathways and processes related to growth, development and acclimation to stress conditions. GSNO, S-nitrosoglutathione; GSNOR, GSNO reductase; O2–, superoxide anion; ONOO–, peroxynitrite; H2S, hydrogen sulfide; HSNO, thionitrous acid (the smallest S-nitrosothiol); Protein-SNO, nitrosated protein.
Although in animals the NO/cGMP-signaling (cyclic guanosine monophosphate) pathway has a main role in transmitting NO signal, plants have developed specific NO signaling different from other eukariotic lineages. According to recent studies, typical NO/cGMP signaling module is absent from representative plant species, while S-nitrosation has emerged as major NO-dependent signaling mechanisms in plants (Astier et al., 2019). Proteins and low-molecular-weight thiols can undergo S-nitrosation, where NO binds the thiol group of cysteinyl residues. S-nitrosation is a reversible post-translational modification by which NO can modulate protein activity.
S-nitrosoglutathione (GSNO) is the most abundant low-molecular-weight nitrosothiol and, in turn, it is considered to be a form of NO storage and long-distance transport (reviewed in Begara-Morales et al., 2019). NADPH-dependent thioredoxin reductase (NTR)/thioredoxin (Trx) system and GSNO reductase (GSNOR) control the extent of S-nitrosation through the regulation of GSNO levels and catalyzing denitrosation reactions (Begara-Morales and Loake, 2016).
Tyrosine nitration is mediated by reactive nitrogen species, such as ONOO– and nitrogen dioxide (NO2), formed as secondary products of NO metabolism in the presence of oxidants, such as superoxide radicals (O2–), hydrogen peroxide (H2O2), and transition metal centers (Radi, 2004). In a relevant position, Tyr nitration can alter protein function and conformation, impose steric restrictions, and also inhibit Tyr phosphorylation. The result may be a loss-of-function (if a large fraction of protein is nitrated in specific critical tyrosines) or a gain-of-function (a small fraction of nitrated protein can elicit a substantive biological signal) (Radi, 2004). Evidence shows that protein Tyr nitration is an important NO-dependent signaling mechanism in plants, as well as nitration of fatty acids and ribonucleic acids (Arasimowicz-Jelonek and Floryszak-Wieczorek, 2019).
It is necessary to understand NO effects in the context of a complex network of molecules (Kolbert et al., 2019). The interaction of NO with other small signaling molecules, such as ROS and hydrogen sulfide (H2S), contributes to the regulation of growth, development, and of biotic and abiotic stresses responses (Singh et al., 2019; Figure 2). So far, there is insufficient knowledge of the specific crosstalk involving RNS, ROS and H2S under P-scarcity.
These mechanisms may account, in part, for the broad spectrum of NO actions in plants exposed to P imbalances, including posttranslational modification of proteins, enzymes, transporters and transcription factors, which may participate, in addition to hormones, in some of the physiological responses summarized here.
The Levels of Nitric Oxide Are Influenced by P Deficiency
Wang et al. (2010) described a relationship between NO generation and P restriction in white lupin (Lupinus albus), where P deficiency enhanced NO production in primary and lateral root tips, with a bigger increase in juvenile and mature cluster roots (bottlebrush like structures). In searching for NO sources, root treatment with the mammalian NOS inhibitor (NG-nitro-L-arginine) and the XOR inhibitor (allopurinol) was found to reduce NO concentration in cluster roots, whereas with the NR inhibitor (tungstate), no effect of treatment was observed. Thus NOS-like activity and XOR may be two of the sources involved in NO production under P deficiency in white lupin.
In Arabidopsis thaliana, however, the NR double mutant (nia1,2) resulted in more sensitivity to P scarcity than WT plants, which indicated an impairment of the mechanisms involved in the acclimation to low P in these mutants. Moreover, P deprivation led to an increased NO production in roots from WT plants but not from nia1,2, which suggested a role for the NR pathway in NO production under P restriction conditions in Arabidopsis (Royo et al., 2015). In regards to soybean plants, P deprivation led to higher levels of NO in the leaves and an increase in NR activity as early as 24 h of P deprivation (Ramos-Artuso et al., 2019). Interestingly, the levels of total N, NO3–, NO2–, and the activities of other enzymes from N metabolism, were not affected. NR activity and NO generation may play a part in sensing P levels in cells triggering early metabolic responses to deal with P scarcity, as was suggested by the changes observed in the proteome in soybean leaves (Ramos-Artuso et al., 2019). Moreover, P deficiency induced an increase in NO level in rice roots (Zhu et al., 2016) where it was involved in cell-wall P reutilization, upstream of ethylene (Zhu et al., 2017).
Overall, NO seems to have a role in conditions of P scarcity in plants, not only in root response but also in shoot, where early changes are triggered as a consequence of P decrease. The two main ways of NO production in plants, NR and arginine-dependent, seem to be involved in the regulation of NO levels in the cell, but other potential sources (such as polyamines and other ways of NO2– reduction) cannot be ruled out, and may also contribute to NO synthesis in conditions of P restriction. Future research will clarify this variable scenario dependent on multiple factors, including plant species, organ, source of N, and the level of P in the cell.
Phosphorus Deficiency Affects N Metabolism and Impacts on NO Levels: Potential Regulatory Implications
It is known that P deprivation leads to alterations in N assimilation (Rabe and Lovatt, 1986; Rufty et al., 1993), but how these changes in N metabolism modify NO levels in cell and affect signaling events, is still unknown. Recent studies of P deficiency in plants have shown an increase in NO levels and suggest this molecule takes part in some responses related to acclimation conditions. Taking into account this scenario, changes in NO levels may either result in or be the result of alteration in N metabolism under conditions of P scarcity since N assimilation and NO generation are strongly connected. As mentioned before NO2– and arginine, both derived from N assimilation and metabolism, are substrates for NO synthesis, and both the amount and the form of N supply (NO3– or NH4+) could affect NO generation (Buet et al., 2019). Zhu et al. (2016) observed an increased NO content in roots from two rice cultivars under P-deficient conditions, where the feeding with NH4+ significantly increased the NO level as compared with NO3– treatment. The effect of NH4+ over the NO levels was also observed in Arabidopsis plants with Fe deficiency (Zhu et al., 2019). In the case of soybean plants fed with NH4+, N assimilation occurs mainly in the roots, where the incorporation into amino acids is faster and stronger than with NO3– as N source. This suggests a protective role, to avoid accumulation of high levels of NH4+, which can produce deleterious effects on cellular functions (Oliveira et al., 2013). In P-deficient leaves, it has been proposed that arginine biosynthesis may act as a protective mechanism for NH4+ detoxification (Rabe and Lovatt, 1986; Rufty et al., 1993). This highlights the need for an evaluation of arginine and polyamines levels in roots, as potential sources of NO in conditions of P deficiency.
In soybean (Glycine max), long-term P deprivation (20 days) led to several changes in N assimilation: rates of 15NO3– uptake and net translocation of 15N from roots to shoots decreased, resulting in the alteration of NO3– assimilation. Asparagine accumulated to high levels in stems and roots, and arginine accumulated to high levels in leaves was also observed (Rufty et al., 1993). Arginine levels increased in leaves of rough lemon (Citrus limon) and summer squash (Cucurbita pepo), when grown under P deficient conditions (Rabe and Lovatt, 1986). Nitrite levels increased in WT Arabidopsis roots after exposure of P restriction for 14-days (Royo et al., 2015) but not in soybean leaves after 24 h of P scarcity (Ramos-Artuso et al., 2019). However, in both plant species, NO increased and NR activity seemed to be involved in NO generation. If the amount of substrates for NO synthesis is affected under P deficiency, its availability may affect NO levels, but this factor is not the only one point of control for NO generation.
During P deficiency, the alteration of nutrient transport may be a non-specific result of changes in energy (low ATP), rates of water flow (hydraulic conductance), and membrane permeability; but other feedback control factors could affect NO3– uptake (Rufty et al., 1993). It is worth mentioning that in C. reinhardtii, NO inhibited the high-affinity uptake of NH4+ and NO3–/NO2–, as well as NR activity in a reversible form which may include post-translational regulation (Sanz-Luque et al., 2013). It has been extensively noted that NO affects NR activity, but the effect depends on several factors, such as the N source (e.g., NO3– concentration in the growth medium) and the level of NO or GSNO reached in each system (Buet et al., 2019, and references therein). Frungillo et al. (2014) has proposed that NO regulates NO3– assimilation pathways and also controls its bioavailability by modulating its own consumption in A. thaliana. The authors observed that high levels of NO and S-nitrosothiols induced a switch from high- to low-affinity NO3– transport reducing NO3– uptake. The authors also observed that genetically elevated levels of GSNO inhibited the activity of NR while reduced levels promoted its activity. Also the enzyme GSNO reductase (GSNOR1) was inhibited by S-nitrosation. As this enzyme catalyses the NADH-dependent reduction of GSNO (stable pool of NO) to oxidized glutathione and NH4+, its inhibition could prevent this GSNO degradation and amplify S-nitrosothiols signals (Frungillo et al., 2014). Further research would highlight whether this regulation of N assimilation could also operate in conditions of P scarcity, modulate NO levels and, in turn, P deficiency responses.
In P scarcity conditions, diverse factors such as changes in the availability of substrates (as NO2– or/and arginine) and modulation in the activity of some enzymes, including NR, XOR or NOS-like, can contribute to modify NO levels in planta. Despite NO origin, it may regulate N assimilation and, as a result, its own levels to participate in signaling events to afford P deficiency.
Plant’s Acclimation to P Restriction
Plants sense low P availability, leading to the activation of a complex signaling network, which runs morphological, metabolic and physiological modifications often with great variations among species or even cultivars. In general, main modifications allow plants to enhance soil exploration, and P availability in the soil solution, as well as to maintain P homeostasis at the whole-plant level.
In this section we will discuss the extent to which the presence of NO could affect key plant acclimation responses to P scarcity (Table 1).
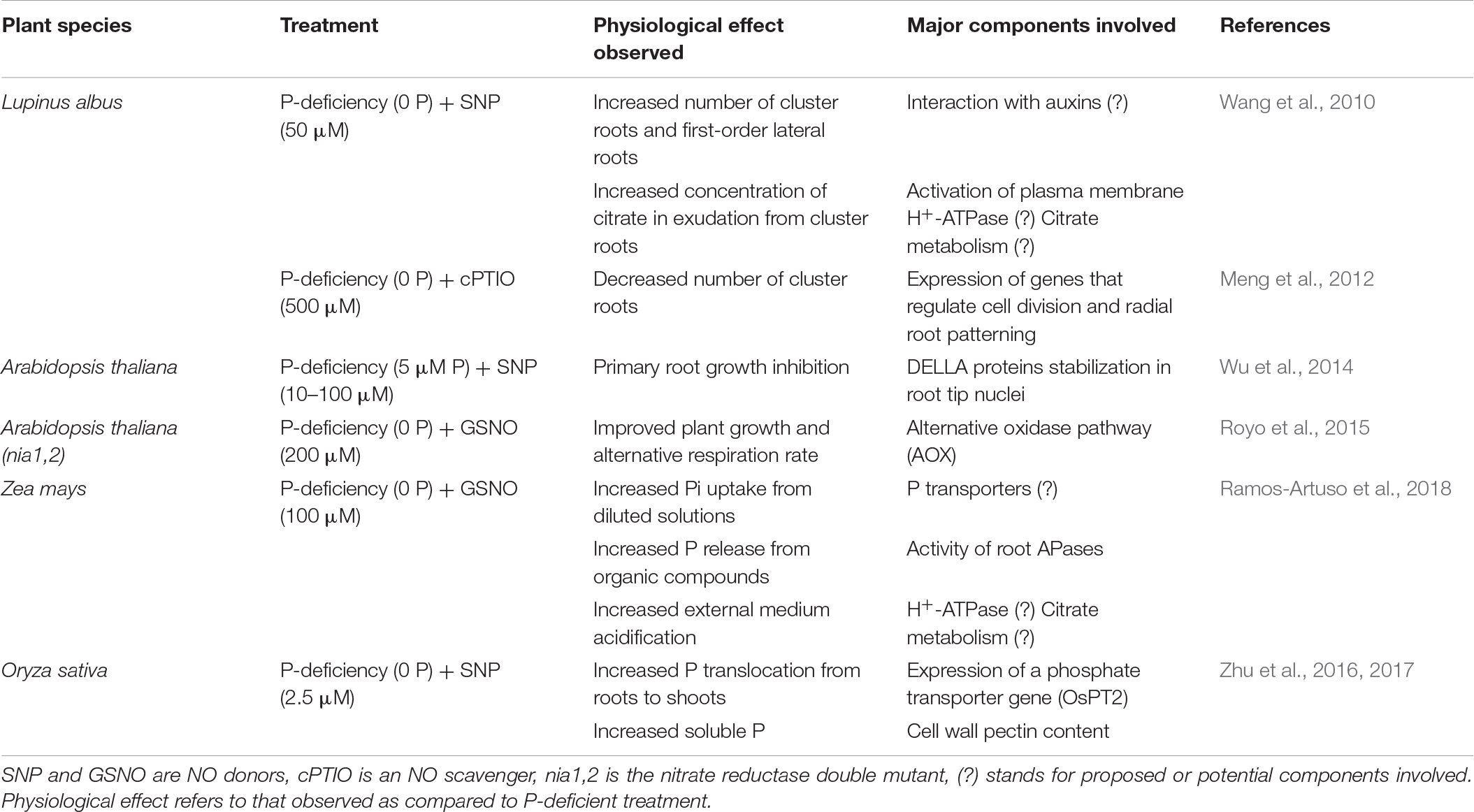
Table 1. Summary of NO effects on some physiological responses observed under P starvation and the potential components involved.
Root Morphology
Cluster Roots
As a response to P starvation, root architecture sustains modifications which shows a higher presence of roots in the topsoil horizon- usually the P-enriched fraction- (Peret et al., 2014; Del-Saz et al., 2018). These morphological responses probably contribute to an efficient acquisition of this nutrient (Aibara and Miwa, 2014).
One of the most remarkable adaptations for some species consists in the development of proteoid or cluster roots when they are growing in conditions of P scarcity. These roots expose an enhanced surface area and strongly acidify the rhizosphere. White lupin (Lupinus albus) is frequently used as a model plant to study the formation and function of cluster roots under P-deficient conditions (Cheng et al., 2011). Shoot P concentration, sucrose and hormones (cytokinins, ethylene, and auxins) have been identified as participants in cluster root development (Cheng et al., 2011; Meng et al., 2013; Müller et al., 2015). In addition, after 32 days of P restriction, cluster roots showed a massive change in gene transcription (Venuti et al., 2019). A relationship between NO and morphological changes in roots has been described in lupin plants under P restriction. Using the fluorescent probe DAF-FM DA, NO was found to increase in the pericycle, endodermis cells and rootlet primordia from plants exposed to P restriction during 20 days. These results, and the timing of NO accumulation, led the authors to suggest NO was involved in the initiation, development and emergence of rootlets in the P deficiency-induced cluster-root formation (Wang et al., 2010; Meng et al., 2012). In addition, treatments with NO donors SNP and GSNO induced changes in roots morphology (Wang et al., 2010; Meng et al., 2012).
Primary and Lateral Roots
In species with non-proteoid roots, the reshaping of the root system architecture under P-deficiency usually includes the development of lateral roots and the inhibition of primary root extension (Peret et al., 2014). A crosstalk between auxins (known as root growth regulators) and NO has been described for cucumber explants. In this system, NO accumulation was detected after exposure to indolacetic acid (IAA, an auxin), and treatment with NO donors SNP (10 μM) and S-nitroso-N-acetyl-penicillamine (SNAP 10 μM) induced the production of adventitious roots to the same extent as IAA (Pagnussat et al., 2002). In tomato and maize plants, treatment with auxins (1-naphthylacetic acid and IAA) or SNP (200 μM) induced similar lateral root formation (Correa-Aragunde et al., 2004; Zandonadi et al., 2010). Further research has led to suggest that NO is required for cell cycle progression and establishment of lateral root primordia in the pericycle as NO modulates cell cycle regulatory genes (Correa-Aragunde et al., 2006). However, the relationship between NO and auxins in the context of root development under P deprivation still needs addressing.
The effect of NO on primary roots was described for tomato growing under sufficient nutrient conditions, where treatment with SNP (200 μM) strongly reduced primary root length, whose effect was reversed in the presence of NO scavenger cPTIO (Correa-Aragunde et al., 2004). In Arabidopsis, the inhibitory effect of exogenous NO treatment (SNP 10–100 μM) was observed for both P-sufficient and P-deprived plants (Wu et al., 2014). Even though the use of cPTIO confirmed a direct action of NO, there remained doubt as only the Fe-containing NO donor (SNP) was used as exogenous NO source (Wu et al., 2014). In Arabidopsis, it was described that localized Fe accumulation in the root tip – rather than the reduction in P concentration- was responsible for the growth inhibition under P restriction (Ward et al., 2008). Growth arrest requires accumulation of transcription factor STOP1 in the nucleus. Under P-restriction, it has been proposed that Fe stimulates the accumulation of STOP1 in root nuclei which, in turn, activates the transcription of malate transporter gene ALMT1 (Godon et al., 2019). The use of other NO donors, and the measurement of NO levels in each system, will confirm the effect of NO on primary root morphology under P scarcity.
Other mechanisms involving GA and NO may play a part in primary root inhibition under P restriction. Knowing that P starvation decreases GA biosynthesis in Arabidopsis, and that the addition of exogenous GA acts as a positive regulator for primary root growth (Jiang et al., 2007; Wu et al., 2014), the interaction between NO and GA on primary root growth has been analyzed and showed that the treatments, both with NO donor SNP and with NO scavenger cPTIO, modulated the effect of P restriction on primary root growth. In assays using Arabidopsis mutants in DELLA proteins (negative regulators of GA signaling), it was found that exogenous NO (SNP 10 μM) stabilized these proteins in root tip nuclei. Thus NO inhibition of primary root growth involves, at least in part, the DELLA-degradation pathway (Wu et al., 2014).
Root Hairs
Enhanced root hair development is a typical adaptive plant response to P starvation, where increasing root surface area for nutrient uptake is mediated by ethylene (Zhang et al., 2003; Song et al., 2016). The description of NO participation as a regulator of root hair development has long been documented. Lettuce plants treated with SNP (10 μM) showed increased root hair number and elongation, and Arabidopsis root hair development was inhibited by cPTIO (0.5–1 mM). Moreover, NO has been proposed as a mediator of auxin action in root hair growth (Lombardo et al., 2006).
Other nutrient’s availability usually affects root hair development, as is the case of Mg deficiency (Liu et al., 2017). Arabidopsis plants exposed to Mg deficiency exhibited increased levels of NO as well as ethylene, and, interestingly, both species were reciprocally influenced and interactively regulated root hair morphogenesis (Liu et al., 2017). Recently, a scheme including auxins has been proposed for plants exposed to Mg deficiency. In Arabidopsis plants subjected to Mg deficiency, both ethylene and NO were required to regulate the rise of auxins in roots (Liu et al., 2018). A positive feedback loop involving auxins, ethylene and NO production under Mg deficiency was found. However, there remains a lack of information regarding the role of ethylene, NO and auxins interaction in root hair development during P scarcity. A similar interaction between NO and phytohormones occurring under P-restriction regulating root hair growth could be anticipated.
P Transport
The upregulation of high-affinity P transporters is a common phenomenon in response to P restriction in order to coordinate nutrient acquisition and distribution (Nussaume et al., 2011). As expected when compared with P-sufficient plants, there was a significant increase in the uptake of Pi from a diluted solution in maize plants exposed for 6 days to P-restriction, and the presence of an NO donor (GSNO 100 μM) further increased the potential capability of roots to incorporate P (Ramos-Artuso et al., 2018). Regulatory pathways integrated by microRNA and ubiquitin/26S proteasome degradation have been proposed for the selective modulation of P transport activity in response to P levels (Lin et al., 2014; Ye et al., 2018). The degradation of P transporters in P-sufficient conditions occurs through ubiquitin-mediated pathways which are reduced under P deficiency, activating Pi uptake as well as root-to-shoot translocation (Wang et al., 2017; Yue et al., 2017). In this regard, NO has been reported to be involved in ubiquitin-targeted protein degradation events in plants and animals (Arnaud et al., 2006; Kapadia et al., 2009). There is no knowledge directly related to how NO may affect P transport in plants; however, the activity of P transporters is regulated at both transcriptional and post-transcriptional levels (Nussaume et al., 2011), remarkably these processes are affected by NO under abiotic stress (Umbreen et al., 2018; Kolbert et al., 2019).
As mentioned before, P is easily redistributed, especially in plants under P-restriction. This includes the internal redistribution of P pools, the release of P from vacuoles, cell walls, and phospholipids in membranes, while at the whole plant level, P is reallocated to the young actively growing tissues (Veneklaas et al., 2012; Baker et al., 2015; Yang et al., 2017). P-translocation and the interconversion of different pools are related to the up-regulation of P transporters and enzymes (RNAses and phosphatases, among others), which release Pi from a broad range of P monoesters.
P starvation induced a specific group of cell-wall localized and intracellular APases, the PAPs (Shane et al., 2014; Ramos-Artuso et al., 2019), and in soybean the expression of a particular PAP (PAP21) increased in roots and old leaves, playing an important role in the utilization and recycling of P from intracellular reserves under P-starving conditions (Li et al., 2016). The same authors found that the overexpression of GmPAP21 enabled plants to achieve around 96% higher fresh weight under P restriction as compared with WT plants, pointing out the role of this enzyme in internal P use efficiency in plants. Results of assays on maize plants (Zea mays) exposed to an NO donor during P restriction suggested NO played a part in the enhanced activity of root APases (Ramos-Artuso et al., 2018). Short term P-deprivation experiments (48 h) showed that the increase in gene expression and in activity of PAP1 in roots from Medicago falcata were dependent on the presence of the ethylene hormone (Li et al., 2011). Also in Arabidopsis, ethylene positively regulates P starvation-induced gene expression and activity of APase (Lei et al., 2011). In addition, during senescence of petunia corolla, ethylene regulated P remobilization and the expression of a P transporter gene (PhPT1) (Chapin and Jones, 2009). An interplay between NO and ethylene occurs not only during plant growth and development, but also when the plant is exposed to abiotic stress (Kolbert et al., 2019), as it has been proposed for rice, where P-restriction led to a quick enhanced NO production followed by an ethylene peak (Zhu et al., 2017). As a result, pectin content increased allowing reutilization of P from the cell wall, and at the same time, the expression of a P transporter (OsPT2) was up-regulated to facilitate the translocation of P from root to shoot, improving growth under P restriction (Zhu et al., 2016, 2017). To our knowledge this is the only study over the effect of NO (applied as SNP), showing that NO increases the expression of a P transporter gene under P deficiency (Zhu et al., 2017).
Since P-restriction produces an increase in NO (Wang et al., 2010; Ramos-Artuso et al., 2019) and in ethylene levels (Borch et al., 1999; Zhu et al., 2016), both species could interact, and contribute to optimizing P availability and translocation inside plant, affecting P use efficiency.
Changes in the Rhizosphere
As a large proportion of P in soil is precipitated, chelated with metals, or becomes a constituent of organic compounds, the exudation of organic acids, protons and enzymes by roots contributes to improving plant’s P availability (Gaume et al., 2001; Shen et al., 2006; Pang et al., 2015). The “strategy” used varies greatly depending on plant species, genotypes and soil conditions (Hallama et al., 2019).
The induced secretion of ribonucleases, nucleases, phosphodiesterases, and APases is involved in P release from soil-localized organic substrates, such as nucleic acids and their degradation products, making P available for root uptake (Plaxton and Tran, 2011). Organic acids released from roots in the form of anions also contribute to increase P availability since they dissolve precipitates and chelate metal cations, and also block binding sites on soil particles. Citrate, malate and succinate from leaves can be transported via the phloem and directed to roots for exudation (Alexova et al., 2017). Citrate is the major organic acid released, and is one of the most effective species for solubilization of sparingly soluble P forms in soils (Igamberdiev and Eprintsev, 2016). NO was found to affect organic acid metabolism in citrus plants (Citrus grandis), where citrate content in roots increased after treatment with SNP (10 and 500 μM), due to an alteration in transport from leaves, whereas malate content increased (after treatment with 500 μM SNP), probably due to changes in the activity of the enzymes responsible for its synthesis and degradation (Yang et al., 2012). However, the dose of SNP used was toxic since plant growth was inhibited. As it has been previously discussed, lupin plants develop cluster roots under P-deficient conditions, characterized by their capacity to exude huge amounts of organic acids with citrate as the main component. Incubation of cluster roots from P-deficient lupin plants in the presence of an NO donor (SNP 50 μM, during 24 h) stimulated even more citrate exudation from the root, while the presence of cPTIO (an NO scavenger) had an inhibitory effect (Wang et al., 2010). Considering the side effects of SNP (León and Costa-Broseta, 2019), it would be interesting to test the effect of NO on organic acids production and exudation when using other NO donors in addition to carefully designed experimental approaches (such as the use of appropriate controls and scavengers).
Plant plasma membrane H+-ATPases transport protons out of the cell, controlling cell’s membrane potential and enabling the major transport processes in the plant, such as root nutrient uptake (Duby and Boutry, 2009). Enhanced H+ release to the rhizosphere occurs as a response to P-shortage and plays an important role in the acclimation to P-deficiency (Shen et al., 2006). Decrease in rhizosphere pH helps to dissolve P from Ca, Al or Fe phosphates and to increase P availability in calcareous soils (Hallama et al., 2019). It has been shown that the activity of plasma membrane H+-ATPase increases in P-restricted proteoid roots from lupin plants (Yan et al., 2002), and the enzyme has been described as an important component in responses to P restriction in soybean roots, where pharmacological and genetic approaches showed a parallel between enzyme activity and P uptake (Shen et al., 2006). Humic substances are naturally present in soils and, in addition to affecting root morphology, they stimulate the activity of H+-ATPase (Canellas et al., 2002). Interestingly, a link was found between NO and the humic-acid stimulation of H+-ATPase. In maize roots, plasma membrane H+-ATPase activity increased in the presence of NO donor SNP (200 μM), and the stimulatory effects of humic acids were inhibited by the addition of NO scavenger PTIO (Zandonadi et al., 2010). Moreover, data supporting a role for NO in the expression and activity of H+-ATPase were described in other systems under study. Calluses from reed (Phragmites communis Trin.) exhibited enhanced H+-ATPase activity 48 h after incubation with SNP (200 μM), and a reduced activity after treatment with PTIO (Zhao et al., 2004). None of the previous studies regarding the influence of NO or humic acids on the activity of H+-ATPase were performed under P restriction. Recently, maize plants exposed 6 days to P deprivation, being simultaneously in the presence of an NO donor (GSNO 100 μM), were found to exhibit higher capacity of external medium acidification as compared with P-deprived plants (Ramos-Artuso et al., 2018). It is worth considering that NO can be generated non-enzymatically in the apoplast, and its synthesis is favored when pH is low (Stöhr and Ullrich, 2002; Bethke et al., 2004). Thus, a mechanism in which NO induces external medium acidification, which, in turn, enhances NO synthesis under P-restricted conditions, may be suggested. Further experiments are necessary to unravel the role of NO over H+-ATPase activity in plants under P-restriction.
Apoplast acidification, through the activity of plasma membrane H+-ATPase, is also influenced by auxins, and it is involved not only in nutrient uptake, but also in cell growth (Rayle and Cleland, 1992). Auxins also induce accumulation of NO in roots, as has been evaluated in Arabidopsis, soybean, tomato, and cucumber (Pagnussat et al., 2002; Correa-Aragunde et al., 2004; Hu et al., 2005; Terrile et al., 2012). It is therefore possible to speculate an interplay between NO and auxins in the modulation of H+-ATPase activity under P scarcity.
Symbiotic Associations
The broad range of adaptive P stress responses includes the establishment of symbiotic association with AM fungi (Mensah et al., 2015), through which plants can enhance their capacity to acquire P from soils by increasing root uptake volume, dissolving insoluble P, mineralizing organic P, and avoiding P slow diffusion from the soil solution to root surface (Zhang et al., 2014). AM symbiosis has been associated with changes in the amount of plant hormones in roots (cytokinins, auxins, ethylene, jasmonic acid, abscisic acid and strigolactones), and NO has recently been described as playing a role at the early stages of that interaction (reviewed in Martínez-Medina et al., 2019b). Five days after inoculation of Medicago truncatula with Glomus mosseae, the expression of the NR gene was significantly upregulated in roots. It was suggested that a diffusible fungal factor was perceived by the host tissues, since the same result was obtained when roots had been physically separated from G. mosseae hyphae by a semipermeable barrier (Weidmann et al., 2004). In search for a link between NR transcript accumulation and NO levels, roots of M. truncatula were exposed to fungal exudates obtained from germinating Gigaspora margarita, simulating the presymbiotic phase of the interaction (Calcagno et al., 2012). During the first minutes after exposure, increases in NO levels were found in the epidermal and cortical tissues of roots evaluated using DAF-2DA and confocal microscopy. Moreover, NR transcript level increased after 10 min of treatment, and AM-dependent NO accumulation was suppressed when NR activity was inhibited with tungstate (Calcagno et al., 2012).
Increased levels of NO, associated with AM-plant interaction, were also confirmed in: roots of Trifolium repens L. inoculated with G. mosseae (Zhang et al., 2013); olive roots in association with Rhizophagus irregularis (Espinosa et al., 2014); P. trifoliata seedlings colonized by D. versiformis (Zou et al., 2017); and tomato plants at the onset of the AM symbiosis with R. irregularis (Martínez-Medina et al., 2019a). Based on these results, Martínez-Medina et al. (2019b) has proposed a model for the establishment of mycorrhizal symbiosis which, during the pre-symbiotic stages, diffusible fungal signals are perceived by the plant, triggering a burst of NO linked with the activation of the symbiotic regulatory pathway. This partially suppresses the host immune responses and prepares the plant for fungal colonization. In later stages, the level of NO in root cells is controlled by the action of phytoglobins (Martínez-Medina et al., 2019b). However, experimental data regarding NO participation during mutualistic interaction in P-restricted conditions are not available. NO’s role in some key P-deficiency responses, on the one hand, and in the interaction with AMF, on the other, opens up interesting queries regarding whether integrated responses involving NO and soil microorganisms may occur under P scarcity.
Concluding Remarks
To date there is little research on NO levels that have been conducted in plants exposed to P scarcity. NO increased after imposition of P-restriction treatments in lupin, Arabidopsis and rice roots, as well as in soybean leaves. NO seems to be implicated in acclimation responses to low P-availability, as it was described for citrate exudation, transcription and activity of a P transporter, P uptake from diluted solutions, external medium acidification and phosphatase activity, as well as modulation of some metabolic pathways. However, other functions of NO (for example, increasing H+-ATPase activity, role in symbiotic interaction, root hairs development, and lateral roots growth) remain to be studied in plants exposed to low P conditions.
Considering that a large amount of research has been developed using NO donors, caution should be taken regarding some observed side effects. In this regard, the use of other NO donors or scavengers in assays should be encouraged to strongly support some of the results. In addition, measurement of NO levels inside the plant as well as in different organs should be promoted to obtain a more integrated view.
Different mechanisms could be involved in NO synthesis during P scarcity but NR seems to have a critical role because of the close relationship between N metabolism and NO generation. Once NO concentration rises to a critical value, it may regulate its own specific level to exert a particular function. An interesting interaction of NO with hormones, such as ethylene, GA, and auxins during P deficiency may be proposed in some key acclimation responses. Moreover, future research in this field may confirm and show additional actors to this scenario. Overall, we encourage research in NO participation during P deficiency in order to find new tools to improve agricultural practices, avoiding fertilizers misuse and consequently, environmental damage.
Author Contributions
AG, FR-A, AB, and MS contributed equally to conceive and write the manuscript. ML prepared the figures and contributed to the writing. All the authors approved it for publication.
Funding
This work was supported by funds from the Agencia Nacional de Promoción Científica y Tecnológica (ANPCyT) (PICT 2017-2492), and Universidad Nacional de La Plata (A322).
Conflict of Interest
The authors declare that the research was conducted in the absence of any commercial or financial relationships that could be construed as a potential conflict of interest.
Acknowledgments
AB, AG, and MS are researchers from Consejo Nacional de Investigaciones Científicas y Técnicas (CONICET). FR-A and ML thank CONICET and ANPCyT for a fellowship. We are grateful to M. J. Doiny and C. Warwick-Foster for language proofreading.
Abbreviations
AM, arbuscular mycorrhizal; APases, acid phosphatases; ARC, amidoxime reducing component; cPTIO, 2-(4-carboxyphenyl)-4,4,5,5-tetramethylimidazoline-1-oxyl-3-oxide; DAF-FM DA, 4-amino-5-methylamino-2′,7′-difluorofluorescein diacetate; GA, gibberellic acid; GSNO, S-nitrosoglutathione; IAA, indolacetic acid; N, nitrogen; NiNOR, nitrite:nitric oxide reductase; NO, nitric oxide; NOFNiR, NO-forming nitrite reductase; NOS, nitric oxide synthase; NR, nitrate reductase; P, phosphate; PAPs, purple acid phosphatases; Pi, inorganic phosphate; PTIO, 2-phenyl-4,4,5,5-tetramethylimidazoline-1-oxyl-3-oxide; ROS, reactive oxygen species; SNAP, S-nitroso -N-acetyl-penicillamine; SNP, sodium nitroprusside; WT, wild type; XOR, xanthine oxidoreductase.
References
Aibara, I., and Miwa, K. (2014). Strategies for optimization of mineral nutrient transport in plants: multilevel regulation of nutrient-dependent dynamics of root architecture and transporter activity. Plant Cell Physiol. 55, 2027–2036. doi: 10.1093/pcp/pcu156
Alexova, R., Nelson, C. J., and Millar, A. H. (2017). Temporal development of the barley leaf metabolic response to P i limitation. Plant Cell Environ. 40, 645–657. doi: 10.1111/pce.12882
Arasimowicz-Jelonek, M., and Floryszak-Wieczorek, J. (2019). A physiological perspective on targets of nitration in NO-based signaling networks in plants. J. Exp. Bot. 70, 4379–4389. doi: 10.1093/jxb/erz300
Arnaud, N., Murgia, I., Boucherez, J., Briat, J. F., Cellier, F., and Gaymard, F. (2006). An iron-induced nitric oxide burst precedes ubiquitin-dependent protein degradation for Arabidopsis AtFer1 ferritin gene expression. J. Biol. Chem. 281, 23579–23588. doi: 10.1074/jbc.M602135200
Astier, J., Gross, I., and Durner, J. (2017). Nitric oxide production in plants: an update. J. Exp. Bot. 69, 3401–3411. doi: 10.1093/jxb/erx420
Astier, J., Mounier, A., Santolini, J., Jeandroz, S., and Wendehenne, D. (2019). The evolution of nitric oxide signalling diverges between the animal and the green lineages. J. Exp. Bot. 70, 4355–4364. doi: 10.1093/jas/sky123/4962501
Baker, A., Ceasar, S. A., Palmer, A. J., Paterson, J. B., Qi, W., Muench, S. P., et al. (2015). Replace, reuse, recycle: improving the sustainable use of phosphorus by plants. J. Exp. Bot. 66, 3523–3540. doi: 10.1093/jxb/erv210
Baxter, A., Mittler, R., and Suzuki, N. (2014). ROS as key players in plant stress signalling. J. Exp. Bot. 65, 1229–1240. doi: 10.1093/jxb/ert375
Begara-Morales, J. C., Chaki, M., Valderrama, R., Mata-Pérez, C., Padilla, M. N., and Barroso, J. B. (2019). The function of S-nitrosothiols during abiotic stress in plants. J. Exp. Bot. 70, 4429–4439. doi: 10.1093/jxb/erz197
Begara-Morales, J. C., and Loake, G. J. (2016). “Protein denitrosylation in plant biology, Protein,” in Gasotransmitters in Plants. Signaling and Communication in Plants, eds L. Lamattina and C. García-Mata (Cham: Springer).
Bethke, P. C., Badger, M. R., and Jones, R. L. (2004). Apoplastic synthesis of nitric oxide by plant tissues. Plant Cell 16, 332–341. doi: 10.1105/tpc.017822
Borch, K., Bouma, T. J., Lynch, J. P., and Brown, K. M. (1999). Ethylene: a regulator of root architectural responses to soil phosphorus availability. Plant Cell Environ. 22, 425–431. doi: 10.1046/j.1365-3040.1999.00405.x
Buet, A., Galatro, A., Ramos-Artuso, F., and Simontacchi, M. (2019). Nitric oxide and plant mineral nutrition: current knowledge. J. Exp. Bot. 70, 4461–4476. doi: 10.1093/jxb/erz129
Calcagno, C., Novero, M., Genre, A., Bonfante, P., and Lanfranco, L. (2012). The exudate from an arbuscular mycorrhizal fungus induces nitric oxide accumulation in Medicago truncatula roots. Mycorrhiza 22, 259–269. doi: 10.1007/s00572-011-0400-4
Canellas, L. P., Olivares, F. L., Okorokova-Façanha, A. L., and Façanha, A. R. (2002). Humic acids isolated from earthworm compost enhance root elongation, lateral root emergence, and plasma membrane H+-ATPase activity in maize roots. Plant Physiol. 130, 1951–1957. doi: 10.1104/pp.007088
Chamizo-Ampudia, A., Sanz-Luque, E., Llamas, A., Galván, A., and Fernández, E. (2017). Nitrate reductase regulates plant nitric oxide homeostasis. Trends Plant Sci. 22, 163–174. doi: 10.1016/j.tplants.2016.12.001
Chamizo-Ampudia, A., Sanz-Luque, E., Llamas, Á., Ocaña-Calahorro, F., Mariscal, V., Carreras, A., et al. (2016). A dual system formed by the ARC and NR molybdoenzymes mediates nitrite-dependent NO production in Chlamydomonas. Plant Cell Environ. 39, 2097–2107. doi: 10.1111/pce.12739
Chapin, L.-J., and Jones, M. L. (2009). Ethylene regulates phosphorus remobilization and expression of a phosphate transporter (PhPT1) during petunia corolla senescence. J. Exp. Bot. 60, 2179–2190. doi: 10.1093/jxb/erp092
Cheng, L., Bucciarelli, B., Shen, J., Allan, D., and Vance, C. P. (2011). Update on white lupin cluster root acclimation to phosphorus deficiency update on lupin cluster roots. Plant Physiol. 156, 1025–1032. doi: 10.1104/pp.111.175174
Chien, S. H., Sikora, F. J., Gilkes, R. J., and McLaughlin, M. J. (2012). Comparing of the difference and balance methods to calculate percent recovery of fertilizer phosphorus applied to soils: a critical discussion. Nutr. Cycl. Agroecosyst. 92, 1–8. doi: 10.1007/s10705-011-9467-8
Childers, D. L., Corman, J., Edwards, M., and Elser, J. J. (2011). Sustainability challenges of phosphorus and food: solutions from closing the human phosphorus cycle. Bioscience 61, 117–124. doi: 10.1525/bio.2011.61.2.6
Cordell, D., Drangert, J. O., and White, S. (2009). The story of phosphorus: global food security and food for thought. Glob. Environ. Change 19, 292–305. doi: 10.1016/j.gloenvcha.2008.10.009
Corpas, F. J., and Barroso, J. B. (2017). Nitric oxide synthase-like activity in higher plants. Nitric Oxide 68, 5–6. doi: 10.1016/j.niox.2016.10.009
Correa-Aragunde, N., Graziano, M., Chevalier, C., and Lamattina, L. (2006). Nitric oxide modulates the expression of cell cycle regulatory genes during lateral root formation in tomato. J. Exp. Bot. 57, 581–588. doi: 10.1093/jxb/erj045
Correa-Aragunde, N., Graziano, M., and Lamattina, L. (2004). Nitric oxide plays a central role in determining lateral root development in tomato. Planta 218, 900–905. doi: 10.1007/s00425-003-1172-7
Del-Saz, N. F., Romero-Munar, A., Cawthray, G. R., Palma, F., Aroca, R., Baraza, E., et al. (2018). Phosphorus concentration coordinates a respiratory bypass, synthesis and exudation of citrate, and the expression of high-affinity phosphorus transporters in Solanum lycopersicum. Plant Cell Environ. 41, 865–875. doi: 10.1111/pce.13155
Duby, G., and Boutry, M. (2009). The plant plasma membrane proton pump ATPase: a highly regulated P-type ATPase with multiple physiological roles. Pflügers Arch. Eur. J. Physiol. 457, 645–655. doi: 10.1007/s00424-008-0457-x
Elser, J., and Bennett, E. (2011). Phosphorus cycle: a broken biogeochemical cycle. Nature 478, 29–31. doi: 10.1038/478029a
Espinosa, F., Garrido, I., Ortega, A., Casimiro, I., and Álvarez-Tinaut, M. C. (2014). Redox activities and ROS, NO and phenylpropanoids production by axenically cultured intact olive seedling roots after interaction with a mycorrhizal or a pathogenic fungus. PLoS One 9:e100132. doi: 10.1371/journal.pone.0100132
Feelisch, M., and Martin, J. F. (1995). The early role of nitric oxide in evolution. Trends Ecol. Evol. 10, 496–499. doi: 10.1016/s0169-5347(00)89206-x
Foresi, N., Correa-Aragunde, N., Parisi, G., Caló, G., Salerno, G., and Lamattina, L. (2010). Characterization of a nitric oxide synthase from the plant kingdom: NO generation from the green alga Ostreococcus tauri is light irradiance and growth phase dependent. Plant Cell 22, 3816–3830. doi: 10.1105/tpc.109.07351
Fröhlich, A., and Durner, J. (2011). The hunt for plant nitric oxide synthase (NOS): Is one really needed? Plant Sci. 181, 401–404. doi: 10.1016/j.plantsci.2011.07.014
Frungillo, L., Skelly, M. J., Loake, G. J., Spoel, S. H., and Salgado, I. (2014). S-nitrosothiols regulate nitric oxide production and storage in plants through the nitrogen assimilation pathway. Nat. Commun. 5:5401. doi: 10.1038/ncomms6401
Gaume, A., Machler, F., De Leon, C., Narro, L., and Frossard, E. (2001). Low-P tolerance by maize (Zea mays L.) genotypes: significance of root growth, and organic acids and acid phosphatase root exudation. Plant Soil 228, 253–264.
Giehl, R. F. H., Meda, A. R., and von Wirén, N. (2009). Moving up, down, and everywhere: signaling of micronutrients in plants. Curr. Opin. Plant Biol. 12, 320–327. doi: 10.1016/j.pbi.2009.04.006
Godon, C., Mercier, C., Wang, X., David, P., Richaud, P., Nussaume, L., et al. (2019). Under phosphate starvation conditions, Fe and Al trigger accumulation of the transcription factor STOP1 in the nucleus of Arabidopsis root cells. Plant J. 937–949. doi: 10.1111/tpj.14374
Gupta, K. J., Fernie, A. R., Kaiser, W. M., and van Dongen, J. T. (2011). On the origins of nitric oxide. Trends Plant Sci. 6, 160–168.
Gupta, K. J., Hancock, J. T., Petrivalsky, M., Kolbert, Z., Lindermayr, C., Durner, J., et al. (2020). Recommendations on terminology and experimental best practice associated with plant nitric oxide research. New Phytol. 225, 1828–1834. doi: 10.1111/nph.16157
Hallama, M., Pekrun, C., Lambers, H., and Kandeler, E. (2019). Hidden miners – the roles of cover crops and soil microorganisms in phosphorus cycling through agroecosystems. Plant Soil 434, 7–45. doi: 10.1007/s11104-018-3810-7
Ham, B. K., Chen, J., Yan, Y., and Lucas, W. J. (2018). Insights into plant phosphate sensing and signaling. Curr. Opin. Biotechnol. 49, 1–9. doi: 10.1016/j.copbio.2017.07.005
Hu, X., Neill, S. J., Tang, Z., and Cai, W. (2005). Nitric oxide mediates gravitropic bending in soybean roots. Plant Physiol. 137, 663–670. doi: 10.1104/pp.104.054494
Igamberdiev, A. U., and Eprintsev, A. T. (2016). Organic acids: the pools of fixed carbon involved in redox regulation and energy balance in higher plants. Front. Plant Sci. 7:1042. doi: 10.3389/fpls.2016.01042
Jarvie, H. P., Sharpley, A. N., Flaten, D., Kleinman, P. J., Jenkins, A., and Simmons, T. (2015). The pivotal role of phosphorus in a resilient water–energy–food security nexus. J. Environ. Qual. 44, 1049–1062. doi: 10.2134/jeq2015.01.0030
Jeandroz, S., Wipf, D., Stuehr, D. J., Lamattina, L., Melkonian, M., Tian, Z., et al. (2016). Occurrence, structure, and evolution of nitric oxide synthase–like proteins in the plant kingdom. Sci. Signal. 9:re2. doi: 10.1126/scisignal.aad4403
Jiang, C., Gao, X., Liao, L., Harberd, N. P., and Fu, X. (2007). Phosphate starvation root architecture and anthocyanin accumulation responses are modulated by the gibberellin-DELLA signaling pathway in Arabidopsis. Plant Physiol. 145, 1460–1470. doi: 10.1104/pp.107.103788
Kapadia, M. R., Eng, J. W., Jiang, Q., Stoyanovsky, D. A., and Kibbe, M. R. (2009). Nitric oxide regulates the 26S proteasome in vascular smooth muscle cells. Nitric Oxide 20, 279–288. doi: 10.1016/j.niox.2009.02.005
Khan, M. S., Zaidi, A., and Ahmad, E. (2016). “Mechanism of phosphate solubilization and physiological functions of phosphate-solubilizing microorganisms,” in Phosphate Solubilizing Microorganisms, eds M. S. Khan, A. Zaidi, and J. Musarrat (Cham: Springer), 31–62. doi: 10.1007/978-3-319-08216-5_2
Kolbert, Z. (2016). Implication of nitric oxide (NO) in excess element-induced morphogenic responses of the root system. Plant Physiol. Biochem. 101, 149–161. doi: 10.1016/j.plaphy.2016.02.003
Kolbert, Z., Feigl, G., Freschi, L., and Poór, P. (2019). Gasotransmitters in action: nitric oxide-ethylene crosstalk during plant growth and abiotic stress responses. Antioxidants 8:167. doi: 10.3390/antiox8060167
Lamattina, L., García-Mata, C., Graziano, M., and Pagnussat, G. (2003). Nitric oxide: the versatility of an extensive signal molecule. Ann. Rev. Plant Biol. 54, 109–136. doi: 10.1146/annurev.arplant.54.031902.134752
Lei, M., Zhu, C., Liu, Y., Karthikeyan, A. S., Bressan, R. A., Raghothama, K. G., et al. (2011). Ethylene signalling is involved in regulation of phosphate starvation-induced gene expression and production of acid phosphatases and anthocyanin in Arabidopsis. New Phytol. 189, 1084–1095. doi: 10.1111/j.1469-8137.2010.03555.x
León, J., and Costa-Broseta, Á. (2019). Present knowledge and controversies, deficiencies and misconceptions on nitric oxide synthesis, sensing and signaling in plants. Plant Cell Environ. 43, 1–15. doi: 10.1111/pce.13617
Li, C., Li, C., Zhang, H., Liao, H., and Wang, X. (2016). The purple acid phosphatase GmPAP21 enhances internal phosphorus utilization and possibly plays a role in symbiosis with rhizobia in soybean. Physiol. Plant. 159, 215–227. doi: 10.1111/ppl.12524
Li, Y. S., Gao, Y., Tian, Q. Y., Shi, F. L., Li, L. H., and Zhang, W. H. (2011). Stimulation of root acid phosphatase by phosphorus deficiency is regulated by ethylene in Medicago falcata. Environ. Exp. Bot. 71, 114–120. doi: 10.1016/j.envexpbot.2010.11.007
Lin, W. Y., Huang, T. K., Leong, S. J., and Chiou, T. J. (2014). Long-distance call from phosphate: systemic regulation of phosphate starvation responses. J. Exp. Bot. 65, 1817–1827. doi: 10.1093/jxb/ert431
Liu, M., Liu, X. X., He, X. L., Liu, L. J., Wu, H., Tang, C. X., et al. (2017). Ethylene and nitric oxide interact to regulate the magnesium deficiency-induced root hair development in Arabidopsis. New Phytol. 213, 1242–1256. doi: 10.1111/nph.14259
Liu, M., Zhang, H., Fang, X., Zhang, Y., and Jin, C. (2018). Auxin acts downstream of ethylene and nitric oxide to regulate magnesium deficiency-induced root hair development in Arabidopsis thaliana. Plant Cell Physiol. 59, 1452–1465. doi: 10.1093/pcp/pcy078
Lombardo, M. C., Graziano, M., Polacco, J. C., and Lamattina, L. (2006). Nitric oxide functions as a positive regulator of root hair development. Plant Signal. Behav. 1, 28–33. doi: 10.4161/psb.1.1.2398
Macintosh, K. A., Doody, D. G., Withers, P. J., McDowell, R. W., Smith, D. R., Johnson, L. T., et al. (2019). Transforming soil phosphorus fertility management strategies to support the delivery of multiple ecosystem services from agricultural systems. Sci. Total Environ. 649, 90–98. doi: 10.1016/j.scitotenv.2018.08.272
Marschner, H. (2011). “Plant-soil relationship,” in Marschner’s Mineral Nutrition of Higher Plants, ed. P. Marschner (Cambridge, MA: Academic Press), 315–371.
Martínez-Medina, A., Pescador, L., Fernández, I., Rodríguez-Serrano, M., García, J. M., Romero-Puertas, M. C., et al. (2019a). Nitric oxide and phytoglobin PHYTOGB1 are regulatory elements in the Solanum lycopersicum–Rhizophagus irregularis mycorrhizal symbiosis. New Phytol. 223, 1560–1574. doi: 10.1111/nph.15898
Martínez-Medina, A., Pescador, L., Terrón-Camero, L. C., Pozo, M. J., and Romero-Puertas, M. C. (2019b). Nitric oxide in plant–fungal interactions. J. Exp. Bot. 70, 4489–4503. doi: 10.1093/jxb/erz289
McLaughlin, M. J., McBeath, T. M., Smernik, R., Stacey, S. P., Ajiboye, B., and Guppy, C. (2011). The chemical nature of P accumulation in agricultural soils—implications for fertiliser management and design: an Australian perspective. Plant Soil 349, 69–87. doi: 10.1007/s11104-011-0907-7
Meng, Z. B., Chen, L. Q., Suo, D., Li, G. X., Tang, C. X., and Zheng, S. J. (2012). Nitric oxide is the shared signalling molecule in phosphorus- and iron-deficiency-induced formation of cluster roots in white lupin (Lupinus albus). Ann. Bot. 109, 1055–1064. doi: 10.1093/aob/mcs024
Meng, Z. B., Di You, X., Suo, D., Chen, Y. L., Tang, C., Yang, J. L., et al. (2013). Root-derived auxin contributes to the phosphorus-deficiency-induced cluster-root formation in white lupin (Lupinus albus). Physiol. Plant. 148, 481–489. doi: 10.1111/j.1399-3054.2012.01715.x
Mensah, J. A., Koch, A. M., Antunes, P. M., Kiers, E. T., Hart, M., and Bücking, H. (2015). High functional diversity within species of arbuscular mycorrhizal fungi is associated with differences in phosphate and nitrogen uptake and fungal phosphate metabolism. Mycorrhiza 25, 533–546. doi: 10.1007/s00572-015-0631-x
Molina-Favero, C., Creus, C. M., Lanteri, M. L., Correa-Aragunde, N., Lombardo, M. C., Barassi, C. A., et al. (2007). Nitric oxide and plant growth promoting rhizobacteria: common features influencing root growth and development. Adv. Bot. Res. 46, 1–33. doi: 10.1016/s0065-2296(07)46001-3
Moreau, M., Lindermayr, C., Durner, J., and Klessig, D. F. (2010). NO synthesis and signaling in plants – where do we stand? Physiol. Plant. 138, 372–383. doi: 10.1111/j.1399-3054.2009.01308.x
Müller, J., Gödde, V., Niehaus, K., and Zörb, C. (2015). Metabolic adaptations of white lupin roots and shoots under phosphorus deficiency. Front. Plant Sci. 6:1014. doi: 10.3389/fpls.2015.01014
Mur, L. A. J., Mandon, J., Persijn, S., Cristescu, S. M., Moshkov, I. E., Novikova, G. V., et al. (2013). Nitric oxide in plants: an assessment of the current state of knowledge. AoB Plants 5:ls052. doi: 10.1093/aobpla/pls052
Nussaume, L., Kanno, S., Javot, H., Marin, E., Pochon, N., Ayadi, A., et al. (2011). Phosphate import in plants: focus on the PHT1 transporters. Front. Plant Sci. 2:83. doi: 10.3389/fpls.2011.00083
Oliveira, H. C., Freschi, L., and Sodek, L. (2013). Nitrogen metabolism and translocation in soybean plants subjected to root oxygen deficiency. Plant Physiol. Biochem. 66, 141–149. doi: 10.1016/j.plaphy.2013.02.015
Pagnussat, G. C., Simontacchi, M., Puntarulo, S., and Lamattina, L. (2002). Nitric oxide is required for root organogenesis. Plant Physiol. 129, 954–956. doi: 10.1104/pp.004036
Pang, J., Yang, J., and Lambers, H. (2015). Physiological and morphological adaptations of herbaceous perennial legumes allow differential access to sources of varyingly soluble phosphate. Physiol. Plant. 154, 511–525. doi: 10.1111/ppl.12297
Pang, J., Zhao, H., Bansal, R., Bohuon, E., Lambers, H., Ryan, M. H., et al. (2018). Leaf transpiration plays a role in phosphorus acquisition among a large set of chickpea genotypes. Plant Cell Environ. 41, 2069–2079. doi: 10.1111/pce.13139
Peret, B., Desnos, T., Jost, R., Kanno, S., Berkowitz, O., and Nussaume, L. (2014). Root architecture responses: in search of phosphate. Plant Physiol. 166, 1713–1723. doi: 10.1104/pp.114.244541
Pierre, W. H., and Parker, F. W. (1927). Soil phosphorus studies: II. The concentration of organic and inorganic phosphorus in the soil solution and soil extracts and the availability of the organic phosphorus to plants. Soil Sci. 24, 119–128. doi: 10.1097/00010694-192708000-00005
Plaxton, W. C., and Tran, H. T. (2011). Metabolic adaptations of phosphate-starved plants. Plant Physiol. 156, 1006–1015. doi: 10.1104/pp.111.175281
Pratt, J., Boisson, A. M., Gout, E., Bligny, R., Douce, R., and Aubert, S. (2009). Phosphate (Pi) starvation effect on the cytosolic Pi concentration and Pi exchanges across the tonoplast in plant cells: an in vivo 31P-nuclear magnetic resonance study using methylphosphonate as a Pi analog. Plant Physiol. 151, 1646–1657. doi: 10.1104/pp.109.144626
Rabe, E., and Lovatt, C. J. (1986). Increased arginine biosynthesis during phosphorus deficiency. Plant Physiol. 81, 774–779. doi: 10.1104/pp.81.3.774
Radi, R. (2004). Nitric oxide, oxidants, and protein tyrosine nitration. Proc. Natl. Acad. Sci. U.S.A. 101, 4003–4008. doi: 10.1073/pnas.0307446101
Raghothama, K. G., and Karthikeyan, A. S. (2005). Phosphate acquisition. Plant Soil 274:37. doi: 10.1007/1-4020-4099-7_2
Ramos-Artuso, F., Galatro, A., Buet, A., Santa-María, G. E., and Simontacchi, M. (2018). Key acclimation responses to phosphorus deficiency in maize plants are influenced by exogenous nitric oxide. J. Plant Physiol. 222, 51–58. doi: 10.1016/j.jplph.2018.01.001
Ramos-Artuso, F., Galatro, A., Lima, A., Batthyány, C., and Simontacchi, M. (2019). Early events following phosphorus restriction involve changes in proteome and affects nitric oxide metabolism in soybean leaves. Environ. Exp. Bot. 161, 203–217. doi: 10.1016/j.envexpbot.2019.01.002
Rayle, D. L., and Cleland, R. E. (1992). The acid growth theory of auxin-induced cell elongation is alive and well. Plant Physiol. 99, 1271–1274. doi: 10.1104/pp.99.4.1271
Royo, B., Moran, J. F., Ratcliffe, R. G., and Gupta, K. J. (2015). Nitric oxide induces the alternative oxidase pathway in Arabidopsis seedlings deprived of inorganic phosphate. J. Exp. Bot. 66, 6273–6280. doi: 10.1093/jxb/erv338
Ruffel, S. (2018). Nutrient-related long-distance signals: common players and possible cross-talk. Plant Cell Physiol. 59, 1723–1732. doi: 10.1093/pcp/pcy152
Rufty, T. W. Jr., Israel, D. W., Volk, R. J., Qiu, J., and Sa, T. (1993). Phosphate regulation of nitrate assimilation in soybean. J. Exp. Bot. 44, 879–891. doi: 10.1007/s00425-014-2165-4
Sakuraba, Y., Kanno, S., Mabuchi, A., Monda, K., Iba, K., and Yanagisawa, S. (2018). A phytochrome-B-mediated regulatory mechanism of phosphorus acquisition. Nat. Plants 4, 1089–1101. doi: 10.1038/s41477-018-0294-7
Santolini, J., André, F., Jeandroz, S., and Wendehenne, D. (2017). Nitric oxide synthase in plants: Where do we stand? Nitric Oxide 63, 30–38. doi: 10.1016/j.niox.2016.09.005
Sanz-Luque, E., Ocaña-Calahorro, F., de Montaigu, A., Chamizo-Ampudia, A., Llamas, Á., Galván, A., et al. (2015). THB 1, a truncated hemoglobin, modulates nitric oxide levels and nitrate reductase activity. Plant J. 81, 467–479. doi: 10.1111/tpj.12744
Sanz-Luque, E., Ocaña-Calahorro, F., Llamas, A., Galván, A., and Fernández, E. (2013). Nitric oxide controls nitrate and ammonium assimilation in Chlamydomonas reinhardtii. J. Exp. Bot. 64, 3373–3383. doi: 10.1093/jxb/ert175
Schoumans, O. F., Chardon, W. J., Bechmann, M. E., Gascuel-Odoux, C., Hofman, G., Kronvang, B., et al. (2014). Mitigation options to reduce phosphorus losses from the agricultural sector and improve surface water quality: a review. Sci. Total Environ. 468, 1255–1266. doi: 10.1016/j.scitotenv.2013.08.061
Schröder, J. J., Smit, A. L., Cordell, D., and Rosemarin, A. (2011). Improved phosphorus use efficiency in agriculture: a key requirement for its sustainable use. Chemosphere 84, 822–831. doi: 10.1016/j.chemosphere.2011.01.065
Shane, M. W., Stigter, K., Fedosejevs, E. T., and Plaxton, W. C. (2014). Senescence-inducible cell wall and intracellular purple acid phosphatases: implications for phosphorus remobilization in Hakea prostrata (Proteaceae) and Arabidopsis thaliana (Brassicaceae). J. Exp. Bot. 65, 6097–6106. doi: 10.1093/jxb/eru348
Shen, H., Chen, J., Wang, Z., Yang, C., Sasaki, T., Yamamoto, Y., et al. (2006). Root plasma membrane H+-ATPase is involved in the adaptation of soybean to phosphorus starvation. J. Exp. Bot. 57, 1353–1362. doi: 10.1093/jxb/erj111
Shen, J., Yuan, L., Zhang, J., Li, H., Bai, Z., Chen, X., et al. (2011). Phosphorus dynamics: from soil to plant. Plant Physiol. 156, 997–1005. doi: 10.1104/pp.111.175232
Singh, S., Kumar, V., Kapoor, D., Kumar, S., and Singh, S. (2019). Revealing on hydrogen sulfide and nitric oxide signals co-ordination for plant growth under stress conditions. Physiol. Plant. 168, 301–317. doi: 10.1111/ppl.13002
Skiba, U., Smith, K. A., and Fowler, D. (1993). Nitrification and denitrification as sources of nitric oxide and nitrous oxide in a sandy loam soil. Soil Biol. Biochem. 25, 1527–1536. doi: 10.1073/pnas.1219993110
Song, L., Yu, H., Dong, J., Che, X., Jiao, Y., and Liu, D. (2016). The molecular mechanism of ethylene-mediated root hair development induced by phosphate starvation. PLoS Genet. 12:e1006194. doi: 10.1371/journal.pgen.1006194
Stöhr, C., and Ullrich, W. R. (2002). Generation and possible roles of NO in plant roots and their apoplastic space. J. Exp. Bot. 53, 2293–2303. doi: 10.1093/jxb/erf110
Stuehr, D. J. (1999). Mammalian nitric oxide synthases. Biochim. Biophys. Acta 1411, 217–230. doi: 10.1016/S0005-2728(99)00016-X
Terrile, M. C., París, R., Calderón-Villalobos, L. I. A., Iglesias, M. J., Lamattina, L., Estelle, M., et al. (2012). Nitric oxide influences auxin signaling through S-nitrosylation of the Arabidopsis TRANSPORT INHIBITOR RESPONSE 1 auxin receptor. Plant J. 70, 492–500. doi: 10.1111/j.1365-313X.2011.04885.x
Ueda, Y., and Yanagisawa, S. (2019). Perception, transduction and integration of nitrogen and phosphorus nutritional signals in the transcriptional regulatory network in plants. J. Exp. Bot. 70, 3709–3717. doi: 10.1093/jxb/erz148
Umbreen, S., Lubega, J., Cui, B., Pan, Q., Jiang, J., and Loake, G. J. (2018). Specificity in nitric oxide signalling. J. Exp. Bot. 69, 3439–3448. doi: 10.1093/jxb/ery184
Veneklaas, E. J., Lambers, H., Bragg, J., Finnegan, P. M., Lovelock, C. E., Plaxton, W. C., et al. (2012). Opportunities for improving phosphorus-use efficiency in crop plants. New Phytol. 195, 306–320. doi: 10.1111/j.1469-8137.2012.04190.x
Venuti, S., Zanin, L., Marroni, F., Franco, A., Morgante, M., Pinton, R., et al. (2019). Physiological and transcriptomic data highlight common features between iron and phosphorus acquisition mechanisms in white lupin roots. Plant Sci. 285, 110–121. doi: 10.1016/j.plantsci.2019.04.026
Wang, B. L., Tang, X. Y., Cheng, L. Y., Zhang, A. Z., Zhang, W. H., Zhang, F. S., et al. (2010). Nitric oxide is involved in phosphorus deficiency-induced cluster-root development and citrate exudation in white lupin. New Phytol. 187, 1112–1123. doi: 10.1111/j.1469-8137.2010.03323.x
Wang, D., Lv, S., Jiang, P., and Li, Y. (2017). Roles, regulation, and agricultural application of plant phosphate transporters. Front. Plant Sci. 8:817. doi: 10.3389/fpls.2017.00817
Wang, F., Deng, M., Xu, J., Zhu, X., and Mao, C. (2018). Molecular mechanisms of phosphate transport and signaling in higher plants. Semin. Cell Dev. Biol. 74, 114–122. doi: 10.1016/j.semcdb.2017.06.013
Wang, Y. L., Almvik, M., Clarke, N., Eich-Greatorex, S., Øgaard, A. F., Krogstad, T., et al. (2015). Contrasting responses of root morphology and root-exuded organic acids to low phosphorus availability in three important food crops with divergent root traits. AoB Plants 7:lv097. doi: 10.1093/aobpla/plv097
Ward, J. T., Lahner, B., Yakubova, E., Salt, D. E., and Raghothama, K. G. (2008). The effect of iron on the primary root elongation of Arabidopsis during phosphate deficiency. Plant Physiol. 147, 1181–1191. doi: 10.1104/pp.108.118562
Weidmann, S., Sanchez, L., Descombin, J., Chatagnier, O., Gianinazzi, S., and Gianinazzi-Pearson, V. (2004). Fungal elicitation of signal transduction-related plant genes precedes mycorrhiza establishment and requires the dmi3 gene in Medicago truncatula. Mol. Plant Microbe Interact. 17, 1385–1393. doi: 10.1094/MPMI.2004.17.12.1385
Wu, A. P., Gong, L., Chen, X., and Wang, J. X. (2014). Interactions between nitric oxide, gibberellic acid, and phosphorus regulate primary root growth in Arabidopsis. Biol. Plant. 58, 335–340. doi: 10.1007/s10535-014-0408-7
Xu, J., Yin, H., Li, Y., and Liu, X. (2010). Nitric oxide is associated with long-term zinc tolerance in Solanum nigrum. Plant Physiol. 154, 1319–1334. doi: 10.1104/pp.110.162982
Yan, F., Zhu, Y., Mü, C., Zo, C., and Schubert, S. (2002). Adaptation of H+ -pumping and plasma membrane H+ ATPase activity in proteoid roots of white lupin under phosphate deficiency. Plant Physiol. 1, 50–63. doi: 10.1104/pp.010869.50
Yang, L. T., Chen, L. S., Peng, H. Y., Guo, P., Wang, P., and Ma, C. L. (2012). Organic acid metabolism in Citrus grandis leaves and roots is differently affected by nitric oxide and aluminum interactions. Sci. Hortic. 133, 40–46. doi: 10.1016/j.scienta.2011.10.011
Yang, S. Y., Huang, T. K., Kuo, H. F., and Chiou, T. J. (2017). Role of vacuoles in phosphorus storage and remobilization. J. Exp. Bot 68, 3045–3055. doi: 10.1093/jxb/erw481
Ye, Q., Wang, H., Su, T., Wu, W.-H., and Chen, Y.-F. (2018). The Ubiquitin E3 Ligase PRU1 regulates WRKY6 degradation to modulate phosphate homeostasis in response to low-Pi stress in Arabidopsis. Plant Cell 30, 1062–1076. doi: 10.1105/tpc.17.00845
Yue, W., Ying, Y., Wang, C., Zhao, Y., Dong, C., Whelan, J., et al. (2017). OsNLA1, a RING-type ubiquitin ligase, maintains phosphate homeostasis in Oryza sativa via degradation of phosphate transporters. Plant J. 90, 1040–1051. doi: 10.1111/tpj.13516
Zandonadi, D. B., Santos, M. P., Dobbss, L. B., Olivares, F. L., Canellas, L. P., Binzel, M. L., et al. (2010). Nitric oxide mediates humic acids-induced root development and plasma membrane H+-ATPase activation. Planta 231, 1025–1036. doi: 10.1007/s00425-010-1106-0
Zhang, R. Q., Zhu, H. H., Zhao, H. Q., and Yao, Q. (2013). Arbuscular mycorrhizal fungal inoculation increases phenolic synthesis in clover roots via hydrogen peroxide, salicylic acid and nitric oxide signaling pathways. J. Plant Physiol. 170, 74–79. doi: 10.1016/j.jplph.2012.08.022
Zhang, Y., Lynch, J. P., and Brown, K. M. (2003). Ethylene and phosphorus availability have interacting yet distinct effects on root hair development. J. Exp. Bot. 54, 2351–2361. doi: 10.1093/jxb/erg250
Zhang, Z., Liao, H., and Lucas, W. J. (2014). Molecular mechanisms underlying phosphate sensing, signaling, and adaptation in plants. J. Integr. Plant Biol. 56, 192–220. doi: 10.1111/jipb.12163
Zhao, L., Zhang, F., Guo, J., Yang, Y., Li, B., and Zhang, L. (2004). Nitric oxide functions as a signal in salt resistance in the calluses from two ecotypes of reed. Plant Physiol. 134, 849–857. doi: 10.1104/pp.103.030023
Zhu, C. Q., Zhu, X. F., Hu, A. Y., Wang, C., Wang, B., Dong, X. Y., et al. (2016). Differential effects of nitrogen forms on cell wall phosphorus remobilization in rice (Oryza sativa) are mediated by nitric oxide, pectin content and the expression of the phosphate transporter OsPT2. Plant Physiol. 171, 1407–1417. doi: 10.1104/pp.16.00176
Zhu, X. F., Dong, X. Y., Wu, Q., and Shen, R. F. (2019). Ammonium regulates Fe deficiency responses by enhancing nitric oxide signaling in Arabidopsis thaliana. Planta 250, 1089–1102. doi: 10.1007/s00425-019-03202-6
Zhu, X. F., Zhu, C. Q., Wang, C., Dong, X. Y., and Shen, R. F. (2017). Nitric oxide acts upstream of ethylene in cell wall phosphorus reutilization in phosphorus-deficient rice. J. Exp. Bot. 68, 753–760. doi: 10.1093/jxb/erw480
Keywords: abiotic stress, acid phosphatases, plant mineral nutrition, phosphate, reactive nitrogen species
Citation: Galatro A, Ramos-Artuso F, Luquet M, Buet A and Simontacchi M (2020) An Update on Nitric Oxide Production and Role Under Phosphorus Scarcity in Plants. Front. Plant Sci. 11:413. doi: 10.3389/fpls.2020.00413
Received: 03 December 2019; Accepted: 23 March 2020;
Published: 15 April 2020.
Edited by:
Manuel Nieves-Cordones, Spanish National Research Council, SpainReviewed by:
John Hancock, University of the West of England, United KingdomSylvia Morais De Sousa, Embrapa Maize and Sorghum, Brazil
Copyright © 2020 Galatro, Ramos-Artuso, Luquet, Buet and Simontacchi. This is an open-access article distributed under the terms of the Creative Commons Attribution License (CC BY). The use, distribution or reproduction in other forums is permitted, provided the original author(s) and the copyright owner(s) are credited and that the original publication in this journal is cited, in accordance with accepted academic practice. No use, distribution or reproduction is permitted which does not comply with these terms.
*Correspondence: Marcela Simontacchi, marcelasimontacchi@agro.unlp.edu.ar