- 1Tianjin Key Laboratory of Electronic Materials and Devices, School of Electronics and Information Engineering, Hebei University of Technology, Tianjin, China
- 2Key Laboratory of Micro-Nano Electronics and Smart System of Zhejiang Province, Department of Information Science & Electronic Engineering, Zhejiang University, Hangzhou, China
WO3 nanosheets was prepared by an acidification method and the Rh catalyst was dispersed on the surface of the nanosheets with a wet impregnation method. The morphology of pristine WO3 and Rh modified WO3 nanosheets and their responses to acetone gas were studied. According to oxygen adsorption combined with TPR results, the sensing and sensitization mechanism of acetone were discussed. It was found that no visible changes in nanostructures or morphologies were observed in WO3 nanosheets with Rh, however, the sensor resistance and sensor response were greatly promoted. The basic sensitization mechanism could be caused by the electronic interaction between oxidized Rh and WO3 surface.
Introduction
Acetone gas is closely related to people with diabetes. Medical research has shown that there is a significant difference of acetone concentration in the breath for diabetics and healthy people, the former being higher than 1.8 ppm and the latter being below 0.8 ppm (Owen et al., 1982; Natale et al., 2014). Therefore, through the quantitative detection of the acetone concentration in human exhaled gases, it could achieve the purpose of diagnosis and monitoring to the disease condition. Metal oxide semiconductors (MOS) have been widely reported for gas sensors with the significant advantages, such as low cost, simple process and small size (Hübner et al., 2010; Choi et al., 2014). Tungsten trioxide (WO3) as an n-type semiconductor has become a research hotspot in the detection of VOC gases in recent years (Kanda and Maekawa, 2005; Kadir et al., 2015; Li et al., 2017). The adsorption and reaction of VOC gas on WO3 surface could change the semiconductor resistance, so the gas response can be improved by adding highly efficient catalytic elements. The introduction of ruthenium (Ru) and silicon (Si) improve the sensitivity of WO3 to acetone (Righettoni et al., 2010; Li et al., 2018). Further, Rh is known as a highly efficient catalyst to the catalytic reaction of acetone gas (Houtman and Barteau, 1991). It has been reported that Rh loaded SnO2 and In2O3 significantly improve the response of acetone (Kim et al., 2011; Kou et al., 2018). Therefore, this highly efficient catalyst could be also loaded onto WO3 surface to increase the response to acetone. In this study, the Rh element was uniformly loaded onto the surface of WO3 nanosheets based on an impregnation approach. This method has been frequently used in our previous work (Li et al., 2018). The experimental results show that the Rh nanoparticles can significantly enhance the response of WO3 nanosheets to acetone without changing the surface morphology of WO3 nanosheets. The basic sensitization mechanism of Rh was also analyzed based on experimental results.
Experimental
WO3 nanosheets was obtained by dropping Na2WO4 solution into H2SO4 solution (Kida et al., 2009). Aqueous solution of RhCl3 was impregnated with WO3 (Rh-WO3) powders and formed a suspension slurry, which was washed by distilled water and dried. Subsequently, the powders were annealed at 500°C in air. Sensor devices were made by the screen-printing technique. The crystal structures were measured by X-ray diffractometer (XRD; D8FOCUS, Germany). The morphology of sample was analyzed using scanning electron microscopy (FE-SEM; Nova Nano SEM 450, FEI, U. S). Nanosheets were also characterized by a transmission electron microscopy (TEM; Tecnai-F20, FEI, U.S). Energy spectrum analysis of materials uses X-ray photoelectron spectroscopy (XPS, Thermo escalab 250Xi, U. S). The catalyst activity was characterized by H2 temperature programmed reduction (H2-TPR; TP-5076, China). The experimental procedure of the TPR was descripted in Figure S1. Gas sensing tests were carried out by a conventional gas flow apparatus (see Figure S2). The gas sample was kept at a constant flow rate of 100 ml/min by mass flow controllers (MFC). The humidity of gas was <20 ppm and temperature of the chamber was about 50°C. The sensor response (S) was defined as S = Ra/Rg, where Ra and Rg are the sensor resistance in air and in the presence of target gases.
Results and Discussion
The morphology of WO3 nanosheets was characterized by SEM and TEM. Figures 1A,B show SEM images of pristine and 1wt.% Rh-WO3 nanosheets. One can see that the sample powders consisted of a large amount of nanoparticles with a lateral size from dozens to several hundred nanometers. According to SEM images, there were no visible changes observed in pristine WO3 and Rh modified one. For the results of specific surface area, pristine WO3 was ~12 m2/g and 1wt. %Rh-WO3 is about 13 m2/g, which indicts no significant change. Figure 2 shows TEM images of the pure WO3 and 1wt.%Rh-WO3 nanosheets. It was obvious that the sample powder is actually composed of highly irregular plate-like nanosheets. The insert image of Figure 2A presents a selected area diffraction (SAD) pattern of pristine WO3 nanosheets, suggesting that the nanosheets have a good crystal quality. In addition, some white particles with dozens of nm in size were observed in WO3 surface, as shown in Figure 2B. With the help of SAD in Figure 2C, these particles were identified as Rh2O3 with lattice spacing of 0.26 nm, corresponding to the (110) plane (JCPDS: 25-0707). It was thought that these large particles of Rh2O3 could be due to the aggregation of Rh during washing and drying process, which were not effectively removed during the washing process. While the lattice spacing of 0.38 nm in the HRTEM image was belong to monoclinic WO3 (JCPDS: 43-1035), which was in a good agreement of XRD results (in Figure S3).
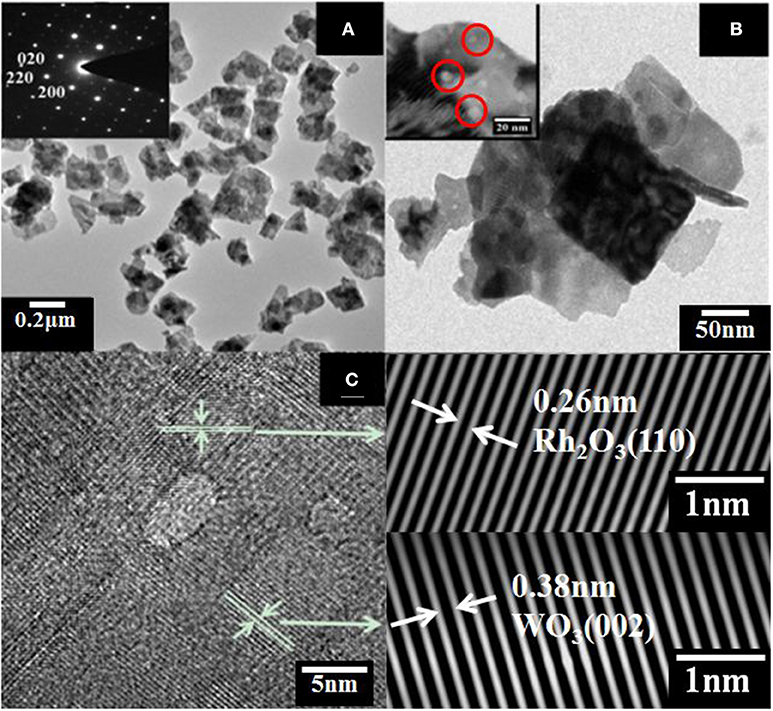
Figure 2. TEM images of (A) WO3 nanosheets insert with selected area diffraction (SAD) pattern, (B) 1wt%Rh-WO3 nanosheets, (C) HRTEM image of 1wt%Rh-WO3 nanosheets and SAD pattern of Rh2O3 and WO3.
The chemical state of Rh on WO3 surface was also analyzed by XPS. Figure 3A presents the XPS spectra of W, detection results of binding energy for W4f7/2 and W4f5/2 being 35.7eV and 37.9eV, respectively, which is in good agreement with W6+ (Dupin et al., 2000). Figure 3B shows the XPS spectra of Rh3d obtained from 1wt%Rh-WO3. Among them, the Rh2d5/2 peaked at 309.45eV is a typical oxide centered on Rh3+. In addition, the Rh2d3/2 located at 314.5eV is also an oxide centered on Rh3+ (Kim et al., 2011). Thus, it could be concluded that Rh was present as an oxidized state of Rh2O3 on WO3 surface. Additional with XPS results, the oxidized state of Rh could be also evidenced by H2-TPR. Figure 3C shows the H2-TPR results of pristine and 1wt%Rh-WO3 nanosheets. As expected, there was one weak peak around 370°C observed in pristine WO3 nanosheets indicating a weak consumption of H2, which may be due to the weak reduction behavior of WO3 surface at a high temperature (Li et al., 2018). In contrast, large consumptions of H2 were observed in 1wt%Rh-WO3, suggesting a strong reduction behavior. There were two overlapped peaks of H2 consumption at a low temperature around 110°C and the intensities of peaks were relatively high. It was believed that the consumption of H2 observed at low temperatures could be due to the reduction of Rh2O3 and peaks located different temperature may be ascribed to different dispersion states of Rh species. There was a broad but weak peak of H2 consumption at around 450°C, which could be attributable to the weak reduction of WO3 surface, i.e., surface lattice oxygen (OL) reacting with H2 at a high temperature. The reduction behavior of Rh-WO3 was much stronger than pristine WO3 indicating that the reactivity of lattice oxygens is slightly promoted by Rh2O3 on the surface. We can see significant differences, comparing this reduction behavior with our previous study of Pt-WO3 nanosheets (in Figure S4). At low temperature, Pt-WO3 produces a negative peak of H2 desorption. Based on the results of TPR and the resistance behavior under PO2, it is concluded that the main sensitization of Pt-WO3 may be caused by redox of Pt. nanoparticles (Li et al., 2017). This phenomenon of Rh may cause different sensitization mechanisms.
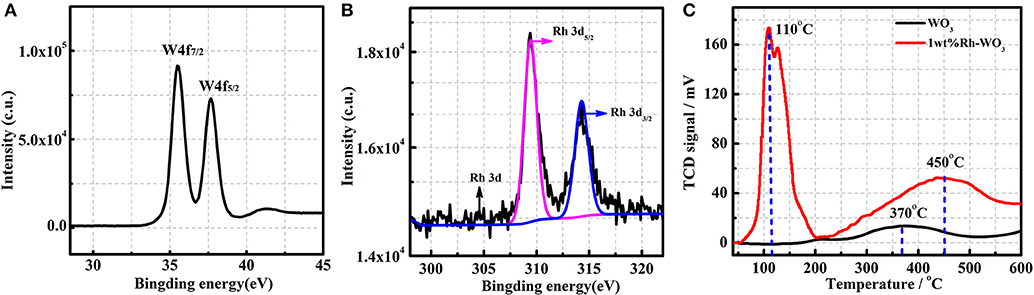
Figure 3. The XPS of 1wt%Rh-WO3: (A) W4f, (B) Rh3d, and (C) the H2-TPR patterns of pure WO3 and 1wt%Rh-WO3.
The sensing properties of pristineWO3 and Rh-WO3 nanosheets were characterized with acetone ranging from 0.5 to 10 ppm. Figure 4A shows the time dependence of sensor resistance. It was worth noting that the introduction of Rh greatly increased the sensor resistance of WO3. For 2wt.% Rh-WO3, the sensor resistance was almost three orders of pristine one. This indicated a strong electronic interaction between Rh2O3 and WO3 surface, forming the well-known P-N junction or fermi-level control sensitization mechanism. Due to the electronic junction of Rh2O3 with WO3, the sensors response and responding speed were significantly promoted. Figure 4B shows the calibration line of sensors resistance with concentration of acetone at an operation temperature of 250°C. It was found that sensor based on 1wt.%Rh-WO3 also responded to 0.5 ppm acetone. One can see that sensor response was increased by 3 times compared with the neat WO3. However, an excess of Rh did not effectively to promote the sensor response. This observation was in conflict with the great enhancement in sensor resistance. In order to explain the reduction in sensor response for 2wt.%Rh-WO3, there were two factors should be considered. Firstly, an excessive amount of Rh could lead to agglomeration of Rh2O3 and poor dispersion on the surface of WO3 nanoparticles. Consequently, some electronic interaction of Rh2O3 with WO3 leading to the high resistance were not effective to the sensitization. Secondly, with increasing the amount of Rh the surface activity of WO3 could be enhanced and then leaded to a catalytic reaction of acetone, which inhibit the diffusion of acetone molecule into inside of sensor films. As a result, the sensor response was reduced by a high loading amount of Rh. This reduction in sensor response could be also observed when increasing operation temperatures. This was evidenced by the strong dependence of sensor response on the operation temperatures for Rh-WO3 as shown in Figure S5a. It was thought that increasing the operating temperature leaded to an enhancement in catalytic activity, which reduces the gas diffusion and sensor response to acetone. When operating at a temperature larger than 250°C, one can note the sensor response greatly decreased with temperatures. It was also found that sensors resistance also obviously decreased with temperature, as shown in Figure S5b. For pristine WO3, the sensor response did not change significantly with temperature and exhibited a lower response at different temperatures. This poor response is associated with a weaker oxygen adsorption on WO3 surface (Zeng et al., 2017). At the same time, the stability of the sensor was also evaluated as shown in Figures S5c,d. It can be seen that Rh-WO3 nanoparticles can work for a long time at 350°C and has favorable response recovery performance.
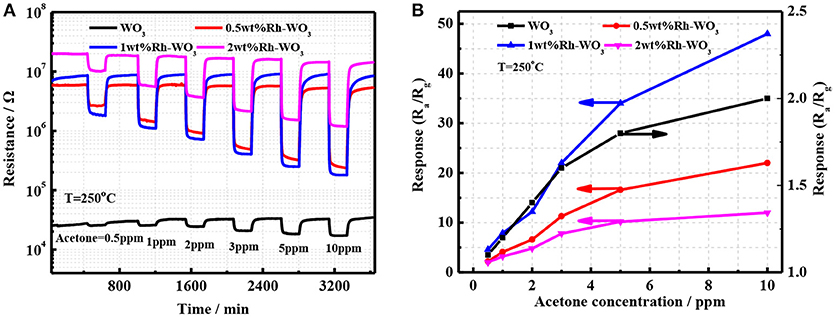
Figure 4. (A) The transient response of four different sensor devices and (B) the sensor response as a function of acetone concentration.
It is well-known that oxygen adsorption in the form of , O−, or O2− on the surface serves as the receptor function and determines the sensing ability and mechanism of MOS gas sensors (Hua et al., 2018a). In order to explore the sensitization effect of Rh-WO3 nanosheets, we analyzed the oxygen adsorption behavior. Figure 5A shows a linear plot of sensor resistance (Rg) with the partial pressure of oxygen (PO2) at a double logarithm-scale for pristine and 1wt.% Rh-WO3 sensors. It was observed that a linear relationship indicating a power-law response within all PO2 ranging from 0.06 to 0.99 atm (1 atm = 100% in volume) and the linear fitting coefficients were 0.42 and 0.62 for pristine and Rh-WO3, respectively. This indicated that the main type of oxygen adsorption was in the form of O− for both sensors (Hua et al., 2018a,b) at working temperature of 300°C through:
In case of 2 wt.% Rh-WO3, the linear plot of lnRg with lnPO2 was also valid. Remarkably, the slope, i.e., fitting coefficient was just 0.29, considerably <0.5. However, it was unlikely that a large amount of Rh on the surface could tailor the form of oxygen ionisorption on the surface. The most probably explanation was that with increasing PO2 the oxidized state of Rh, which has been limited to be exposed to atmosphere due to the aggregation of particles, was enhanced and then the electronic interaction between Rh2O3 and WO3 surface was promoted. Consequently, new depletion regions formed, leading to an increase in sensor resistance with PO2 and a reduction in the fitting coefficient. This has also been observed in our previous Pt-WO3 sensor (Li et al., 2017).
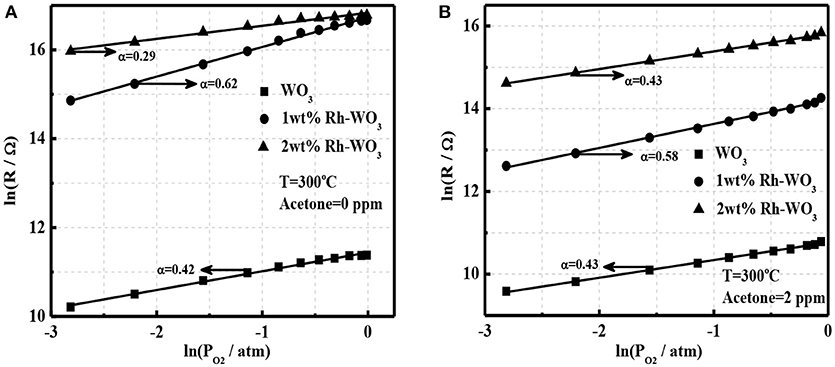
Figure 5. The power-law response to oxygen for pristine WO3 and Rh-WO3 (A) in the absence of acetone and (B) in the presence of 2 ppm acetone at 300°C.
According to our recent study, it was found that the power-law response of oxygen in the presence of reducing gas such as H2, CO, and acetone can be used to clarify the basic sensing mechanism of gas sensors (Hua et al., 2018c). Figure 5B shows the power-law response of oxygen in the presence of acetone (2 ppm) for pure and Rh-WO3 sensors. A very good linearity was observed for all sensors indicating that the basic sensing mechanism of acetone could be explained by the oxidation of acetone with oxygen adsorbates by:
For simplicity, it was assumed that acetone catalytic reaction was a complete reaction only producing CO2 and H2O. However, in fact the oxidation of acetone was rather complex. In addition, it was also found that linear coefficients of the power-law response were all around 0.5, which was consistent with Figure 5A and Equation (1). Importantly, for 2wt.%Rh-WO3, the fitting coefficient significantly raised up compared with that in the absence of acetone. This clearly supported our explanation for the degradation of sensitization effect with large loading amount of Rh and the reduction in the exponent of the power-law response to oxygen. In this respect, we believe that the basic sensitization mechanism of Rh on WO3 could be ascribed to the electronic interaction between Rh2O3 and WO3 (p-n junction), which was very similar with the fermi-level control model, popular for Pd-SnO2 sensors (Tang et al., 2015). The key factor to achieve a good sensitization effect highly relies on an elegant dispersion of Rh2O3 on WO3 surface, which can enhance the electronic interaction with WO3 surface as schematically drawn in Figure S6. This finding was similar with the case of Pt and Ru loaded WO3 nanosheets, however, it was significantly different with Pd and Fe loaded WO3. For the later one, the chemical sensitization effect of Pd and Fe plays a vital role through the reaction of surface lattice oxygens with reducing gases.
Conclusion
In summary, Rh as a noble catalyst was dispersed onto the surface of WO3 nanosheets through a wet impregnation method. Experimental results indicated that Rh was in a form of oxidized state Rh2O3 on WO3 surface and an excessive amount of Rh can lead to an aggregation of Rh2O3 and poor sensitization effect as well. An electronic interaction between Rh2O3 and WO3 surface was evidenced by an extremely high argument in sensor resistance and it was thought that such an electronic was responsible for the observed sensitization effect of Rh loading. To achieve a good sensitization effect, an elegant dispersion of Rh2O3 is required, which highly relies on an effective dispersion method and a proper loading amount. Additionally, a power-law response to oxygen was observed for both pristine and Rh-WO3 in the presence of acetone, which indicts that oxygen adsorption on the surface of WO3 serves as a basic receptor function.
Author Contributions
YL performed the experiments and analyzed the data with help from DH, CT, CZ, and XT. ZH, MW and EL conceived and guided the study. ZQ wrote the manuscript based on experimental data.
Funding
This study was supported by the National Natural Science Foundation of China (Grant NO. 61501167) and Natural Science Foundation of Tianjin (Grant NO. 15JCYBJC52100).
Conflict of Interest Statement
The authors declare that the research was conducted in the absence of any commercial or financial relationships that could be construed as a potential conflict of interest.
Supplementary Material
The Supplementary Material for this article can be found online at: https://www.frontiersin.org/articles/10.3389/fchem.2018.00385/full#supplementary-material
References
Choi, K. I., Hwang, S. J., Dai, Z., Kang, Y. C., and Lee, J. H. (2014). Rh-catalyzed WO3 with anomalous humidity dependence of gas sensing characteristics. RSC Adv. 4, 53130–53136. doi: 10.1039/C4RA06654E
Dupin, J. C., Gonbeau, D., Vinatier, P., and Levasseur, A. (2000). Systematic XPS studies of metal oxides, hydroxides and peroxides. Phys. Chem. Chem. Phys. 2, 1319–1324. doi: 10.1039/a908800h
Houtman, C., and Barteau, M. A. (1991). Adsorbed states of acetone and their reactions on rhodium(111) and rhodium(111)-(2.times.2)oxygen surfaces. J. Phys. Chem. 95, 3755–3764.
Hua, Z., Li, Y., Zeng, Y., and Wu, Y. (2018a). A theoretical investigation of the power-law response of metal oxide semiconductor gas sensors ?: schottky barrier control. Sens. Actuators B Chem. 255, 1911–1919. doi: 10.1016/j.snb.2017.08.206
Hua, Z., Qiu, Z., Li, Y., Zeng, Y., Wu, Y., Tian, X., et al. (2018b). A theoretical investigation of the power-law response of metal oxide semiconductor gas sensors ??: size and shape effects. Sens. Actuators B Chem. 255, 3541–3549. doi: 10.1016/j.snb.2017.09.189
Hua, Z., Tian, C., Huang, D., Yuan, W., Zhang, C., Tian, X., et al. (2018c). Power-law response of metal oxide semiconductor gas sensors to oxygen in presence of reducing gases. Sens. Actuators B Chem. 267, 510–518. doi: 10.1016/j.snb.2018.04.002
Hübner, M., Simion, C. E., Haensch, A., Barsan, N., and Weimar, U. (2010). CO sensing mechanism with WO3, based gas sensors. Sens. Actuators B Chem. 151, 103–106. doi: 10.3-106.10.1016/j.snb.2010.09.040
Kadir, R. A., Zhang, W., Wang, Y., Ou, J. Z., Wlodarski, W., O'Mullane, A. P., et al. (2015). Anodized nanoporous WO3 schottky contact structure for hydrogen and ethanol sensing. J. Mater. Chem. A 3, 7994–8001. doi: 10.1039/C4TA06286H
Kanda, K., and Maekawa, T. (2005). Development of a WO3, thick-film-based sensor for the detection of VOC. Sens. Actuators B Chem. 108, 97–101. doi: 10.1016/j.snb.2005.01.038
Kida, T., Nishiyama, A., Yuasa, M., Shimanoe, K., and Yamazoe, N. (2009). Highly sensitive NO2 sensors using lamellar-structured WO3 particles prepared by an acidification method. Sens. Actuators B Chem. 135, 568–574. doi: 10.1016/j.snb.2008.09.056
Kim, S. J., Hwang, I. S., Na, C. W., Kim, I. D., Kang, Y. C., and Lee, J. H. (2011). Ultrasensitive and selective C2H5OH sensors using Rh-loaded In2O3 hollow spheres. J. Mater. Chem. 21, 18560–18567. doi: 10.1039/C1JM14252F
Kou, X., Xie, N., Chen, F., Wang, T., Guo, L., Wang, C., et al. (2018). Superior acetone gas sensor based on electrospun SnO2 nanofibers by Rh doping. Sens. Actuators B Chem. 256, 861–869. doi: 10.1016/j.snb.2017.10.011
Li, Y., Hua, Z., Wu, Y., Zeng, Y., Qiu, Z., and Tian, X., et al. (2017). Surface modification of Pt-loaded WO3 nanosheets for acetone sensing application. Chem. Lett. 47, 167–170. doi: 10.1246/cl.170990
Li, Y., Hua, Z., Wu, Y., Zeng, Y., Qiu, Z., and Tian, X., et al. (2018). Modified impregnation synthesis of Ru-loaded WO3, nanoparticles for acetone sensing. Sens. Actuators B Chem. 265, 249–256. doi: 10.1016/j.snb.2018.03.037
Natale, C. D., Paolesse, R., Martinelli, E., and Capuano, R. (2014). Solid-state gas sensors for breath analysis: a review. Anal. Chim. Acta 824, 1–17. doi: 10.1016/j.aca.2014.03.014
Owen, O. E., Trapp, V. E., Skutches, C. L., Mozzoli, M. A., Hoeldtke, R. D., and Boden, G. A. (1982). Acetone metabolism during diabetic ketoacidosis. Diabetes 31, 242–248. doi: 10.2337/diabetes.31.3.242
Righettoni, M., Tricoli, A., and Pratsinis, S. E. (2010). Si: WO3 sensors for highly selective detection of acetone for easy diagnosis of diabetes by breath analysis. Anal. Chem. 82, 3581–3587. doi: 10.1021/ac902695n
Tang, W., Wang, J., Qiao, Q., Liu, Z., and Li, X. (2015). Mechanism for acetone sensing property of Pd-loaded SnO2, nanofibers prepared by electrospinning: fermi-level effects. J. Mater. Sci. 50, 2605–2615. doi: 10.1007/s10853-015-8836-0
Keywords: WO3, Rh, acetone, surface modification, gas sensors
Citation: Qiu Z, Hua Z, Li Y, Wang M, Huang D, Tian C, Zhang C, Tian X and Li E (2018) Acetone Sensing Properties and Mechanism of Rh-Loaded WO3 Nanosheets Front. Chem. 6:385. doi: 10.3389/fchem.2018.00385
Received: 12 July 2018; Accepted: 09 August 2018;
Published: 11 September 2018.
Edited by:
Wen Zeng, Chongqing University, ChinaReviewed by:
Dachi Yang, Nankai University, ChinaGuotao Duan, Institute of Solid State Physics, Hefei Institutes of Physical Science (CAS), China
Copyright © 2018 Qiu, Hua, Li, Wang, Huang, Tian, Zhang, Tian and Li. This is an open-access article distributed under the terms of the Creative Commons Attribution License (CC BY). The use, distribution or reproduction in other forums is permitted, provided the original author(s) and the copyright owner(s) are credited and that the original publication in this journal is cited, in accordance with accepted academic practice. No use, distribution or reproduction is permitted which does not comply with these terms.
*Correspondence: Zhongqiu Hua, emhvbmdxaXVodWFAaGVidXQuZWR1LmNu
Mengjun Wang, d2FuZ21lbmdqdW5AaGVidXQuZWR1LmNu