- Graduate School of Environmental Science, Hokkaido University, Sapporo, Japan
Gibberellic acid (GA3), indole-3-acetic acid (IAA), salicylic acid (SA), abscidic acid (ABA), jasmonic acid (JA) 1-amino cyclopropane-1-carboxylic acid (ACC) and aminoethoxyvinylglycine (AVG) are popular growth regulators of plants. However, the effects of their exogenous addition on the biomass production of aquatic plants, including Lemnoideae plants, “duckweeds,” are largely unknown. In this study, the growth of Lemna minor was tested for 10 d in Hoagland medium containing each compound at different concentrations of 0–50 μM. GA3, IAA, and SA were found to have no apparent positive effect on the growth at all concentrations tested. Conversely, ACC and JA moderately and AVG and ABA severely inhibited the growth of L. minor. Among the tested compounds, ascorbic acid had an apparent growth-promoting effect.
Introduction
Duckweeds, a general name for plants belonging to the Lemnoideae subfamily, represent the smallest free-floating monocotyledonous aquatic plants with vascular systems. Lemnoideae includes five genera: Landoltia, Lemna, Spirodela, Wolffiella, and Wolffia. The plant body consists of “frond,” leaf-like structure, and “root” (except for Wolffiella and Wolffia, which are rootless duckweeds). Some duckweeds develop seed-like “turion,” which contains high starch and anthrocyanin depending on the temperature, light and nutrient conditions (Smart and Trewavas, 1983). Although duckweeds are flowering plants, they primarily reproduce asexually by vegetative propagation. Duckweeds are robust, environmentally well-adapted, and ubiquitously distributed from 55° S to 70° N. They were recently highlighted as a potential biomass.
The following are the advantages of duckweeds as biomass: (1) they grow at significantly high rates; (2) they grow on wastewater and uptake and remove contaminant minerals as nutrients; (3) they can be used as slow, but low-cost wastewater treatment systems driven by sun light; (4) they provide nonfood competitive biomass with high protein and starch, but low lignin and cellulose content; and (5) they change their constituent starch and protein content depending on the growth condition. Thus, duckweeds are expected to be one of the next-generation biomass resources to be associated with animal feed, biofuel and starch-based green chemistry (Cheng, 2010; Baliban et al., 2013). Increase in the growth rate of duckweeds should contribute to the increasing availability of duckweed biomass and allow the prevention of global warming and realization of sustainable industries and societies. Increasing attention is being paid to producing duckweeds coupled with wastewater treatment (Xu et al., 2012; Zhao et al., 2014; Toyama et al., 2018).
Researchers have long attempted to increase the growth of plants by adding chemical compounds in addition to mineral fertilizers. In 1917, Bottomley reported that water extracts from bacterized peat contained certain growth-promoting organic compounds for Lemna minor, namely, auximones (Bottomley, 1917, 1920). Gorham clearly showed that sucrose at concentrations higher than 1% increased the growth of Spirodela polyrhiza (Gorham, 1945). Landolt found that sucrose promoted the growth of all Lemnoideae strains tested under low light intensities, and this effect was attenuated under higher intensities (Landolt, 1957). Lactic acid has been known to act as a plant growth regulator for carrot, marigold, sunflower and sugarcane (Hildebrandt et al., 1954). Further, dimers or larger polymers of l-lactic acid at 1,000 ppm were shown to promote the growth of both L. minor and Zea mays L. by 2-fold (Kinnersley et al., 1990). Organic fertilizer is now widely used in crop and vegetable farms.
Developing technologies that enable duckweed growth by the addition of chemical compounds requires the understanding of the basic traits of duckweeds exposed to exogenously added representative bioactive organic compounds. Plant growth regulator compounds, including plant hormones, profoundly influence the growth and differentiation of plant cells, tissues, and organs. Many biotic and abiotic chemical compounds are known to affect plants; for example, regulation of cell size and cell division by ethylene, regulation of cell cycle by auxins (IAA) and cytokines, induction of seed germination and stem elongation by gibberellins (GA), maintenance of seed dormancy by abscisic acid (ABA) and an endogenous signaling against pathogens and abiotic stresses by salicylic acid (SA) and jasmonic acid (JA).
In aquatic plants such as L. minor, the effects of growth regulators on biomass production have rarely been investigated. Gibberellic acid (GA3) has been reported to homeopathically potentize and reduce the growth rate of L. gibba (Scherr et al., 2009). However, the reproducibility of the result was confirmed later by the same group, showing that growth was increased by GA3 (Majewsky et al., 2014). Scherr et al. (2007) also found that eleven potentized compounds, including IAA, decreased the growth of L. gibba. Idris et al. (2007) prepared three mutants of plant growth-promoting Bacillus amyloliquefaciens FZB42 that showed reduction of 15–38% in the level of IAA. The growth-promoting activity of these mutants against L. minor concomitantly reduced to 17–19%, suggesting that IAA is a predominant plant growth-promoting factor. These controversial and confusing findings prompted us to determine comprehensively the effect of exogenous general plant growth regulator compounds on the growth of L. minor.
Materials and Methods
Duckweed Culture
Sterile L. minor RDSC #5512 isolated from the Botanical Garden of Hokkaido University was used in this study. About 50 fronds of L. minor were aseptically transferred every 10 d to new 500 mL flasks containing 250 mL Hoagland medium for subculture. Hoagland medium contains per liter of 36.1 mg KNO3, 293 mg K2SO4, 103 mg MgSO4-7H2O, 147 mg CaCl2-2H2O, 5 mg NaHPO4-2H2O, 0.95 mg H3BO3, 0.39 mg MnCl2-4H2O, 0.03 mg CuSO4-5H2O, 0.08 mg ZnSO4-7H2O, 0.23 mg H2MoO4, and 3.33 mg FeSO4-7H2O. The pH was adjusted to 7.0 using sodium hydroxide. Plant culture conditions were 28°C, 60–70% humidity, 5000 lux (75 μmol/m2/s photon density) and photoperiod of 16 h light / 8 h dark. Sterility of L. minor was confirmed when no bacterial colony was formed on R2A agar plate after incubation at 30°C for 3 d. R2A agar contained per liter 0.50 g each of yeast extract, proteose peptone (Difco no. 3), casamino acids, glucose, and soluble starch; 0.3 g each of Na-pyruvate and K2HPO4; and 0.05 g MgSO4-7 H2O. The pH was adjusted to 7.2 using sodium hydroxide. Agar was added at 15 g before autoclaving.
Plant Growth Regulators
The plant growth regulator compounds used in this study were GA3 (Sigma Aldrich), indole-3-acetic acid (IAA; Wako Pure Chemical Ind. Ltd.), SA (Wako), 1-aminocyclopropane-1-carboxylic acid (ACC; Wako), aminoethoxyvinylglycine (AVG; Sigma Aldrich). Ascorbic acid (Wako) was also tested for the plant growth experiment. Stock solution of chemicals was prepared in MilliQ water and filter-sterilized.
Evaluation of the Growth of L. minor
Sterile L. minor was transferred to Petri dish containing appropriate amount of Hoagland medium, its two fronds were separated using a pair of forceps and inoculated into 100 mL flasks containing each compound in 50 mL Hoagland medium. Flasks were prepared in three replicates for every experiment. The effects of these compounds on plant growth were quantified by evaluating four parameters: frond numbers, fresh weight or dry weight, root length and chlorophyll a+b content. After the number of fronds was counted, five plants of 10 d culture were randomly obtained from each flask, and the length of the longest roots was measured as the root length for every treatment. For the fresh weight, whole plants in the flask were carefully removed, blotted dry on paper towel, and weighed by a micro-scale (Sartorius, Göttingen Germany). Dry weight was measured in nylon tea bag filters after freeze drying (FDU-1110; EYELA).
Chlorophyll contents of L. minor were measured as previously described (Suzuki et al., 2014). Briefly, several plants were placed into 2 mL Eppendorf tubes containing 0.5 g of 0.1 mm glass beads (YGB01, Yasui Kikai, Japan). Next, 1 mL of cold ethanol previously saturated with Ca(CO3)2 was added and vigorously shaken by using a Multi-beads shocker MB755U(S) (Yasui Kikai) at 2,700 vibration per minute for 300 s at 4°C. After glass beads and cell debris were removed by centrifugation, the chlorophyll content was quantified by measuring photometric absorption at 649 and 665 nm. The concentration in units of μg/mL of chlorophyll a was calculated as [13.5275 (A665) – 5.2007 (A649)] and chlorophyll b as [−7.0741 (A665) + 22.4327 (A649)]. The chlorophyll content was determined as mg chlorophyll a+b/g fresh weight of specimens. Statistical significance of each value was validated by unpaired t-test and one-way ANOVA using triplicate samples.
Results and Discussion
GA3
Rice farmers of Japan had long noted a fungal disease called “foolish seedling” or bakanae disease that causes rice plants to grow taller and reduces seed production. Plant pathologists found that this symptom in rice plants was caused by chemicals secreted by a pathogenic fungus, Gibberella fujikuroi. These fungal compounds possessing plant growth promoting activity were later named gibberellins A1, A2, and A3 or gibberellic acid (Takahashi et al., 1955). GA3 can also promote the growth of maize by inhibiting peroxidase secretion into the apoplast (Fry, 1979). The treatment of maize plants with GA3 increased the length of the leaf blade elongation zone compared with that in the control via an increase in cell division and cell elongation duration (de Souza and MacAdam, 2001). In other plants such as potatoes as well, GA3 treatments significantly increased the number of sprouts, stems, and tubers at very high concentrations of 100 mM in the cultivar “Fambo,” but the total biomass did not increase (Virtanen et al., 2013). Oota and Tsudzuki (1971) reported that the number of fronds was increased to 125% by the application of 100 μM GA3. Inada and Shimmen (2000) investigated the effects of the exogenous addition of GA3 to L. minor. These researchers observed no significant changes in root length up to 1 μM, but found that it slight decrease at 10 μM concentration. Conversely, they also reported that exogenous addition of >1 mM GA3 to the root segment of L. minor for 12 h inhibited root elongation in a concentration-dependent manner up to 1 μM (Inada and Shimmen, 2000). These researchers attributed this inconsistency to the fact that the root tip part was not included in the root segments used and the duration of exposure to GA3 differed. However, they reported that uniconazole-P (Un-P), a gibberellin biosynthesis inhibitor, also inhibited the elongation of segments at 10 nM similar to the decrease in the root length in the experiment involving whole plants. These data show that the effect of GA3 on the growth of L. minor is still controversial.
In our experiment, L. minor was grown in the presence of different concentrations of GA3 ranging from 0 to 50 μM in Hoagland medium (Figure 1). After 10 d, the growth traits of L. minor, such as the frond number, root length, fresh weight, and chlorophyll content, were observed. Both the number of fronds and root length were not considerably different among the treatments, suggesting that L. minor is insensitive to exogenous GA3 at these concentrations (Figures 1A,C). This result was also supported by the fresh weight of the plants (Figure 1B). Conversely, the chlorophyll content was increased from 0.37 to 0.59 mg g−1 fresh weight after treatment with 5 μM GA3 (Figure 1D). On the other hand, we observed that ABA, another endonegenous stress signaling compound and an antagonist of GA severely inhibited the growth of L. minor (Supplementary Figure 1).
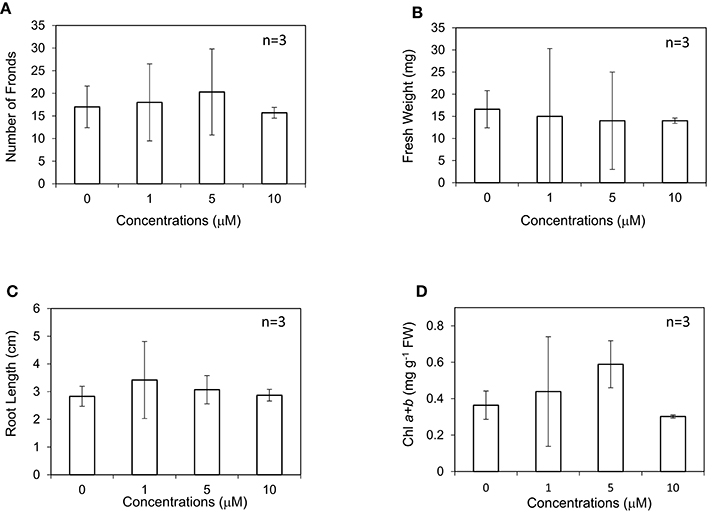
Figure 1. Effect of gibberellic acid (GA3) on the frond number (A), fresh weight (B), root length (C), and chlorophyll content (D) of Lemna minor. Each value was measured after 10 d of cultivation. SD values are shown in line segments.
IAA
Indole-3-acetic acid is the principal auxin in plants; it controls many important physiological processes, including cell enlargement and division, tissue differentiation, and responses to light and gravity (Taiz and Zeiger, 1998). Auxins stimulate cell elongation and influence a host of other developmental responses such as root initiation, vascular differentiation, tropic responses, apical dominance, and the development of auxiliary buds, flowers and fruits. Auxins are synthesized in the stem and root apices and transported through the plant axis. Several other indole derivatives, as precursors to IAA, are known to express auxin activity, probably by converting to IAA in the tissue. This hormone is widely used in agriculture and horticulture to prevent leaf abscission and fruit drop, promote flowering and fruiting, and control weeds. Exogenous application of IAA has been reported to increase the growth of the root and shoot of wheat seedlings and to protect plants against stress (Egamberdieva, 2009). Yang et al. (1993) reported significant promotion of stem elongation in pea seedlings by exogenous IAA at concentrations from 50 to 1,000 μM. Conversely, exogenous IAA at 1 μM was found to retard germination and decrease the fresh weight of germinated soybean seeds. A small, but significant growth promotion by IAA up to 25 ppm (143 μM) was reported for crude culture of L. minor (Blackman and Robertson-Cuninghame, 1954); they also noted frond epinasty and root inhibition at higher concentrations. Conversely, Inada and Shimmen (2000) reported that exogenous IAA up to 1 μM inhibited the elongation of root segments in L. minor.
In this study, L. minor was grown in the medium containing different concentrations of IAA at 0–50 μM. The root length was found to decrease by approximately 50% at all the concentrations of IAA higher than 1 μM (Figure 2C; Inada and Shimmen, 2000). Conversely, frond numbers, fresh weight, and chlorophyll content of L. minor were not affected by the addition of IAA except for significant growth inhibition at 50 μM (Figures 2A,B,D). Notably, the amount of chlorophyll was not decreased even after treatment with 50 μM IAA. We also tested IAA at 0.1 and 0.5 μM but no plant growth-promoting activity was observed (data not shown). In contrast to the generally positive effects of IAA on the growth of soil plants, no plant growth-promoting activity was observed in our experiment for L. minor.
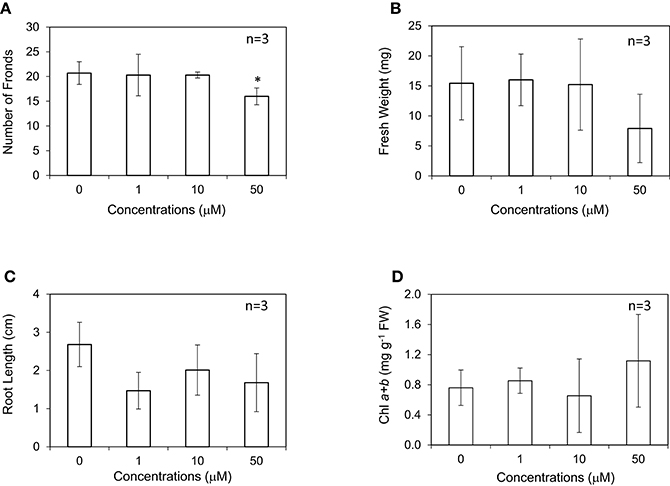
Figure 2. Effect of indole-3-acetic acid (IAA) on the frond number (A), fresh weight (B), root length (C), and chlorophyll content (D) of Lemna minor. Each value was measured after 10 d of cultivation. SD values are shown in line segments. *p < 0.05 against 0 μM control.
SA
Salicylic acid is an endogenous signaling compound abundantly produced under abiotic and biotic stresses, including drought, temperature, heavy metals, and pathogen infection. Despite its broad distribution in plants, SA has basal levels differing widely among species, with up to 100-fold differences been reported (Raskin et al., 1990). The role of SA in photosynthetic parameters and short-term acclimation to high light was deduced from the phenotypes shown by Arabidopsis thaliana plants with contrasting endogenous SA levels. Moreover, the effects of exogenous SA on photosynthesis parameters were found to differ depending on the dose and plant species tested. High concentrations (1–5 mM) of SA reduced the photosynthetic rate and RuBisCO activity in barley plants (Pancheva and Popova, 1998). A. thaliana mutants with different endogenous levels of SA showed growth phenotype that was inversely correlated to SA content (Vicente and Plasencia, 2011).
Shimakawa et al. (2012) reported that the amount of endogenous SA was increased under nutrient-poor conditions, and exogenous SA application induced flowering in Lemna aequinoctialis. This suggests that SA in Lemnoideae plants is also a signaling compound in response to environmental stresses. Recently, the exogenous application of 50 μM SA was reported to reduce the accumulation of Cd, leading to less toxicity (Lu et al., 2018). In this study, L. minor was grown in the medium containing different concentrations of SA at 0–50 μM. SA did not significantly affect the number of fronds, but generally and clearly decreased fresh weight and root length of L. minor (Figures 3A–C). Although the range of errors is large, the amount of chlorophyll was not found to decrease (Figure 3D). Another endogenous stress signaling compound, JA clearly inhibited the growth of L. minor (Supplementary Figure 1).
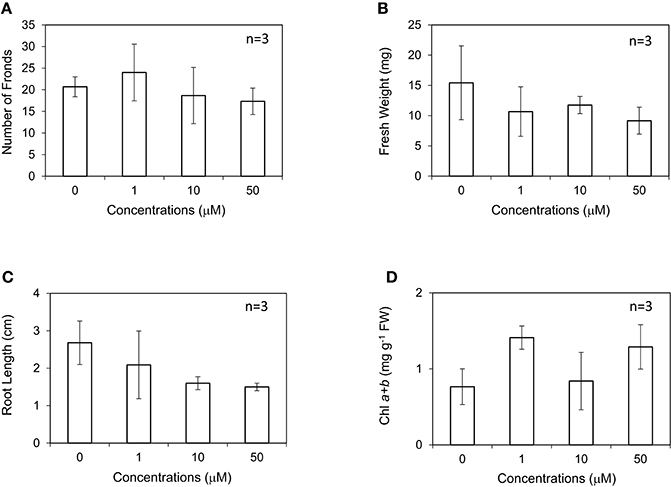
Figure 3. Effect of salicylic acid (SA) on the frond number (A), fresh weight (B), root length (C), and chlorophyll content (D) of Lemna minor. Each value was measured after 10 d of cultivation. SD values are shown in line segments.
ACC
Ethylene affects plant growth at all stages of development from seed germination to organ senescence and promotion of fruit ripening (Corbineau et al., 2004). Ethylene is known to be a key initiator of fast underwater elongation of submerged plants (Jackson, 2008). Treatments of submerged and non-submerged Oryza sativa with ethylene stimulated internode elongation (Métraux and Kende, 1983). Moreover, when ethylene synthesis was blocked with aminooxyacetic acid and aminoethoxyvinylglycine (AVG) in partially submerged plants, internode elongation was inhibited. This growth inhibition was reversed when ethylene biosynthesis was restored with ACC, an immediate precursor of ethylene. ABA and ethylene are known to have antagonistic functions for controlling plant growth and development, including seed germination and early seedling development (Cheng et al., 2009). External addition of ACC to plants changes the convergent point between these two signaling pathways. Under light condition, A. thaliana shows an elongated hypocotyl and shortened root growth in the presence of 1 μM exogenous ACC (Smalle et al., 1997). When grown in darkness, both the hypocotyls and roots were reduced in the wild type at 0.5 μM and in the are2 mutant at 10 μM of exogenous ACC (Vanderstraeten and Straeten, 2017).
Ethylene is known to be a gibbosity regulator of L. gibba and L. minor (Elzenga et al., 1980); however, its effect on growth has not been investigated. In this study, L. minor was grown with different concentrations of ACC under 16-h light photoperiod condition. In contrast to submerged plants, the effects of exogenous ACC on L. minor growth were apparently negative (Figures 4A–C). Addition of 1–50 μM of ACC significantly decreased the frond numbers, fresh weight, and root length. This might be explained by the effect of enhanced ethylene production in the plant body that reduced the growth and development. With regard to the effect of ACC on chlorophyll content, although the range of errors was large, the amount of chlorophyll was surprisingly increased up to 50 μM ACC (Figure 4D).
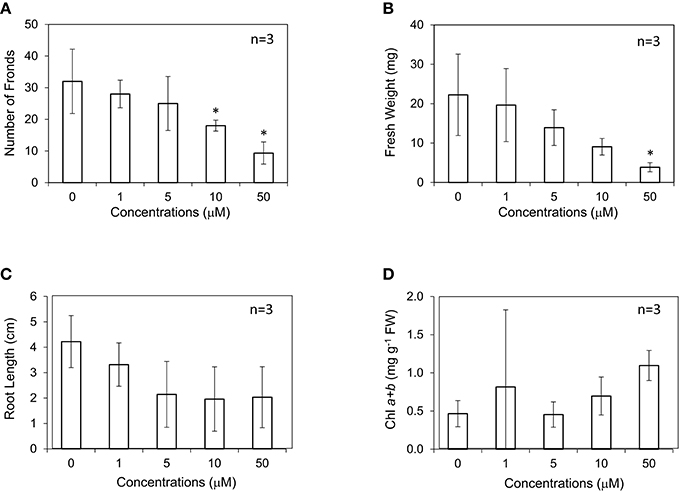
Figure 4. Effect of 1-aminocyclopropane-1-carboxyl acid (ACC) on the frond number (A), fresh weight (B), root length (C), and chlorophyll content (D) of Lemna minor. Each value was measured after 10 d of cultivation. SD values are shown in line segments. *p < 0.05 against 0 μM control.
AVG
AVG is often used as a specific inhibitor of ethylene biosynthesis to analyze the effects of ethylene on plant growth, development and stress response (Abeles et al., 1992) that potentially can mitigate growth suppression under stress condition. Further, the addition of 1 μM of AVG was found to significantly suppress the pistil length of L. gibba (Mader, 2004) but no report about the effect of AVG on overall plant growth are yet available.
AVG remarkably inhibited the growth of L. minor. AVG decreased the frond number, fresh weight and chlorophyll content of L. minor at all concentrations (Figures 5A–D). Roots were lost in almost all the surviving plants. Growth of L. minor was also impaired by the addition of very low concentration (0.5 μM) of AVG (data not shown). Interestingly, the amount of chlorophyll was decreased, but not as much as the degree of growth defect (Figure 5D). Only this trait can be rationally explained by the inverse effect of ACC and AVG on the biosynthesis of ethylene. However, our experimental results indicate that AVG is not a compound useful for enhanced production of duckweed biomass. Notably, spraying of AVG onto the shoots of cotton blocked ethylene accumulation in the leaves under waterlogging stress. This application subsequently improved leaf growth and enhanced the production of both fruit and cotton cultivars even under non-waterlogging condition (Najeeb et al., 2015).
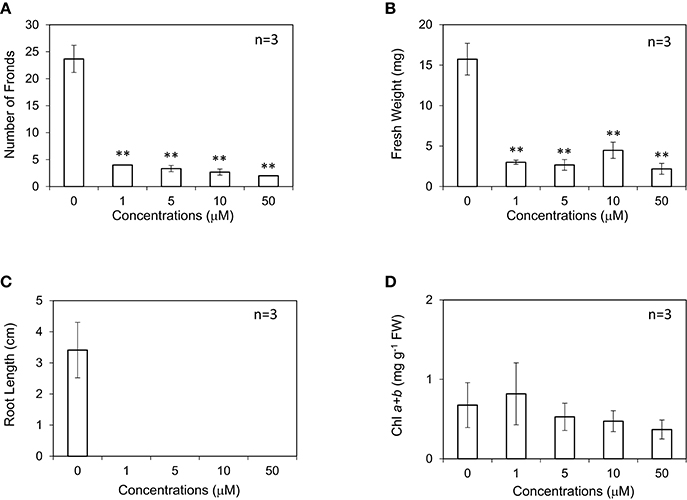
Figure 5. Effect of aminoethoxyvinylglycine (AVG) on the frond number (A), fresh weight (B), root length (C), and chlorophyll content (D) of Lemna minor. Each value was measured after 10 d of cultivation. SD values are shown in line segments. **p < 0.01 against 0 μM control.
AsA
L-Ascorbic acid (AsA) is an abundant water-soluble antioxidant in plants that is capable of scavenging toxic reactive oxygen species (ROS) emitted during photosynthesis. Overproduction of ROS is triggered by various environmental stress-induced oxidative damages. Exogenous AsA is known to protect lipids and proteins against oxidative stresses, thereby promoting the growth of plants. Application of AsA to foliar has been reported to improve the growth of strawberry plants under high temperature condition (44°C; Ergin et al., 2014). For example, exogenous AsA at 3 mM increased cell turgidity of the strawberry plants and hence their growth. Foliar or seed treatment with 20 and 40 mg L−1 AsA improved the seedling growth and yield of maize under low temperature stress (Ahmad et al., 2014). Moreover, exogenous application of AsA has been found effective in mitigating the adverse effects of water stress in wheat (Hafez and Gharib, 2016). Once used, AsA can be recycled by several different mechanisms in the plant cells (Gallie, 2013; Akram et al., 2017).
AsA showed significant growth-promoting activity in L. minor. The highest increase in frond number was observed at 10 ppm, 57 μM AsA, which was 1.7-fold higher than that in the control experiment with no AsA addition (Figures 6A,B). The number of fronds was slightly lower at 50 ppm AsA than at 10 and 5 ppm AsA; however, the dry weight of plants was equivalent or even slightly higher (Figure 6C). All fronds of L. minor grown in the presence of AsA showed higher chlorophyll content than that in the control, suggesting that AsA reduced ROS accumulation in the apoplast. The chlorophyll content after treatment with 10 ppm AsA was higher than that in the control (Figure 6D). Recently, Ishizawa et al. (2017) reported that L. minor accumulated ROS and expressed an evident antioxidant enzyme activity. Furthermore, the antioxidant enzyme activity was markedly increased in the presence of plant growth-inhibiting bacteria. These results are not inconsistent with our observation that an antioxidant AsA increased the growth of L. minor.
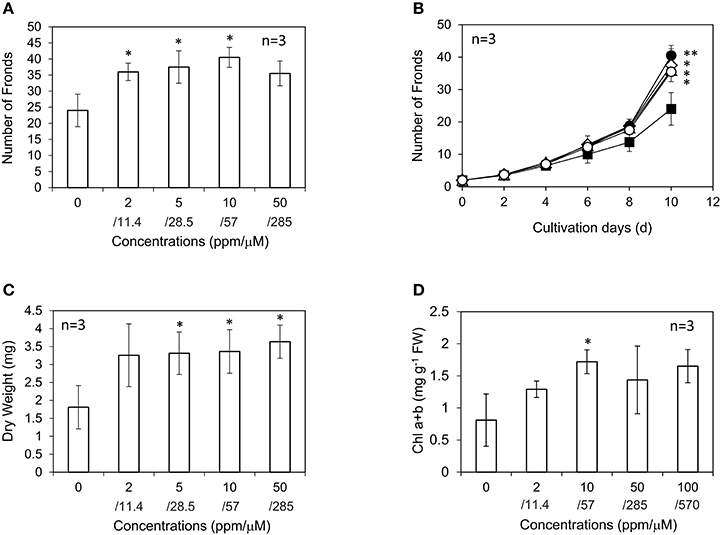
Figure 6. Effect of ascorbic acid on the frond number (A), frond number during 10 d (B), dry weight (C), and chlorophyll content (D) of Lemna minor. Each value was measured after 10 d of cultivation except for (B). Symbols are 0 ppm, closed square; 2 ppm, open triangle; 5 ppm, open diamond; 10 ppm, closed circle; 50 ppm, open circle. SD values are shown in line segments. *p < 0.05 against 0 μM control. **p < 0.01 against 0 μM control.
Conclusions
• Exogenous application of the plant hormones gibberellic acid (GA3), indole-3-acetic acid (IAA), and salicylic acid (SA) showed no apparent growth-promoting effect on frond number and fresh weight of L. minor. Root length was tended to be decreased by IAA and SA. Jasmonic acid (JA) moderately and abscidic acid (ABA) severely inhibited the growth of L. minor.
• Growth of L. minor was inhibited by both antagonistically functioning compounds in ethylene production, ACC and AVG, where the latter acted more severely. These results suggest that L. minor, a Lemnoideae plant, behaves uniquely against plant growth regulator compounds.
• AsA significantly promoted the growth of L. minor under normal growth condition at concentrations higher than 2 ppm (11.4 μM). To the best of our knowledge, AsA has till date not been shown to promote the growth of Lemnoideae plants.
Author Contributions
DU, AK, RJ, and MS designed and performed the experiments and drafted the manuscript. KM and MM interpreted the results revised the manuscript and supervised the study.
Conflict of Interest Statement
The authors declare that the research was conducted in the absence of any commercial or financial relationships that could be construed as a potential conflict of interest.
Acknowledgments
This study was supported by the Advanced Low Carbon Technology Research and Development Program (ALCA, grant number JPMJAL1108) of the Japan Science and Technology Agency (JST), Japan Society for the Promotion of Science (JSPS) KAKENHI (grant number, 16K14844) and the Kobayashi International Scholarship Foundation.
Supplementary Material
The Supplementary Material for this article can be found online at: https://www.frontiersin.org/articles/10.3389/fchem.2018.00251/full#supplementary-material
References
Abeles, F. B., Morgan, P. W., and Saltveit, M. E. (1992). Ethylene in Plant Biology, 2nd Edn. Cambridge: Academic Press.
Ahmad, I., Basra, S. M. A., and Wahid, A. (2014). Exogeneous application of ascorbic acid, salicylic acid, hydrogen peroxide improves the productivity of hybrid maize at low temperature stress. Int. J. Agric. Biol. 16, 825–830.
Akram, N. A., Shafig, F., and Ashraf, M. (2017). Ascorbic acid- A potential oxidant scavenger and its role in plant development and abiotic stress tolerance. Front. Plant. Sci. 8:613. doi: 10.3389/fpls.2017.00613
Baliban, R. C., Elia, J. A., Floudas, C. A., Xiao, X., Zhang, Z., Li, J., et al. (2013). Thermochemical conversion of duckweed biomass to gasoline, diesel and jet fuel: process synthesis and global optimization. Ind. Eng. Chem. Res. 52, 11436–11450. doi: 10.1021/ie3034703
Blackman, G. E., and Robertson-Cuninghame, R. C. (1954). Interactions in the physiological effects of growth substances in plant development. J. Exp. Bot. 5, 184–203. doi: 10.1093/jxb/5.2.184
Bottomley, W. B. (1917). Some effects of organic growth-promoting substances: (Auximones) on the growth of Lemna minor in mineral culture solutions. Proc. R. Soc. Lond. Ser B 89, 481–507. doi: 10.1098/rspb.1917.0007
Bottomley, W. B. (1920). The growth of Lemna plants in mineral solutions and in their natural media. Ann. Bot. 34, 345–352 doi: 10.1093/aob/os-34.3.345
Cheng, J. (2010). “Biological process for ethanol production,” in Biomass to Renewable Energy Processes, ed J. Cheng (Boca Raton, FL: CRC Press), 209–270.
Cheng, W. H., Chiang, M. H., Hwang, S. G., and Lin, P. C. (2009). Antagonism between abscisic acid and ethylene in Arabidopsis acts in parallel with the reciprocal regulation of their metabolism and signaling pathways. Plant. Mol. Biol. 71, 61–80. doi: 10.1007/s11103-009-9509-7
Corbineau, F., Xia, Q., Bailly, C., and El-Maarouf-Bouteau, H. (2004). Ethylene, a key factor in the regulation of seed dormancy. Front. Plant Sci. 5:539. doi: 10.3389/fpls.2014.00539
Elzenga, J. T. M., De Lange, L., and Hipeterse, A. H. (1980). Further indications that ethylene is the gibbosity regulator of the Lemna gibba/Lemna minor complex in natural waters. Acta Bot. Neerl. 29, 225–229. doi: 10.1111/j.1438-8677.1980.tb01199.x
Egamberdieva, D. (2009). Alleviation of salt stress by plant growth regulators and IAA producing bacteria in wheat. Acta Physiol. Plant. 31, 861–864. doi: 10.1007/s11738-009-0297-0
Ergin, S., Aydogan, C., Ozturk, N., and Turhan, E. (2014). Effects of ascorbic acid application in strawberry plants during heat stress. Turk. J. Agric. Nat. Sci. 2, 1486–1491.
Fry, S. C. (1979). Phenolic components of the primary cell wall and their possible role in the hormonal regulation of growth. Planta 146, 343–351. doi: 10.1007/BF00387807
Gallie, D. R. (2013). The role of L-ascoric acid recycling in responding to environmental stress and in promoting plant growth. J. Exp. Bot. 64, 433–443. doi: 10.1093/jxb/ers330
Gorham, P. R. (1945). Growth factor studies with Spirodela polyrhiza (L.) Schleid. Am. J. Bot. 32, 496–505. doi: 10.1002/j.1537-2197.1945.tb05150.x
Hafez, E. M., and Gharib, H. S. (2016). Effect of exogenous application of ascorbic acid on physiological and biochemical characteristics of wheat under water stress. Int. J. Plant Prod. 19, 579–596.
Hildebrandt, A. C., Riker, A. J., and Watertor, J. I. (1954). Growth and inhibition of tissue cultures on media with different concentration of organic acids. Phytopathology 44, 422–428.
Idris, E. E., Iglesias, D. J., Talon, M., and Borriss, R. (2007). Tryptophan-dependent production of indole-3-acetic acid (IAA) affects level of plant growth promotion by Bacillus amyloliquefaciens FZB42. Mol. Plant Microbe. Interact. 20, 619–626. doi: 10.1094/MPMI-20-6-0619
Inada, S., and Shimmen, T. (2000). Regulation of elongation growth by gibberellin in root segments of Lemna minor. Plant Cell Physiol. 41, 932–939. doi: 10.1093/pcp/pcd018
Jackson, M. B. (2008). Ethylene-promoted elongation: an adaption to submergence stress. Ann. Bot. 101, 229–248. doi: 10.1093/aob/mcm237
Ishizawa, H., Kuroda, M., Morikawa, M., and Ike, M. (2017). Differential oxidative and antioxidative response of duckweed Lemna minor toward plant growth promoting/inhibiting bacteria. Plant Phys. Biochem. 118, 667–673. doi: 10.1016/j.plaphy.2017.08.006
Kinnersley, A. M., Scott, I. I. I., T. C, Yopp, J. H., and Whitten, G. H. (1990). Promotion of plant growth by polymers of lactic acid. Plant Growth Reg. 9, 137–146. doi: 10.1007/BF00027441
Landolt, E. (1957). Physiologishe und ökologische Untersuchungen an Lemnaceen. Ber. Schweiz. Bot. Ges. 67, 271–410.
Lu, Q., Zhang, T., Zhang, W., Su, C., Yang, Y., and Hu, D. (2018). Alleviation of cadmium toxicity in Lemna minor by exogenous salicylic acid. Ecotoxicol. Environ. Saf. 147, 500–508. doi: 10.1016/j.ecoenv.2017.09.015
Mader, J. C. (2004). Differential in vitro development of inflorescences in long and short day Lemna spp.: involvement of ethylene and polyamines. J. Plant Physiol. 161, 653–663. doi: 10.1078/0176-1617-01079
Majewsky, V., Scherr, C., Arlt, S. P., Kiener, J., Frrokaj, K., Schindler, T., Klocke, P., et al. (2014). Reproducibility of effects of homeopathically potentised gibberellic acid on the growth of Lemna gibba L. in a randomised and blinded bioassay. Homeopathy 103, 113–126. doi: 10.1016/j.homp.2013.12.004
Métraux, J. P., and Kende, H. (1983). The role of ethylene in the growth response of submerged deep water rice. Plant Physiol. 72, 441–446. doi: 10.1104/pp.72.2.441
Najeeb, U., Atwell, B. J., Gange, M. P., and Tan, D. K. Y. (2015). Aminoethoxyvinylglycine (AVG) ameliorates waterlogging-induced damage in cotton by inhibiting ethylene synthesis and sustaining photosynthetic capacity. Plant Growth Reg. 76, 83–98. doi: 10.1007/s10725-015-0037-y
Oota, Y., and Tsudzuki, T. (1971). Resemblance of growth substances to metal chelators with respect to their actions on duckweed growth. Plant Cell Physiol. 12, 619–631.
Pancheva, T. V., and Popova, L. P. (1998). Effect of the salicylic acid on the synthesis of ribulose-1,5-bisphosphate carboxylase/oxygenase in barley leaves. Plant Physiol. 152, 381–386. doi: 10.1016/S0176-1617(98)80251-4
Raskin, I., Skubazt, H., Tang, W., and Meeuse, B. J. D. (1990). Salicylic acid levels in thermogenic and non-thermogenic plants. Ann. Bot. 66, 376–373. doi: 10.1093/oxfordjournals.aob.a088037
Scherr, C., Simonc, M., Spranger, J., and Baumgartner, S. (2007). Duckweed (Lemna gibba L.) as a test organism for homeopathic potencies J. Alt. Comp. Med. 13, 931–937. doi: 10.1089/acm.2007.0506
Scherr, C., Simonc, M., Spranger, J., and Baumgartner, S. (2009). Effects of potentised substances on growth rate of the water plant Lemna gibba L. Comp. Ther. Med. 17, 63–70. doi: 10.1016/j.ctim.2008.10.004
Shimakawa, A., Shiraya, T., Ishizuka, Y., Wada, K. C., Mitsui, T., and Takeno, K. (2012). Salicylic acid is involved in the regulation of starvation stress-induced flowering in Lemna paucicostata. J. Plant Phys. 169, 987–991. doi: 10.1016/j.jplph.2012.02.009
Smart, C. C., and Trewavas, A. J. (1983). Abscisic-acid-induced turion germination in Spirodela polyrhiza L. I. Production and development of the turion. Plant Cell Environ. 6, 507–514.
Smalle, J., Haegman, M., Kurepa, J., Van Montagu, M., and Straeten, D. V. (1997). Ethylene can stimulate Arabidopsis hypocotyl elongation in the light. Proc. Natl. Acad. Sci. U.S.A. 94, 2756–2761. doi: 10.1073/pnas.94.6.2756
de Souza, I. R., and MacAdam, J. W. (2001). Gibberellic acid and dwarfism effects on the growth dynamics of B73 maize (Zea mays L.) leaf blades: a transient increase in apoplastic peroxidase activity precedes cessation of cell elongation. J. Exp. Bot. 52, 1673–1682.
Suzuki, W., Sugawara, M., Miwa, K., and Morikawa, M. (2014). Plant growth-promoting bacterium Acinetobacter calcoaceticus P23 increases the chlorophyll content of the monocot Lemna minor (duckweed) and the dicot Lactuca sativa (lettuce). J. Biosci. Bioeng. 118, 41–44. doi: 10.1016/j.jbiosc.2013.12.007
Takahashi, N., Kitamura, H., Kawarada, A., Seta, Y., Takai, M., Tamura, S., et al. (1955). Isolation of gibberellins and their properties. Bull. Agric. Chem. Soc. Jpn. 19, 267–277.
Toyama, T., Hanaoka, T., Tanaka, Y., Morikawa, M., and Mori, K. (2018). Comprehensive evaluation of nitrogen removal rate and biomass, ethanol, and methane production yields by combination of four major duckweeds and three types of wastewater effluent. Biores. Technol. 250, 464–473. doi: 10.1016/j.biortech.2017.11.054
Vanderstraeten, L., and Van Der Straeten, D. (2017). Accumulation and transport of 1-aminocyclopropane-1-carboxylic acid (ACC) in plants: current status, considerations for future research and agronomic applications. Front. Plant Sci. 8:38. doi: 10.3389/fpls.2017.00038
Rivas-San Vicente, M, and Plasencia, J. (2011). Salicylic acid beyond defence: its role in plant growth and development. J. Exp. Bot. 62, 3321–3338. doi: 10.1093/jxb/err031
Virtanen, E., Häggman, H., Degefu, Y., Välimaa, A. L., and Seppänen, M. (2013). Effects of production history and gibberellic acid on seed potatoes. J. Agric. Sci. 5, 145–153. doi: 10.5539/jas.v5n12p145
Xu, J., Cheng, J., and Stomp, A.-M. (2012). Growing Spirodela polyrhiza in swine wastewater for production of animal feed and fuel ethanol: a pilot study. Clean 40, 760–765. doi: 10.1002/clen.201100108
Yang, T., Law, D. M., and Davies, P. J. (1993). Magnitude and kinetics of stem elongation induced by exogenous indole-3-acetic acid in intact light-grown pea seedlings. Plant Pysiol. 102, 717–724. doi: 10.1104/pp.102.3.717
Keywords: Lemna minor, plant growth regulators, gibberellic acid, indole-3-acetic acid, salicylic acid, 1-aminocyclopropane-1-carboxylic acid, aminoethoxyvinylglycine, ascorbic acid
Citation: Utami D, Kawahata A, Sugawara M, Jog RN, Miwa K and Morikawa M (2018) Effect of Exogenous General Plant Growth Regulators on the Growth of the Duckweed Lemna minor. Front. Chem. 6:251. doi: 10.3389/fchem.2018.00251
Received: 19 February 2018; Accepted: 08 June 2018;
Published: 09 July 2018.
Edited by:
Klaus J. Appenroth, Friedrich-Schiller-Universität-Jena, GermanyReviewed by:
Hai Zhao, Chengdu Institute of Biology (CAS), ChinaElif Öztetik, Anadolu University, Turkey
Copyright © 2018 Utami, Kawahata, Sugawara, Jog, Miwa and Morikawa. This is an open-access article distributed under the terms of the Creative Commons Attribution License (CC BY). The use, distribution or reproduction in other forums is permitted, provided the original author(s) and the copyright owner(s) are credited and that the original publication in this journal is cited, in accordance with accepted academic practice. No use, distribution or reproduction is permitted which does not comply with these terms.
*Correspondence: Masaaki Morikawa, bW9yaWthd2FAZWVzLmhva3VkYWkuYWMuanA=
†Present Address: Desi Utami, Department of Agricultural Microbiology, Faculty of Agriculture, Universitas Gadjah Mada, Yogyakarta, Indonesia
Ami Kawahata, R&D Center, HAYASHIBARA Co., Ltd., Okayama, Japan
Masayuki Sugawara, Graduate School of Life Sciences, Tohoku University, Sendai, Japan