- Laboratory of Molecular and Cluster Dynamics, Department of Ion and Cluster Chemistry, J. Heyrovský Institute of Physical Chemistry, Academy of Sciences of the Czech Republic, Prague, Czech Republic
This review summarizes some recent experiments with ice nanoparticles (large water clusters) in molecular beams and outlines their atmospheric relevance: (1) Investigation of mixed water–nitric acid particles by means of the electron ionization and sodium doping combined with photoionization revealed the prominent role of HNO3 molecule as the condensation nuclei. (2) The uptake of atmospheric molecules by water ice nanoparticles has been studied, and the pickup cross sections for some molecules exceed significantly the geometrical sizes of the ice nanoparticles. (3) Photodissociation of hydrogen halides on water ice particles has been shown to proceed via excitation of acidically dissociated ion pair and subsequent biradical generation and H3O dissociation. The photodissociation of CF2Cl2 molecules in clusters is also mentioned. Possible atmospheric consequences of all these results are briefly discussed.
1. Introduction
The fact that small atmospheric ice particles and aerosols are important players in atmospheric chemistry has been recognized and outlined in textbooks, e.g., Finlayson-Pitts and Pitts (2000), and numerous review articles, e.g., Peter (1997); Ravishankara (1997); Finlayson-Pitts (2009); and Vaida (2011). Perhaps the most pronounced example is the stratospheric ozone depletion process, where the ice particles in polar stratospheric clouds (PSC) play a key role (Peter, 1997; Solomon, 1999; Prenni and Tolbert, 2001; Lu, 2010). The investigations of small nanometer-size particles directly in the atmosphere are difficult thus such studies are yet scarce, and large ambiguities exist in this size region. However, these small particles have large surface to volume ratio and offer a substantial playground for the surface assisted chemistry and photochemistry in the atmosphere. Recently Kulmala et al. (2013) stressed the importance of the particles from this size range as the initial step in the atmospheric aerosol formation.
To investigate the relevant processes in laboratory, a vast number of studies on bulk ice surfaces are carried out including uptake and reactivity of atmospheric gasses on ice (Hanson, 1995; Oppliger et al., 1997; Huthwelker et al., 2006; Marcotte et al., 2013), and their photochemistry (Klán and Holoubek, 2002; Yabushita et al., 2002). On the other hand, the cluster physicists and chemists have developed a plethora of approaches for studying clusters in laboratory experiments in molecular beams which enable controlled generation of nanometer-size particles with composition corresponding to the atmospheric species (Campargue, 2001; Buch and Devlin, 2003). The details of ice nanoparticles generation in supersonic expansions has been recently investigated by Kim et al. (2004), Manka et al. (2012), and Li et al. (2013). The individual particles can be investigated under controlled conditions in vacuum by various means: e.g., ionization (electron, photon) and mass spectrometry (MacTaylor and Castleman, 2000; Lengyel et al., 2012b); infrared (IR) spectroscopy (Yacovitch et al., 2011; 2012; Preston et al., 2012; Fujii and Mizuse, 2013) or ultraviolet (UV) photodissociation experiments (Kreher et al. 1999; Li and Huber, 2001; Poterya et al. 2007, 2008a; 2011; Ončák et al., 2008, 2011); particle (electron, photon, neutron) scattering (Heath et al., 2002; Kim et al., 2004; Manka et al., 2012); special methods such as sodium doping and subsequent spectroscopies (Bobbert et al., 2002; Yoder et al., 2011; Pradzynski et al., 2012). Such experiments provide unprecedented molecular-level insight into the small particle generation, their (photo)chemistry and (photo)physics and detailed dynamics of the processes on/in these particles. This in turn offers valuable data for understanding and modeling of atmospheric chemistry. Some of the methods of aerosol particle spectroscopy have been reviewed in recent book (Signorell and Reid, 2011).
Here we review some recent results of the molecular beam experiments with the ice nanoparticles performed mostly in our laboratory. Our versatile experiment allows to look at different aspects of the ice nanoparticle chemistry and physics: e.g., nucleation, pickup processes, and photochemistry. The purpose of this review is to summarize the different viewpoints outlined in the individually published articles to provide a new perspective for such experiments with ice nanoparticles, and also to discuss some possible direct consequences for atmospheric chemistry and physics. Wherever similar experiments exist in the literature, we attempt to review them briefly and outline our work in their context.
We will start with nucleation of nitric acid ice particles as revealed by special Na-doping method. Then we report about ice particle growth by pickup of molecules. Finally, we discuss UV photodissociation of atmospheric molecules on ice nanoparticles which is the major focus of our studies.
2. Materials and Methods
The majority of experiments reviewed in this article was performed in our laboratory on CLUster Beam apparatus (CLUB). A detailed description of CLUB setup can be found in Fárník (2011) and some recent extensions of this apparatus are outlined below. It is schematically depicted in Figure 1: it represents a unique and versatile setup for variety of experiments with clusters. The molecular beam is produced by a continuous supersonic gas expansion through a nozzle into vacuum. The mean cluster sizes can be controlled by the expansion conditions: pressure, temperature and nozzle geometry. The clusters can be doped with foreign molecules either in coexpansion or by passing them through a pickup cell filled with the molecular gas.
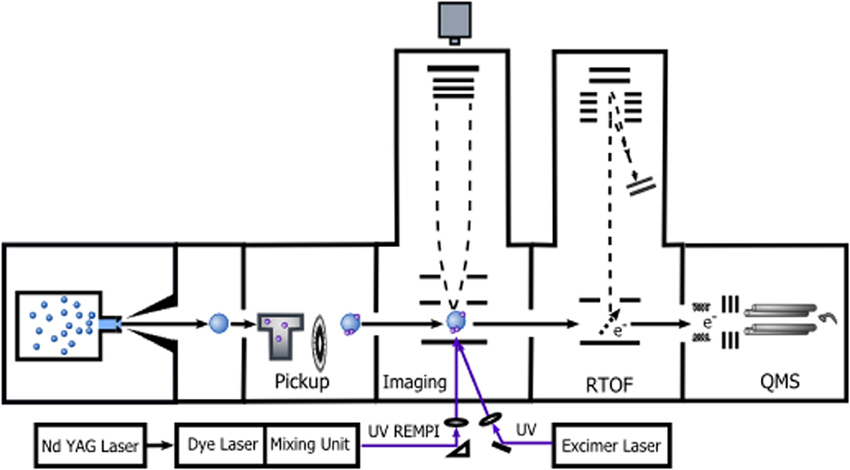
Figure 1. Schematics of the experimental apparatus CLUB and the experiments outlined in this article.
After passing through several differentially pumped high vacuum chambers the cluster beam enters the chamber where the photodissociation experiments are performed. The molecules in clusters are excited with nanosecond UV laser pulses. The desired photofragments are then selectively photoionized by resonance enhanced multiphoton ionization (REMPI) method and their kinetic energies are recorded. The time-of-flight (TOF) technique was used to measure the fragment velocity originally. Recently we have implemented the velocity map imaging (VMI). The ionized photofragments are detected with a large-area position sensitive detector with phosphor screen and the resulting images are recorded with a fast CCD camera. The image processing delivers a detailed 3D information about the photofragment velocities from which the photodissociation dynamics can be learned. More details about imaging and VMI techniques can be found in Chandler and Houston (1987); Eppink and Parker (1997); Gebhardt et al. (2001); and Whitaker (2003).
The next chamber along the beam path contains a new reflectron TOF mass spectrometer (RTOF) with electron and photo-ionization. It has been first implemented and described in our recent studies by Lengyel et al. (2012b, 2013), Kočišek et al. (2013a,b). At the end of the apparatus, the clusters can be also detected and analyzed by a quadrupole mass spectrometer after electron ionization. This option serves the beam alignment and analysis. Also cluster velocities can be measured using a (pseudorandom) chopper and the quadrupole detection to measure the cluster flight-times. Subsequently the cluster pickup cross sections can be evaluated from change in the cluster velocity in the pickup experiments. This method has been described by Fedor et al. (2011b).
Recently we have built another setup for studies of isolated molecules and small clusters: Apparatus for IMaging (AIM). Some of the results reviewed here were obtained on AIM. It implements the velocity mapping according to the design of Eppink and Parker (1997) and it has been described recently by Fedor et al., (2011a); Fárník et al. (2012); Poterya et al. (2014). We have built essentially identical VMI system for CLUB apparatus after testing it on AIM.
Several pulsed UV-laser systems are available in the laboratory: a fixed wavelength (193 nm) excimer laser; and two tunable systems (~200–400 nm) consisting of Nd:YAG lasers, dye lasers and frequency mixing units. In addition, a tunable IR OPO/OPA system can be also used. The laser beams from all these systems can be directed into different viewports in CLUB and AIM apparatus for the various experiments.
3. Results and Discussion
3.1. HNO3 Molecule as Nucleation Center
The PSC particles in the stratosphere can be pure water ice (type II), or ice containing nitric acid (type Ia), and nitric and sulphuric acids (type Ib) (Peter, 1997). To mimic these particles in laboratory experiments we have generated mixed water–nitric-acid clusters by nitric acid vapor expansions. Kay et al. (1981) first investigated these clusters by TOF mass spectrometry. Recently, Lengyel et al. (2012b) have characterized these clusters by mass spectrometry using two complementary ionization methods: (1) electron ionization and (2) photoionization after Na-doping. The analysis of the electron ionization mass spectra suggested that the second (and each subsequent) HNO3 molecule attaches more efficiently to the cluster when the previous HNO3 molecule(s) are already acidically dissociated: for example at least four water molecules are needed to dissociate an HNO3 molecule, therefore (HNO3)2·(H2O)n clusters with two HNO3 molecules appear for n ≥ 4–5 and have presumably the zwitterionic structure NO−3·H3O+·(H2O)n− 1·(HNO3). Analogically, the larger clusters with more HNO3 molecules are efficiently generated when all the previous HNO3 molecules in the cluster dissociate to ion pairs. These results were in accordance with the previous study by Kay et al. (1981). The Na-doping experiments below revealed another important property of mixed HNO3-water clusters: the HNO3 molecule is an effective nucleation center around which the clusters start to grow.
In electron ionization experiments generally the information about the neutral precursor clusters can be obtained rather indirectly from the mass spectra of the ionized fragments. To gain more insight into the nature of the neutral parent clusters the Na-doping method can be applied. This technique has been developed in Buck's laboratory (Bobbert et al., 2002; Zeuch and Buck, 2013) and used for size resolved infrared spectroscopy of large clusters (Forck et al., 2010; 2012; Pradzynski et al., 2012). Yoder et al. (2011) also proposed it as a general method for atmospheric aerosol detection and sizing (see also Forysinski et al., 2011). The experiments are based on the phenomena of solvated electron generation from Na in water clusters. The binding energy of the solvated electron (e.g., ~3 eV for water clusters) is significantly lower than the ionization potential of the cluster constituents. Therefore the clusters can be ionized with single low energy UV photon resulting in soft, essentially fragmentation-free, ionization. The measured mass spectrum after Na-pickup and photoionization thus reveals the original neutral cluster size distribution.
However, in our case of mixed (HNO3)m(H2O)n clusters no signal was detected after the Na doping: this observation contradicted the strong signals from the mixed cluster after electron ionization, and also the strong signals from the pure water clusters after the Na doping. The reason for this absence of signal from the mixed cluster turned out to be the fast charge transfer reaction: Na + HNO3 → Na+ + HNO−3. Therefore the cluster could not be ionized with the low energy photon as in the case of the solvated electron. Thus the Na-doping method as “the sizer for atmospheric aerosols” (Yoder et al., 2011) is limited to aerosols where sodium does not react with the constituent molecules.
This observation implied, that the nitric acid vapor expansions generated clusters which always contained at least one HNO3 molecule, i.e., no pure water clusters were formed.1 This in turn means that the HNO3 molecule acts as a very effective condensation nuclei. Could it play the same role in the atmosphere?
The stratospheric particles containing HNO3 molecules, i.e., type Ia PSCs are the most common ones, and thus determining for the ozone depletion. Yet, large uncertainties remain concerning their formation mechanism and composition (Peter, 1997; Finlayson-Pitts and Pitts, 2000; Prenni and Tolbert, 2001; Stetzer et al., 2006). The generally accepted models usually start with PSCs forming on background stratospheric aerosols composed primarily of sulphuric acid. Prenni and Tolbert (2001) outlined the interplay between nitric and sulphuric acids in PSCs. Although many studies concentrated on nitric acid aerosols, e.g., Dickens and Sloan (2002) and Stetzer et al. (2006), the initial steps of small cluster formation have not been studied in detail. Our present findings suggest that the initial clusters can be efficiently formed around HNO3 molecule.
3.2. Particle Growth and Pickup Cross Sections
The atmospheric processes involving molecules on ice particles start by the pickup process in which the molecule lands on the particle. One of the quantities determining the further processes is the effective cross section of the ice nanoparticle for the pickup of the molecule. We can measure this quantity in our experiment by passing the cluster beam through a cell filled with a particular gas: the molecules collide with the clusters and are adsorbed, and the clusters are slowed down by these inelastic collisions. Cuvellier et al. (1991) demonstrated that by accurate measurements and analysis of cluster velocities after the pickup process the cluster mean sizes N could be determined. To calculate N they assumed that the pickup cross section corresponded to the geometrical one, σg(N) = π R2N (RN is the particle radius). This assumption can be tested, if the mean cluster size N is known (e.g., from other experiments). Then the effective pickup cross section σ(N) can be derived from the velocity measurements. We have carefully tested this method for the well studied argon clusters, compared it to other methods, and confirmed by theoretical simulations: (Fedor et al., 2011b) delivered reliable pickup cross sections for ArN clusters.
Subsequently, Lengyel et al. (2012a) have applied this method to ice nanoparticles (H2O)N (N ≈ 260, RN ≈ 1.2Å). Pickup of various atmospherically relevant molecules has been measured, e.g., water, methane, NOx, hydrogen halides, and some volatile organic compounds. The water cluster mean sizes have been measured previously by Bobbert et al. (2002), therefore we could determine σ from the velocity measurements.
Our study has shown that the pickup cross sections can be significantly larger than the geometrical cross sections. Specifically σ ≈ 1000 Å2 for pickup of water molecules on (H2O)N, N ≈ 260, which is two-times larger than the geometrical cross section σg ≈ 500 Å2. The hexagonal ice density was used to calculate this geometrical cross section. Considering different densities of the cluster, the corresponding σg varied between 400 and 670 Å2. It is also worth mentioning that the above geometrical cross section is in agreement with theoretical calculations (Buch et al., 2004) which take into consideration real water–water potentials and hydrogen bonding in the cluster.
Our own molecular dynamics (MD) simulations were detailed in the corresponding publication (Lengyel et al., 2012a). The geometrical size of our simulated clusters was in agreement with the above σg. Yet the cross section obtained from the MD simulations of the pickup processes on the clusters was 950 Å2 quite in agreement with the measured value. Thus the measured value has been confirmed by the MD simulations within the experimental error bars. More recently we have developed also a semiempirical analytical model which describes the measured cross sections. The polarizability of the molecule and clusters leads to the attraction of the molecules which collide with the clusters with scattering parameters significantly larger than the cluster radius resulting in the larger pickup cross section.
Our results can be compared to the measurements of pickup cross sections for protonated and deprotonated water clusters by Zamith et al. (2010a,b, 2013). In their experiments the charged clusters can be size selected and thus σ(N) for individual cluster sizes can be obtained. Our neutral cluster measurements are performed with the cluster size distributions produced in the expansions and are referred to the mean cluster size σ(N). Also the mass range of the experiments with ionic clusters is somewhat below our usual cluster sizes. Nevertheless, in the region where our data overlap, the agreement between the cross sections of ionic and neutral species is good (Zamith et al., 2013).2
More recently, we have investigated the size dependence of the pickup cross section. It turned out that the data depart even more from the geometrical value for the larger clusters N ≥ 300. In this region they exceed even the values from MD simulations. It appears that highly irregular particle shape for the larger clusters has to be invoked to explain these observations. There is an evidence that the larger clusters in supersonic expansions can grow by smaller cluster coagulation rather than by addition of individual molecules (Bobbert et al., 2002). This together with the directionality of the hydrogen bond in ice can give rise to highly irregular cluster shapes. We are currently working on theoretical simulations of this hypothesis.
It ought to be mentioned that the effective cross section is velocity dependent. We cannot lower the cluster beam velocity substantially to achieve the collision velocities corresponding to the real atmospheric temperatures (below 300 K). However, we can extrapolate the measured σ value using theoretical velocity dependence as outlined by Lengyel et al. (2012a). This extrapolation would yield σ≈ 1400 Å2 at the atmospherically relevant temperatures, i.e., cross section almost by factor of 3 larger than the geometrical value.
The atmospheric nucleation models generally assume the geometrical cross sections of spherical particles even for the small particles in nanometer-size range. For example recently Vehkamäki et al. (2012) have described a model which explicitly treats situations close to our experimentally studied case: the small cluster growth by monomer collisions with the cluster. The nucleation rates in their model are proportional to the collision coefficients which are taken to be the hard sphere collision rates. Thus the nucleation rates are proportional to the particle cross sections. Considering our experimental cross sections rather than the geometrical ones, the nucleation rates would be different by factor of 2–3. Our experiments have also shown that the cross sections differ for different molecules.
3.3. Photochemistry on Nanoparticles
Large amount of attention has been paid to the photochemistry of hydrogen halides in water clusters due to their relevance to the ozone depletion process (Hurley et al., 2002, 2003; Dermota et al., 2005). Photodissociation in clusters has been also investigated for other relevant species, e.g., OClO (Fenner et al., 1997; Kreher et al., 1999), and nitric acid (Li and Huber, 2001). We concentrate on photodissociation dynamics of hydrogen halides and freons in/on large rare gas and water clusters.
3.3.1. Hydrogen halides and hydronium radical
We investigated the HX (X = Cl, Br, I) photodissociation on (H2O)n, n ≈ 102–103, clusters to mimic the UV-photochemistry of hydrogen halides on ice particles in PSCs. The prerequisite for our present experiments were numerous previous studies of (HX)m·Rgn clusters (Rg = Ar, Kr, Xe, Ne) performed originally in Buck's laboratory (Buck, 2002; Slavíček et al., 2003; 2004 Nahler et al., 2004a,b; Fárník et al., 2005; Fárník and Buck, 2006). The rare gas cluster represents a non-reactive solvent environment in which the HX photodissociation proceeds. The understanding of the photodissociation process in this inert solvent is necessary to gain some insight into the comparatively complex HX·(H2O)n system where chemistry can occur.
The rare gas experiments revealed “mechanistic effects” of photodissociating fragment: caging versus direct exit. These processes are expressed in the measured kinetic energy distributions (KED) of H-fragments as peaks at zero kinetic energy and at energies corresponding to the photodissociation of the isolated molecule, respectively, see Figure 2B. The vertical arrows label the energies corresponding to the photodissociation of isolated HCl molecule. The mass spectra Figure 2A serve not only to confirm the pickup of the molecule by the clusters, but also in case of multiple pickup the spectra reveal the dynamics of the molecules on the cluster surface and generation of small (HCl)m clusters. Despite the fact that the rare gas cluster represents an inert solvent environment the observed processes could be quite complex. For example Nahler et al. (2004a); Fárník and Buck (2006); and Poterya et al. (2008b) observed rare gas compounds HXeY (Y = I, Cl, C2H) generation and orientation. Therefore, detailed understanding of the rare gas systems facilitates the interpretation of the more complex results obtained with the water clusters below.
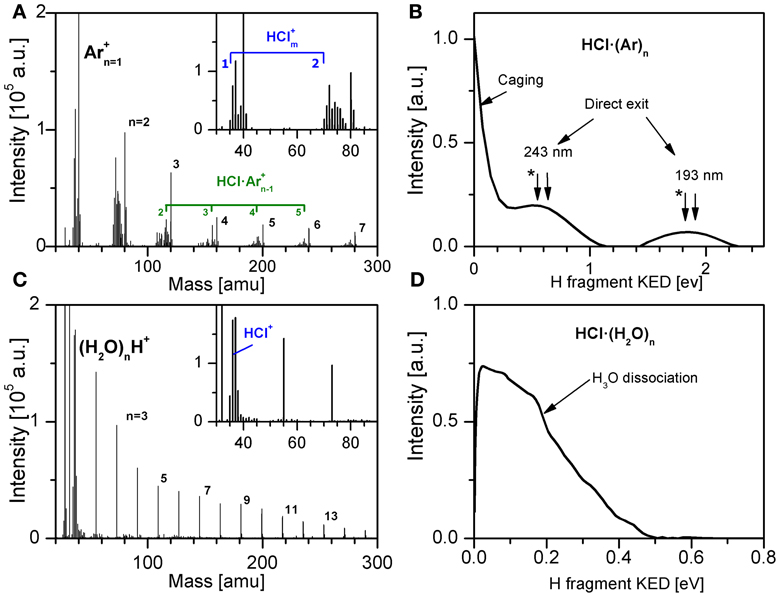
Figure 2. Examples of experimental data measured for HCl pickup on Arn (A,B), and HCl on (H2O)n (C,D): mass spectra (A,C) and H-fragment KED after the photodissociation with 193 nm (B,D). The KE corresponding to H fragment from free HCl molecule resulting in Cl* spin-orbit excited state is labeled by * in (B).
Large water clusters (H2O)n, n ≈ 102–103 were generated in our experiments and HX molecules were deposited on their surface in the pickup process discussed above. Figure 2C shows that the HCl molecules on the water cluster do not coagulate to HCl clusters as opposed to the Ar case shown in Figure 2A. An example of the H-fragment KED measured in several our studies (Poterya et al., 2007, 2008a; 2011; Ončák et al., 2008, 2011) is shown in Figure 2D. It exhibits some differences compared to the rare gas case: the direct exit fast fragments are missing entirely as well as the sharp peak at zero kinetic energy (cage effect); rather slow fragments extending to ≈0.5 eV occur with a broad maximum below 0.1 eV. These KEDs were invariant whether HCl, HBr or HI were adsorbed on (H2O)n, while they were distinctly different for these species on Rgn (the fast direct exit fragments reflect the different energetics of the corresponding HX molecule).
For the water–hydrogen halide system we have to consider the possibility of HX acidic dissociation on the cluster in its ground state. Do the UV-photons excite the covalently bound HX molecule on ice or H3O+·X− ion pair structure? The experimental observation together with an earlier theoretical calculations of Sobolewski and Domcke (2002, 2003, 2007) lead to the proposal that the hydrogen atom originated from species with structure of neutral hydronium radical H3O. This hypothesis has been confirmed by experiments with deuterated species: namely, using HX·(H2O)n, DX·(H2O)n, and HX·(D2O)n and assuming the hydronium radical model, we expect to generate H3O, H2DO, and D2HO in these clusters, respectively. The expected H-atom signal ratio from these species then would be 3:2:1 (assuming the same clusters, expansion and pickup conditions, and observing only the H-signal, not D). This ratio has been confirmed for all three hydrogen halides, supporting the H3O hypothesis. The molecule was acidically dissociated on the ice nanoparticle and the ion pair structure was UV excited to a state of charge-transfer-to-solvent (CTTS) character where it relaxed to a biradical state where the hydrogen was released from H3O. It ought to be mentioned that subsequently there has been found an evidence for the H3O radical also in time dependent experiments of Hydutsky et al. (2009).
Interestingly, no significant H/D scrambling occurred in the clusters upon the acidic ionization and the H3O+·X− ion pair remained of rather local nature. This can be clearly seen from the signal ratios: namely in HX·(H2O)n clusters with n ≈ 500, there is 103-times more H-atoms than in HX·(D2O)n. If the H/D atoms in these clusters were all equivalent (statistical scrambling), there would be 103-times higher probability to detect an H atom from HX·(H2O)n cluster than from HX·(D2O)n. However, the observed ratio of the signals from these two systems was only equal to 3 (not 103) meaning that the H/D exchange did not occur within the clusters.
Our experiments complemented by theoretical investigations have delivered a clear picture of HX·(H2O)n photochemistry: HX first acidically dissociates, the system is photoexcited to a CTTS state, and Cl and H3O radicals are generated. Ončák et al. (2008, 2011) and Poterya et al., (2011) argued that the acidic dissociation in the ground state leads to a significant red-shift of the absorption spectra of the X−H3O+(H2O)n−1 species with possible atmospheric consequences.
There is more than three orders of magnitude increase between 150 and 320 nm (HCl and Cl2 absorption band maximum, respectively) in the actinic flux of photons in the stratosphere at ~50 km altitude. Therefore the ozone depletion models assume conversion of the reservoir species HCl to Cl2 on the ice particles in PSC. Subsequently, Cl2 is photolyzed to release Cl radicals into the stratosphere. However, the above mentioned red shift of the absorption spectra when HCl is adsorbed on the ice particles and acidically dissociated can significantly enhance the direct production of Cl radicals from photodissociation on ice particles (Ončák et al., 2008). Ončák et al., (2011) have outlined some questions which should be addressed in the future, namely: Should these direct processes on ice particles be included in the stratospheric ozone depletion models? How large is the spectral shift for particles of real atmospheric sizes? The shifts in our studies were calculated for fairly small clusters and they are actually smaller for the bulk ice. Will the Cl radicals leave the ice particles after the photodissociation or remain caged? We are currently working on some of these issues.
3.3.2. Photochemistry of freon-12
Recently, we have implemented the imaging and velocity mapping techniques for the photodissociation of molecules in clusters and Fedor et al. (2011a) tested it on the example of HBr photodissociation in rare gas clusters. With this new tool we have concentrated on another important atmospheric molecule, freon-12 CF2Cl2, relevant for stratospheric ozone depletion. In analogy to the hydrogen halides studies, Poterya et al. (2014) have first investigated the freon photodissociation dynamics in the environment of rare gas clusters. The mass spectrum in Figure 3A illustrates that freon molecules tend to generate (CF2Cl2)n clusters in large ArN clusters. On the contrary no clusters were generated in pure freon expansions even at elevated stagnation pressures. The KEDs of Cl-fragments in different clusters exhibit caging and direct dissociation as illustrated in Figure 3B. Besides, the photodissociation dynamics of the bare CF2Cl2 molecule has been investigated by VMI technique in hitherto unreported details. Previous TOF studies (Baum and Huber, 1993; Yen et al., 1993) revealed some controversy concerning slow Cl fragments. Our VMI experiments revealed also some slower fragments in addition to the direct photodissociation yielding the fast fragments with kinetic energy Ekin ≈ 0.97 eV. Several processes were proposed to be responsible for these slow fragments, including concerted dissociation of two Cl atoms from single CF2Cl2 molecule.
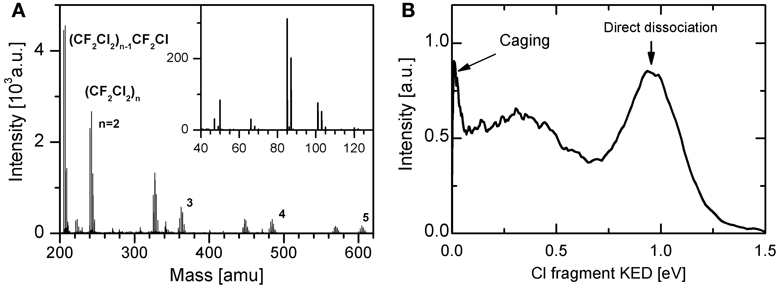
Figure 3. Examples of experimental data measured for CF2Cl2/Ar system: electron ionization mass spectrum (A), and Cl-fragment KED after the photodissociation with 193 nm (B).
There are two important results of our CF2Cl2 photodissociation experiments with possible consequences for atmospheric chemistry. The first one concerns the photodissociation of an isolated CF2Cl2 molecule. The observation of the slow Cl fragments which accounted for more than 20% of the total Cl signal cannot be explained by the direct fission of the C–Cl bond in CF2Cl2 molecule. Several pathways generating these slow fragments have been proposed by Poterya et al. (2014). They involve also concerted dissociation of two Cl atoms from the molecule, or secondary decay of an excited CF2Cl radical. Both these channels would yield two Cl atoms generated by one UV photon. Yet, the quantum yield measurements of Taketani et al. (2005) resulted in the quantum yield Φ = 1 at 193 nm. This value is also recommended for the atmospheric modeling (IUPAC). The proposed mechanisms leading to two Cl atoms released from one molecule upon single photon absorption does not have to be necessarily in discrepancy with the measured quantum yield of 1, if a compensating channel would exist where no Cl fragments were generated upon the photon absorption. The possibility of 2 Cl atoms released by a single UV photon could have consequences for the ozone hole modeling. However, it ought to be mentioned that the possibility of multiphoton processes (193 + 235 nm) could not be entirely excluded from our measurements as discussed by Poterya et al. (2014).
The second important result of the above study concerns the photodissociation in the clusters: it showed that part of the Cl fragments is caged totally in the cluster, while still significant fraction leaves the cluster undisturbed. Only in much larger clusters the probability of the direct exit from the cluster diminishes. The fragments which leave the cluster could contribute to the Cl budged in the atmosphere. Yet, it should be noted that the present experiments were performed for the model systems where the CF2Cl2 molecules were embedded inside the rare gas clusters. Currently we are performing the experiments with CF2Cl2 molecule deposited on the surface of large water clusters.
4. Summary
In summary, the present review shows several examples where detailed molecular level investigations of processes on nanoparticles in laboratory molecular beam experiments can assist understanding of atmospheric processes including ice and aerosol particles. The community of aerosol physicists and chemists has been mainly concentrated on the field measurements and large scale global modeling, while the molecular beam experiments have been focused on single molecules and small clusters. However, the message of the present review is to show that there is a large potential in overlapping these two areas, and that the molecular beams can offer unprecedented detailed insight even into the atmospherically relevant processes.
Funding
Grant Agency of the Czech Republic, grants Nos.: 203/09/0422, P208/11/0161 and 14-08937S.
Conflict of Interest Statement
The authors declare that the research was conducted in the absence of any commercial or financial relationships that could be construed as a potential conflict of interest.
Acknowledgments
The authors would like to acknowledge the contribution of P. Slavíček, J. Fedor, A. Pysanenko, J. Lengyel, and J. Kočišek to the previous studies reviewed in this article.
Footnotes
1. ^Unless the nitric acid was diluted below concentrations of 1 mol% HNO3.
2. ^Zamith et al. (2013) pointed out that the definition of the geometrical cross section σg = π × (RN + rm)2 should include the molecular radius rm. We have omitted rm to have the same σg for the water clusters when different molecules are being picked up by them. Considering this difference, both experiments agree with one another in the region where the data overlap.
References
Baum, G., and Huber, J. R. (1993). Photodissociation of CF2Cl2 at 193 nm investigated by photofragment spectroscopy. Chem. Phys. Lett. 203, 261–264. doi: 10.1016/0009-2614(93)85398-8
Bobbert, C., Schütte, S., Steinbach, C., and Buck, U. (2002). Fragmentation and reliable size distributions of large ammonia and water clusters. Eur. Phys. J. D 19, 183–192. doi: 10.1140/epjd/e20020070
Buch, V., Bauerecker, S., Devlin, J. P., Buck, U., and Kazimirski, J. K. (2004). Solid water clusters in the size range of tens-thousands of H2O: a combined computational/spectroscopic outlook. Int. Rev. Phys. Chem. 23, 375–433. doi: 10.1080/01442350412331316124
Buch, V., and Devlin J. P. (ed.). (2003). Water in Confining Geometries. Berlin: Springer. doi: 10.1007/978-3-662-05231-0
Buck, U. (2002). Photodissociation of hydrogen halide molecules in different cluster environment. J. Phys. Chem. A 106, 10049–10062. doi: 10.1021/jp0208079
Campargue, R. (ed.). (2001). Atomic and Molecular Beams. The State of the Art 2000. Berlin: Springer. doi: 10.1007/978-3-642-56800-8
Chandler, D. W., and Houston, P. L. (1987). Two-dimensional imaging of state-selected photodissociation products detected by multiphoton ionization. J. Chem. Phys. 87, 1445. doi: 10.1063/1.453276
Cuvellier, J., Meynadier, P., de Pujo, P., Sublemontier, O., Visticot, J.-P., Berlande, J., et al. (1991). A simple method to determine the mean cluster size in a molecular beam. Z. Phys. D 21, 265–269. doi: 10.1007/BF01426384
Dermota, T. E., Hydutsky, D. P., Bianco, N. J., and Castleman, A. W. (2005). Photoinduced ion-pair formation in the (HI)m(H2O)n cluster system. J. Chem. Phys. 123, 214308. doi: 10.1063/1.2135291
Dickens, D. B., and Sloan, J. J. (2002). The nucleation and freezing of dilute nitric acid aerosols. J. Phys. Chem. A 106, 10543–10549. doi: 10.1021/jp0259566
Eppink, A. T. J. B., and Parker, D. H. (1997). Velocity map imaging of ions and electrons using electrostatic lenses: application in photoelectron and photofragment ion imaging of molecular oxygen. Rev. Sci. Instrum. 68, 3477. doi: 10.1063/1.1148310
Fárník, M. (2011). Molecular Dynamics in Free Clusters and Nanoparticles Studied in Molecular Beams. Prague: ICT Prague Press. ISBN: 978-80-7080-781-1.
Fárník, M., and Buck, U. (2006). Oriented xenon hydride molecules in the gas phase. Int. Rev. Phys. Chem. 25, 583–612. doi: 10.1080/01442350600847746
Fárník, M., Nahler, N. H., Buck, U., Slavíček, P., and Jungwirth, P. (2005). Photodissociation of HBr on the surface of Arn clusters at 193 nm. Chem. Phys. 315, 161–170. doi: 10.1016/j.chemphys.2005.03.025
Fárník, M., Poterya, V., Kočišek, J., Fedor, J., and Slavíček, P. (2012). Short review on the acetylene photochemistry in clusters: photofragment caging and reactivity. Mol. Phys. 110, 2817–2828. doi: 10.1080/00268976.2012.706389
Fedor, J., Kočišek, J., Poterya, V., Votava, O., Pysanenko, A., Lipciuc, L., et al. (2011a). Velocity map imaging of hbr photodissociation in large rare gas clusters. J. Chem. Phys. 134, 154303. doi: 10.1063/1.3578610
Fedor, J., Poterya, V., Pysanenko, A., and Fárník, M. (2011b). Cluster cross sections from pickup measurements are the established methods consistent. J. Chem. Phys. 135, 104305. doi: 10.1063/1.3633474
Fenner, K., Furlan, A., and Huber, J. R. (1997). Photofragmentation of OClO clusters in a supersonic jet at 360 and 275 nm. J. Phys. Chem. A 101, 5736–5741. doi: 10.1021/jp9710289
Finlayson-Pitts, B. J. (2009). Reactions at surfaces in the atmosphere: integration of experiments and theory as necessary (but not necessarily sufficient) for predicting the physical chemistry of aerosols. Phys. Chem. Chem. Phys. 11, 7760–7779. doi: 10.1039/b906540g
Finlayson-Pitts, B. J., and Pitts, J. N. (2000). Chemistry of the Upper and Lower Atmosphere. San Diego: Academic Press.
Forck, R. M., Dauster, I., Schieweck, Y., Zeuch, T., Buck, U., Ončák, M., et al. (2010). Observation of two classes of isomers of hydrated electrons in sodium-water clusters. J. Chem. Phys. 132, 221102. doi: 10.1063/1.3439393
Forck, R. M., Pradzynski, C. C., Wolff, S., Ončák, M., Slavíček, P., and Zeuch, T. (2012). Size resolved infrared spectroscopy of Na(CH3OH)n (n = 4–7) clusters in the oh stretching region: unravelling the interaction of methanol clusters with a sodium atom and the emergence of the solvated electron. Phys. Chem. Chem. Phys. 9, 3004–3016. doi: 10.1039/c2cp23301k
Forysinski, P., Zielke, P., Luckhaus, D., Corbett, J., and Signorell, R. (2011). Photoionization of small sodium-doped acetic acid clusters. J. Chem. Phys. 134, 094314. doi: 10.1063/1.3559464
Fujii, A., and Mizuse, K. (2013). Infrared spectroscopic studies on hydrogen-bonded water networks in gas phase clusters. Int. Rev. Phys. Chem. 32, 266–307. doi: 10.1080/0144235X.2012.760836
Gebhardt, C. R., Rakitzis, T. P., Samartzis, P. C., Ladopoulos, V., and Kitsopoulos, T. N. (2001). Slice imaging: a new approach to ion imaging and velocity mapping. Rev. Sci. Instrum. 72, 3848. doi: 10.1063/1.1403010
Hanson, D. R. (1995). Reactivity of ClONO2 on H218O ice and organic liquids. J. Phys. Chem. 99, 13059–13061. doi: 10.1021/j100035a003
Heath, C. H., Streletzsky, K. A., Wyslouzil, B. E., Wölk, J., and Strey, R. (2003). Small angle neutron scattering from D2O-H2O nanodroplets and binary nucleation rates in a supersonic nozzle. J. Chem. Phys. 118, 5465–5473. doi: 10.1063/1.1554736
Hurley, S. M., Dermota, T. E., Hydutsky, D. P., and Castleman, A. W. (2002). Dynamics of hydrogen bromide dissolution in the ground and excited states. Science 298, 202–204. doi: 10.1126/science.1075307
Hurley, S. M., Dermota, T. E., Hydutsky, D. P., and Castleman, A. W. (2003). The ultrafast dynamics of HBr-water clusters: influences on ion-pair formation. J. Chem. Phys. 118, 9272. doi: 10.1063/1.1568729
Huthwelker, T., Ammann, M., and Peter, T. (2006). The uptake of acidic gases on ice. Chem. Rev. 106, 1375–1444. doi: 10.1021/cr020506v
Hydutsky, D. P., Bianco, N. J., and Castleman, A. W. (2009). Photochemistry and solvation of HI(H2O)n clusters: evidence of biradical formation. Chem. Phys. Lett. 476, 15–18. doi: 10.1016/j.cplett.2009.05.005
IUPAC. (2000). subcommittee on gas kinetic data evaluation, data sheet PCL15. Available online at: http://www.iupac-kinetic.ch.cam.ac.uk/. Accessed on 15th Dec 2000.
Kay, B. D., Hermann, V., and Castleman, A. W. (1981). Studies of gas-phase clusters: the solvation of HNO3 in microscopic aqueous clusters. Chem. Phys. Lett. 80, 469–474. doi: 10.1016/0009-2614(81)85059-2
Kočišek, J., Lengyel, J., and Fárník, M. (2013a). Ionization of large homogeneous and heterogeneous clusters generated in acetylene-Ar expansions: cluster ion polymerization. J. Chem. Phys. 138, 124306. doi: 10.1063/1.4796262
Kočišek, J., Lengyel, J., Fárník, M., and Slavíček, P. (2013b). Energy and charge transfer in ionized argon coated water clusters. J. Chem. Phys. 139, 214308. doi: 10.1063/1.4834715
Kreher, C., Carter, R., and Huber, J. R. (1999). Photodissociation of OClO and Ar/OClO and H2O/OClO clusters studied by the resonance enhanced multiphoton ionzation-time of flight method. J. Chem. Phys. 110, 3309. doi: 10.1063/1.478196
Kim, Y. J., Wyslouzil, B. E., Wilhelmski, G., Wölk, J., and Strey, R. (2004). Izothermal nucleation rates in a supersonic nozzles and the properties of small water clusters. J. Phys. Chem. A 108, 4365–4377. doi: 10.1021/jp037030j
Klán, P., and Holoubek, I. (2002). Ice (photo)chemistry. Ice as medium for long-term (photo)chemical transformations – environmental implications. Chemosphere 46, 1201–1210. doi: 10.1016/S0045-6535(01)00285-5
Kulmala, M., Kontkanen, J., Junninen, H., Lehtipalo, K., Manninen, H. E., Nieminen, T., et al. (2013). Direct observations of atmospheric aerosol nucleation. Science 339, 943–946. doi: 10.1126/science.1227385
Lengyel, J., Gorejová, R., Herman, Z., and Fáarník, M. (2013). Proton transfer in hydrogen-bonded network of phenol molecules: intracluster formation of water. J. Phys. Chem. A 117, 11225–11232. doi: 10.1021/jp406773s
Lengyel, J., Kočišek, J., Poterya, V., Pysanenko, A., Svrčková, P., Fárník, M., et al. (2012a). Uptake of atmospheric molecules by ice nanoparticles: pickup cross sections. J. Chem. Phys. 137, 034304. doi: 10.1063/1.4733987
Lengyel, J., Pysanenko, A., Kočišek, J., Poterya, V., Pradzynski, C., Zeuch, T., et al. (2012b). Nucleation of mixed nitric acid–water ice nanoparticles in molecular beams that starts with a HNO3 molecule. J. Phys. Chem. Lett. 3, 3096–3101. doi: 10.1021/jz3013886
Li, Q., and Huber, J. R. (2001). The photodissociation of water-clustered HNO3 studied at 193 nm by the LIF method. Chem. Phys. Lett. 345, 415–422. doi: 10.1016/S0009-2614(01)00915-0
Li, T., Donadio, D., and Galli, G. (2013). Ice nucleation at the nanoscale probes no man's land of water. Nat. Commun. 4, 1877. doi: 10.1038/ncomms2918
Lu, Q.-B. (2010). Cosmic-ray-driven electron-induced reactions of halogenated molecules adsorbed on ice surfaces: implications for atmospheric ozone depletion and global climate change. Phys. Rep. 487, 141–167. doi: 10.1016/j.physrep.2009.12.002
MacTaylor, R. S., and Castleman, A. W. (2000). Cluster ion reactions: insights into processes of atmospheric significance. J. Atmos. Chem. 36, 23–63. doi: 10.1023/A:1006376914390
Manka, A., Pathak, H., Tanimura, S., Wölk, J., Strey, R., and Wyslouzil, B. E. (2012). Freezing water in no-man's land. Phys. Chem. Chem. Phys. 14, 4505–4516. doi: 10.1039/c2cp23116f
Marcotte, G., Ayotte, P., Bendounan, A., Sirotti, F., Laffon, C., and Parent, P. (2013). Dissociative adsorption of nitric acid at the surface of amorphous solid water revealed by X-ray absorption spectroscopy. J. Phys. Chem. Lett. 4, 2643–2648. doi: 10.1021/jz401310j
Nahler, N. H., Fárník, M., and Buck, U. (2004a). Search for oriented HXeX molecules from the photolysis of HCl and HBr in xenon clusters. Chem. Phys. 301, 173–182. doi: 10.1016/j.chemphys.2003.11.016
Nahler, N. H., Fárník, M., Buck, U., Vach, H., and Gerber, R. B. (2004b). Photodissociation of HCl and small (HCl)m complexes in and on large Arn clusters. J. Chem. Phys. 121, 1293. doi: 10.1063/1.1763570
Ončák, M., Slavíček, P., Fárník, M., and Buck, U. (2011). Photochemistry of hydrogen halides on water clusters: simulations of electronic spectra and photodynamics, and comparison with photodissociation experiments. J. Phys. Chem. A 115, 6155–6168. doi: 10.1021/jp111264e
Ončák, M., Slavíček, P., Poterya, V., Fárník, M., and Buck, U. (2008). Emergence of charge-transfer-to-solvent band in the absorption spectra of hydrogen halides on ice nanoparticles: spectroscopic evidence for acidic dissociation. J. Phys. Chem. A 112, 5344–5353. doi: 10.1021/jp8012305
Oppliger, R., Allanic, A., and Rossi, M. J. (1997). Real-time kinetics of the uptake of ClONO2 on ice and in the presence of HCl in the temperature range 160 K≤ T ≤200 K. J. Phys. Chem. A 101, 1903–1911. doi: 10.1021/jp963065q
Peter, T. (1997). Microphysics and heterogeneous chemistry of polar stratospheric clouds. Ann. Rev. Phys. Chem. 48, 785–822. doi: 10.1146/annurev.physchem.48.1.785
Poterya, V., Fárník, M., Ončák, M., and Slavíček, P. (2008a). Water photodissociation in free ice nanoparticles at 243 nm and 193 nm. Phys. Chem. Chem. Phys. 10, 4835. doi: 10.1039/b806865h
Poterya, V., Fáarník, M., Slavíček, P., Buck, U., and Kresin, V. V. (2007). Photodissociation of hydrogen halide molecules on free ice nanoparticles. J. Chem. Phys. 126, 071101. doi: 10.1063/1.2709635
Poterya, V., Fedor, J., Pysanenko, A., Tkáč, O., Lengyel, J., Ončák, M., et al. (2011). Photochemistry of HI on argon and water nanoparticles: hydronium radical generation in HI·(H2O)n. Phys. Chem. Chem. Phys. 13, 2250–2258. doi: 10.1039/c0cp01518k
Poterya, V., Kočišek, J., Pysanenko, A., and Fárník, M. (2014). Caging of Cl atoms from photodissociation of CF2Cl2 in clusters. Phys. Chem. Chem. Phys. 16, 421–429. doi: 10.1039/c3cp51926k
Poterya, V., Votava, O., Fárník, M., Ončák, M., Slavíček, P., Buck, U., et al. (2008b). Generation and orientation of organoxenon molecule H-Xe-CCH in the gas phase. J. Chem. Phys. 128, 104313. doi: 10.1063/1.2837656
Pradzynski, C. C., Forck, R. M., Zeuch, T., Slavíček, P., and Buck, U. (2012). A fully size-resolved perspective on the crystallization of water clusters. Science 337, 1529–1532. doi: 10.1126/science.1225468
Prenni, A. J., and Tolbert, M. A. (2001). Studies of polar stratospheric cloud formation. Acc. Chem. Res. 34, 545–553. doi: 10.1021/ar950186k
Preston, T. C., Wang, C. C., and Signorell, R. (2012). Infrared spectroscopy and modeling of co-crystalline CO2·C2H2 aerosol particles. I. The formation and decomposition of co-crystalline CO2·C2H2 aerosol particles. J. Chem. Phys. 136, 094509. doi: 10.1063/1.3690063
Ravishankara, A. R. (1997). Heterogeneous and multiphase chemistry in the troposphere. Science 276, 1058–1065. doi: 10.1126/science.276.5315.1058
Signorell, R., and Reid J. P. (ed.). (2011). Fundamentals and applications in aerosol spectroscopy. Boca Raton, FL: CRC Press; Taylor and Francis Group. doi: 10.1201/b10417
Slavíček, P., Jungwirth, P., Lewerenz, M., Nahler, N. H., Fárník, M., and Buck, U. (2003). Pickup and photodissociation of hydrogen halides in floppy neon clusters. J. Phys. Chem. A 107, 7743–7754. doi: 10.1021/jp0357525
Slavíček, P., Jungwirth, P., Lewerenz, M., Nahler, N. H., Fárník, M., and Buck, U. (2004). Photodissociation of hydrogen iodine on the surface of large argon clusters: the orientation of the librational wave function and the scattering from the cluster cage. J. Chem. Phys. 120, 4498. doi: 10.1063/1.1643895
Sobolewski, A. L., and Domcke, W. (2002). Hydrated hydronium: a cluster model of the solvated electron? Phys. Chem. Chem. Phys. 4, 4–10. doi: 10.1039/b107373g
Sobolewski, A. L., and Domcke, W. (2003). Photochemistry of HCl(H2O)4: cluster model of the photodetachment of the chloride anion in water. J. Phys. Chem. A 107, 1557–1562. doi: 10.1021/jp021533s
Sobolewski, A. L., and Domcke, W. G. (2007). Computational studies of the photophysics of hydrogen-bonded molecular systems. J. Phys. Chem. A 111, 11725–11735. doi: 10.1021/jp075803o
Solomon, S. (1999). Stratospheric ozone depletion: a review of concepts and history. Rev. Geophys. 37, 275–316. doi: 10.1029/1999RG900008
Stetzer, O., Möhler, O., Wagner, R., Benz, S., Saathoff, H., Bunz, H., et al. (2006). Homogeneous nucleation rates of nitric acid dihydrate (nad) at simulated stratospheric conditions – part I: experimental results. Atmos. Chem. Phys. 6, 3023–3033. doi: 10.5194/acp-6-3023-2006
Taketani, F., Takahashi, K., and Matsumi, Y. (2005). Quantum yields for Cl(2Pj) atom formation from the photolysys of chlorofluorocarbons and chlorinated hydrocarbons at 193.3 nm. J. Phys. Chem. A 109, 2855–2860. doi: 10.1021/jp044218+
Vaida, V. (2011). Perspective: water cluster mediated atmospheric chemistry. J. Chem. Phys. 135, 020901. doi: 10.1063/1.3608919
Vehkamäki, H., McGrath, M. J., Kurtén, T., Julin, J., Lehtinen, K. E. J., and Kulmala, M. (2012). Rethinking the application of the first nucleation theorem to particle formation. J. Chem. Phys. 136, 094107. doi: 10.1063/1.3689227
Whitaker, B. (ed.). (2003). Imaging in Molecular Dynamics. Cambridge: Cambridge University Press. doi: 10.1017/CBO9780511535437
Yabushita, A., Inoue, Y., Senga, T., Kawasaki, M., and Sato, S. (2002). Photodissociation of chlorine molecules adsorbed on amorphous and crystalline water ice films. J. Phys. Chem. B 106, 3151–3159. doi: 10.1021/jp012855j
Yacovitch, T. I., Heine, N., Brieger, C., Wende, T., Hock, C., Neumark, D. M., et al. (2012). Vibrational spectroscopy of atmospherically relevant acid cluster anions: bisulfate versus nitrate core structures. J. Chem. Phys. 136, 241102. doi: 10.1063/1.4732148
Yacovitch, T. I., Wende, T., Jiang, L., Heine, N., Meijer, G., Neumark, D. M., et al. (2011). Infrared spectroscopy of hydrated bisulfate anion clusters: HSO4(H2O)1-16. J. Phys. Chem. Lett. 2, 2135–2140. doi: 10.1021/jz200917f
Yen, M., Johnson, P. M., and White, M. G. (1993). The vacuum ultraviolet photodissociation of the chlorofluorocarbons. photolysis of CF3Cl, CF2Cl2, and CFCl3 at 187, 125, and 118 nm. J. Chem. Phys. 99, 126–139. doi: 10.1063/1.465791
Yoder, B. L., Litman, J. H., Forysinski, P. W., Corbett, J. L., and Signorell, R. (2011). Sizer for neutral weakly bound ultrafine aerosol particles based on sodim doping and mass spectrometric detection. J. Phys. Chem. Lett. 2, 2623–2628. doi: 10.1021/jz201086v
Zamith, S., de Tournadre, G., Labastie, P., and L'Hermite, J.-M. (2013). Attachment cross-sections of protonated and deprotonated water clusters. J. Chem. Phys. 138, 034301. doi: 10.1063/1.4775401
Zamith, S., Feiden, P., Labastie, P., and L'Hermite, J.-M. (2010a). Sticking properties of water clusters. Phys. Rev. Lett. 104, 103401. doi: 10.1103/PhysRevLett.104.103401
Zamith, S., Feiden, P., Labastie, P., and L'Hermite, J.-M. (2010b). Attachment cross sections of protonated water clusters. J. Chem. Phys. 133, 154305. doi: 10.1063/1.3505302
Keywords: molecular beams, photodissociation, water clusters, atmospheric chemistry, aerosols, photochemistry, molecular dynamics
Citation: Fárník M and Poterya V (2014) Atmospheric processes on ice nanoparticles in molecular beams. Front. Chem. 2:4. doi: 10.3389/fchem.2014.00004
Received: 16 October 2013; Accepted: 31 January 2014;
Published online: 24 February 2014.
Edited by:
Alkwin Slenczka, Universität Regensburg, GermanyReviewed by:
Leonid Surin, University of Cologne, GermanyGereon Niedner-Schatteburg, Technische Universität Kaiserslautern, Germany
Marcel Mudrich, Albert-Ludwigs-Universität Freiburg, Germany
Copyright © 2014 Fárník and Poterya. This is an open-access article distributed under the terms of the Creative Commons Attribution License (CC BY). The use, distribution or reproduction in other forums is permitted, provided the original author(s) or licensor are credited and that the original publication in this journal is cited, in accordance with accepted academic practice. No use, distribution or reproduction is permitted which does not comply with these terms.
*Correspondence: Michal Fárník, Laboratory of Molecular and Cluster Dynamics, Department of Ion and Cluster Chemistry, J. Heyrovský Institute of Physical Chemistry, Academy of Sciences of the Czech Republic, Dolejškova 3, Prague 8, 18223, Czech Republic e-mail:bWljaGFsLmZhcm5pa0BqaC1pbnN0LmNhcy5jeg==