- 1Chair of Aroma and Smell Research, Department of Chemistry and Pharmacy, Emil Fischer Center, Friedrich-Alexander-Universität Erlangen-Nürnberg (FAU), Erlangen, Germany
- 2Department Sensory Analytics, Fraunhofer Institute for Process Engineering and Packaging (IVV), Freising, Germany
Ramson (Allium ursinum) is known for its typical garlic-like aroma. Both ramson and garlic belong to the genus allium which is characterized by a high content of sulfurous compounds. However, in contrast to garlic, ramson is in general not associated with an unpleasant breath following consumption. While there is data available regarding the metabolism of volatile garlic constituents in the human body, the metabolism of ramson was not yet addressed. To elucidate if ramson has an impact on the body odor, this study aimed at identifying volatile ramson-derived metabolites in human milk and urine. Therefore, milk and urine samples were gathered before and after ramson consumption, and were analyzed sensorially by a trained human sensory panel as well as chemo-analytically applying gas chromatography-mass spectrometry/olfactometry (GC-MS/O). Sensory evaluation revealed a garlic-/cabbage like odor in milk samples obtained after ramson consumption, demonstrating that ramson consumption affected the milk aroma. Analyzes by means of GC-MS/O further confirmed excretion of three ramson-derived metabolites in milk and urine samples collected after ramson consumption, namely allyl methyl sulfide (AMS), allyl methyl sulfoxide (AMSO) and allyl methyl sulfone (AMSO2). Of these metabolites only AMS had a garlic-/cabbage-like odor, while the other two were odorless. These metabolites were subsequently quantified using stable isotope dilution assays. Nine urine sets, each comprising eight urine samples, and nine milk sets, each comprising four samples, were analyzed. In case of the urine sets a time interval of about 24 h was monitored, in case of the milk sets a time interval of up to 9 h. Despite the fact that all samples contained the same metabolites there were relevant differences found between individual subjects, especially with regard to the temporal rate of metabolite excretion. Generally, the maxima of metabolite excretion were observed in milk sets within 3 h after ramson consumption. In urine the highest AMS and AMSO amounts were observed within 2 h whereas the maximum concentration of AMSO2 was reached about 2 to 4 h after ramson ingestion. This study suggests that ramson constituents are heavily metabolized in the human body.
Introduction
Spice plants and condiments such as garlic are used world-wide to modulate the taste of dishes. However, this is associated with the side-effect that some people emit a distinct smell after consumption of garlic. The typical aroma of garlic is due to its pronounced content of sulfur-containing compounds. In general, a high sulfur substance content is typical for the genus Allium, which includes garlic. However, another representative of the genus Allium is ramson (Allium ursinum) which is commonly not associated with aversive effects like unpleasant breath despite the fact that the volatile oil of ramson comprises compounds that have also been identified in garlic, namely sulfides, ajoenes, and dithiins (Sendl et al., 1992; Benkeblia and Lanzotti, 2007; Godevac et al., 2008; Sabha et al., 2012). In relation to body odor and excretion processes of ramson-derived substances, biotransformation processes in the body need to be considered as these processes might lead to neoformation of compounds, whereas others might be degraded. Potential flavor effects of human nutrition on the sensory properties of human milk are in this context of special interest as human milk is commonly the sole food source of a newborn, at least during the first months of life. Besides possible effects on the infants' health, both positive and negative, it has been reported that the maternal diet can influence the suckling behavior and later dietary habits of the infant (Mennella et al., 2001). These behavioral effects have been attributed to a changed flavor profile of the milk, implicating that these flavor changes were supposed to be attractive to the children. However, final evidence in terms of sensory and chemo-analytical data, as well as proof of attractiveness of such flavor impressions to the infants was not provided. In this context it is interesting to note that intake of specific foods might impact diverse physiological processes and parameters such as blood pressure and secretory processes. There are several studies which demonstrate that the consumption of garlic can lead to reduction in blood pressure which is particularly distinctive in case of hypertensive patients (Reinhart et al., 2008; Ried, 2016; Choudhary et al., 2017). Such processes might also impact lactation processes, e.g., with elevated milk secretion, which might directly impact suckling behavior and milk intake of the children.
On the other hand, the analysis of urine opens the possibility to investigate low-molecular weight compounds. The dietary influence on the urine composition was demonstrated for instance for asparagus: people who consumed asparagus excreted urine with a sulfurous odor (Pelchat et al., 2011). Furthermore, the odorous compounds in urine have also been used as indicators for diseases or metabolic syndromes, e.g., trimethylaminuria, in which case the persons concerned excreted increased concentrations of trimethylamine via urine leading to a fishy odor (Humbert et al., 1970).
In case of garlic ingestion our group already identified three metabolites excreted via human milk and urine, namely allyl methyl sulfide (AMS), allyl methyl sulfoxide (AMSO) and allyl methyl sulfone (AMSO2) with only AMS present in garlic itself whereas the other two compounds are only formed within the human body (Scheffler et al., 2016a,b). In case of ramson there is no data available concerning its metabolism within the human body. To close this gap the present study aimed at elucidating volatile ramson-derived metabolites in humans. Therefore, human milk and urine samples gathered before and after ramson consumption were analyzed. Additionally, the identified compounds were quantified and their temporal excretion was monitored over a time period of about 9 h (milk) or 24 h (urine). The main consideration during all investigations was thereby to obey a consumption protocol that is well in line with a real-life ramson consumption scenario.
Materials and Methods
The materials and methods that were used in this study are in most parts in line with those described in our previous studies which addressed the identification and quantification of garlic-derived metabolites in human milk and urine (Scheffler et al., 2016a,b; Scheffler et al., submitted). For this reason only a short overview is provided here. Detailed descriptions are given in the respective studies and in the Supplementary Material provided for this study.
Study Design and Ethics Approval
The study was conducted in agreement with the Declaration of Helsinki. All participants gave written, informed consent prior to the testing day. They were able to withdraw from the study at any time. The study (registration no. 163_16 Bc) was approved by the Ethical Committee of the Medical Faculty, Friedrich-Alexander-Universität Erlangen-Nürnberg.
Human Milk Samples
Human milk samples were obtained from 13 different mothers (age range 27–39 years, mean 33). The volunteers had no known illnesses and their milk production exceeded their infants' need. The sampling took place 9 to 37 seven weeks postpartum (mean 19 weeks). To avoid sulfurous compounds in the milk samples that were not associated with ramson consumption, the test persons were asked to avoid food containing high amounts of sulfur substances (e.g., garlic, onion, ramson, chives, cabbage, and leek) on the testing day and 2 preceding days. Additionally, they were asked to keep record of their food during these 3 testing days. Each mother provided one milk sample before ramson consumption and three further samples after ramson ingestion. The samples were collected according to the normal lactation period of each mother and immediately analyzed. Four consecutive milk samples formed a milk set in each case. In a pre-test one mother consumed about 3 g of ramson. All other test persons consumed about 10 g of ramson.
Four milk sets were collected for identification experiments. They were labeled “M” with Arabic numerals, e.g., M 2-1 to M 2-4. Quantification experiments were performed after completion of identification experiments. In case of the quantification experiments nine milk sets were gathered and labeled “M” with Roman numerals, e.g., M II-1 to M II-4.
Human Urine Samples
Human urine samples were obtained from four volunteers (age 24–28 years, mean 26, two females, two males). One volunteer conducted the whole experimental series four times, the other three volunteers participated twice. Urine samples were collected in sterile brown glass bottles. At the testing day as well as 2 days preceding the testing day, the test persons were instructed to avoid sulfurous compounds and to record consumed foods and beverages as described for the milk sampling (cf. chapter 2.2). On the testing day ramson was freshly washed, ground and aliquoted into portions of 10 g. The volunteers provided one urine sample before and seven samples after ingestion of 10 g of ramson at about the following times: 0.5, 1, 2, 4, 6, 8, and 24 h after ramson consumption. Eight consecutive urine samples comprised one urine set. The samples were kept frozen at −80°C until further analysis.
To rule out illnesses of the test persons, the first sample of each set was tested with a dipstick test. With the multiple test stripes (Combi-Screen Plus, Analyticon Biotechnologies AG, Lichtenfels, Germany) simultaneous testing of following urine parameters was possible: ascorbic acid, bilirubin, blood, glucose, ketones, leucocytes, nitrite, pH, protein, specific gravity/density, and urobilinogen.
For identification experiments one urine set was collected. The respective samples were labeled U 1-1 to U 1-8. For quantification analyses nine urine sets were analyzed. The urine samples of each set were labeled “U” with Roman numerals (e.g., U II-1 to U II-8) and according to their sampling time:
- Pre: 3 to 7 min before ramson consumption
- 0.5 h post: 0.45 h to 0.55 h after ramson consumption
- 1 h post: 1.00 h to 1.05 h after ramson consumption
- 2 h post: 1.95 h to 2.10 h after ramson consumption
- 4 h post: 4.00 h to 4.10 h after ramson consumption
- 6 h post: 6.00 h to 6.10 h after ramson consumption
- 8 h post: 8.00 h to 8.15 h after ramson consumption
- 24 h post: 23.95 h to 24.20 h after ramson consumption
U II to U V were obtained at the same testing day with each test person consuming ramson portions of about 10 g. Likewise, U VI to U IX were provided at the same testing days.
Isolation, Identification and Quantification of Ramson-Derived Metabolites
The volatile fraction of the urine and milk samples was isolated by means of Solvent-assisted flavor evaporation (SAFE)-distillation (Engel et al., 1999). The obtained distillate was analyzed by means of high resolution gas chromatography-olfactometry (HRGC-O) as well as high resolution gas chromatography-mass spectrometry (HRGC-MS) and two-dimensional HRGC-MS/O (heart-cut). Ramson-derived metabolites were identified by comparing their retention indices (RI) of two analytical capillaries with different polarities (DB-5 and DB-FFAP), their respective odor as well as their mass spectra with those of reference standards. RI values were calculated according to van Den Dool and Kratz (1963). A relative concentration of the identified metabolites was calculated by normalizing respective peak areas to the amount of investigated milk or urine (in kg) in order to express the concentration in units of area/kg milk or urine. In case of the urine samples, the concentration was additionally expressed as area/mmol creatinine. Therefore, the creatinine levels in each urine sample were determined using a creatinine kit (Labor+Technik Eberhard Lehmann GmbH, Berlin, Germany). The peak area was divided by the amount of investigated urine (in L) and the creatinine content (in mmol/L) of the respective urine sample. In subsequent studies the respective metabolites were quantified by means of stable isotope dilution analysis (SIDA). Isotopically labeled standards were added to the samples prior to sample work up and solutions consisting of defined mixtures of analyte standards and their respective isotopically labeled standards were analyzed. Calibration curves were calculated as functions between the intensity ratios of standard to labeled standard and their respective mass ratios (cf. Table 1). Calibration curves were prepared in triplicates, and at 3 different days. For quantification the average of these calibration curves was used. With the resulting calibration function, the known amount of isotopic labeled standard added to the sample and the intensity ratio of analyte to isotopic labeled standard the amount of the analyte in the sample was calculated.
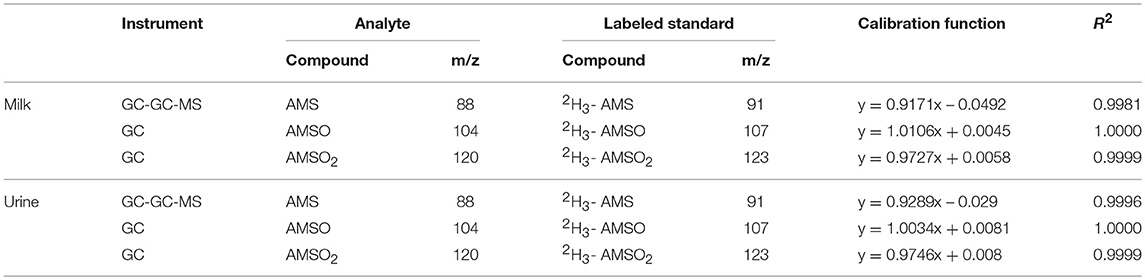
Table 1. Parameters for quantification including the instrument which was used for measurement, selected m/z-ratios of analytes and isotopically labeled standards and calibration factors.
In order to express the concentration of ramson-derived metabolites as μg/kg milk or urine or μg/mmol creatinine, the calculated amounts of AMS, AMSO and AMSO2 were divided by the amount of investigated sample (in kg) or normalized to creatinine content as described above.
Aroma Profile Analysis of Human Milk Samples
Milk samples were additionally evaluated sensorially (orthonasally) prior to the sample work up. Aroma profile analysis was performed based on sensory pre-evaluations and the intensity of the following attributes were rated on a scale from 0 (no perception) to 3 (strong perception): hay-like, fishy, fatty, rancid, sweaty, metallic, grassy-green, sweet, egg white-like, buttery, lactic, and garlic/cabbage-like. The evaluated attributes corresponded to those described in Scheffler et al. (2016b).
Results
Aroma Profile Analysis of Human Milk Samples in Relation to Ramson Ingestion
Aroma profile analyses were performed on all human milk samples that had been obtained before and after ramson consumption in the course of identification experiments. Samples were rated according to the attributes fishy, fatty, metallic, grassy-green, rancid, sweaty, buttery, sweet, hay-like, egg white-like, lactic, and garlic-/cabbage-like. The overall intensity of the milk samples as well as the odor attributes were mostly rated as being just detectable (intensity 1) or even not perceivable (intensity 0). Overall, the attribute garlic-/cabbage-like was not perceivable in all samples obtained before ramson intervention. After consumption of 10 g of fresh ramson the milk samples were rated as having a slight (0.5–1.0) garlic-/cabbage-like odor by most of the panelists. The odor change was most pronounced in milk samples that were provided 2 to 5 h after ramson consumption. Exemplary aroma profiles of a sample series of one milk set are displayed in Figure 1. Nevertheless, this does not rule out a potential sensory detection via tasting that was, however, not possible due to work safety considerations. All other aroma profiles of the milk samples that were also collected for the identification experiments are provided in Figure S1 of the Supplementary Material. Moreover, aroma profile analyses were repeated for those samples that were collected during the quantification experiments. Also in these cases a slight garlic-/cabbage-like odor was perceived in the milk samples that were obtained after ramson consumption (data not shown). Hence, the results from the identification experiments could be confirmed.
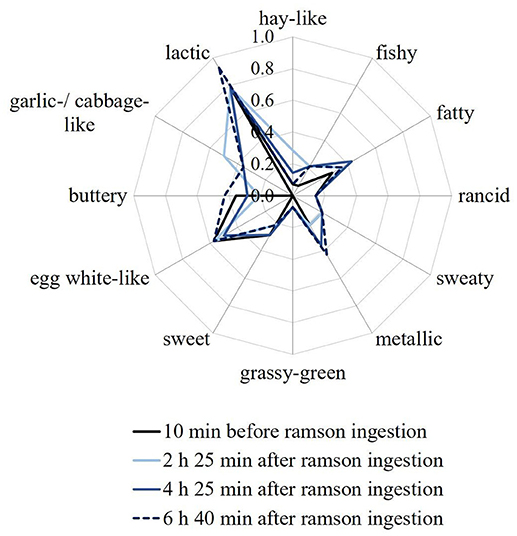
Figure 1. Odor profiles of human milk samples of set M 2, as a representative sample. The samples were collected at different times before and after ingestion of 10 g of ramson: 10 min before, 2 h 25 min after, 4 h 25 min after and 6 h 40 min after ramson intervention. Panelists were asked to rate the orthonasal perception on a scale from 0 (no perception) to 3 (strong perception). Values are mean ratings of all panelists. Note: The scale is only presented up to the value of 1 for better visualization.
Identification of Ramson-Derived Metabolites
Comparative GC-O Analysis
Comparative GC-O analyses were performed for the corresponding human milk and urine samples collected before and after ramson consumption in order to identify odor-active compounds that derived from ramson consumption. In both, urine and milk samples, the analyses revealed one additional odor-active substance that was only detectable in samples obtained after ramson intervention. This substance had a garlic-/cabbage-like odor, a RI < 1000 on the FFAP capillary and RI 702 on the DB-5 capillary. By comparison of the odor quality and the respective RI-values with reference substances (cf. Table 2), this compound was identified as AMS. All other odors were perceived in all samples, irrespective of ramson consumption, hence they cannot be associated with ramson consumption but rather contribute to the typical smell of human milk and urine.
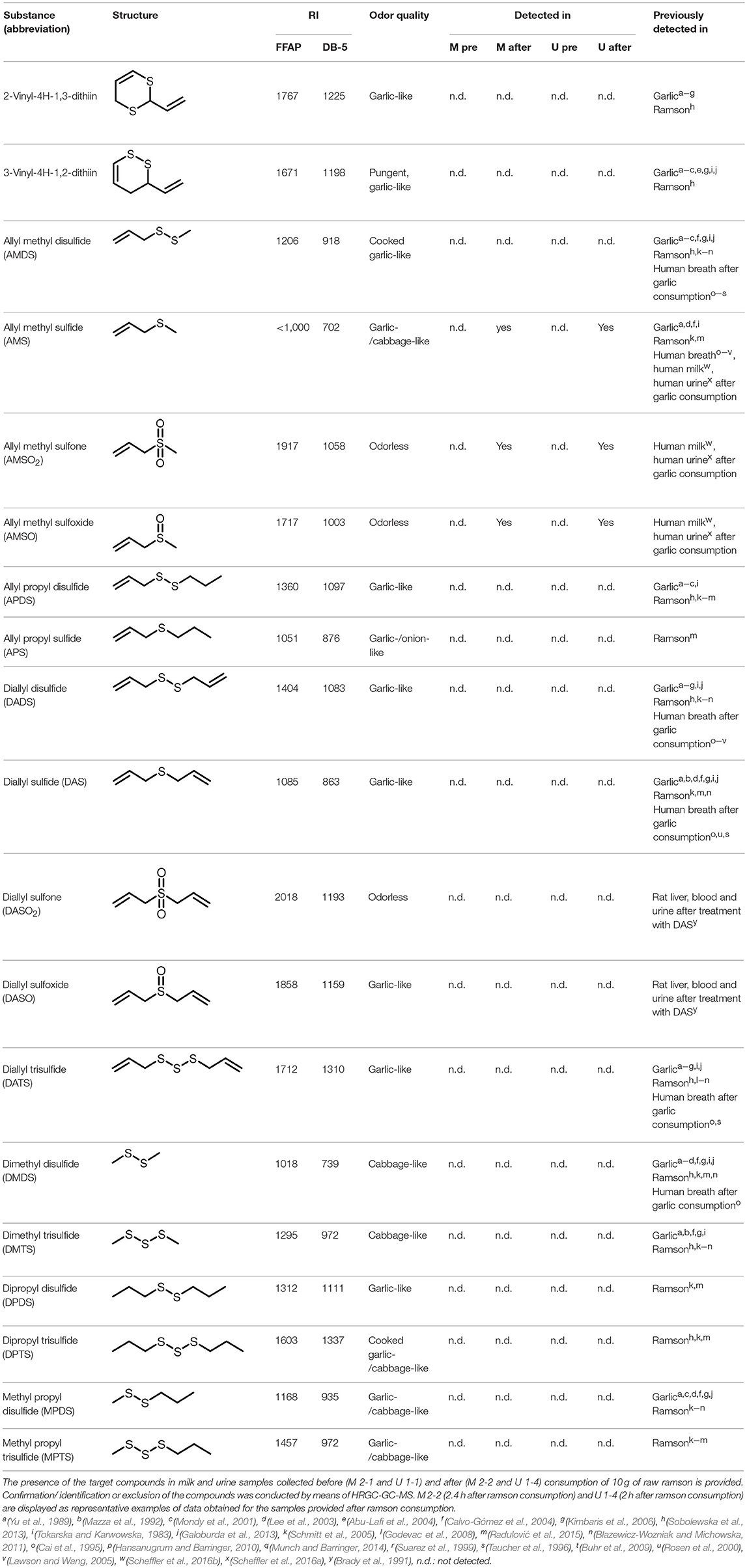
Table 2. Compilation of investigated substances, their chemical structures, retention indices (RI) on two different chromatographic capillaries (DB-FFAP and DB-5), their odor qualities and literature reports on these substances.
Identification of Ramson-Derived Volatiles in Milk and Urine Samples Applying HRGC-MS and HRGC-GC-MS
In order to further identify less odor-active or odorless ramson-derived compounds in the milk and urine samples, the chromatograms obtained from GC-MS analyses of control samples prior to ramson intervention were compared to those obtained after intervention in full scan-mode. Additionally, the samples were screened for potential ramson-derived metabolites via targeted search, taking into consideration compounds that had previously been reported in literature as constituents of ramson. Moreover, we screened for substances that had been reported in garlic, or in breath after garlic consumption, respectively, or that had been proposed as potential metabolites in humans based on animal studies (cf. Table 2). To this aim, a targeted search by means of GC-MS and GC-GC-MS was conducted utilizing standard solutions of the respective compounds. GC-GC-MS analyses thereby allowed screening for minor quantities of the target substances in the low μg/mL-range. The concentrations of the respective reference compounds thereby ranged between 0.5 and 12.7 μg/mL DCM. These investigations revealed two additional ramson-derived metabolites, namely AMSO and AMSO2. Moreover, GC-GC-MS analysis confirmed the identification of AMS in urine and milk samples collected after ramson consumption. Representative chromatograms are displayed in Figure 2. The other compounds of the targeted search were not detectable in any urine or milk samples, even at concentrations as low as about 1–10 μg/mL, irrespective if sampling took place prior to or after ramson ingestion. These compounds were: 2-vinyl-4H-1,3-dithiin, 3-vinyl-4H-1,2-dithiin, allyl methyl disulfide (AMDS), allyl propyl disulfide (APDS), allyl propyl (APS), diallyl disulfide (DADS), diallyl sulfide (DAS), diallyl sulfoxide (DASO2), diallyl sulfone (DASO), diallyl trisulfide (DATS), dimethyl disulfide (DMDS), dimethyl trisulfide (DMTS), dipropyl disulfide (DPDS), dipropyl trisulfide (DPTS), methyl propyl disulfide (MPDS), and methyl propyl sulfide (MPTS).
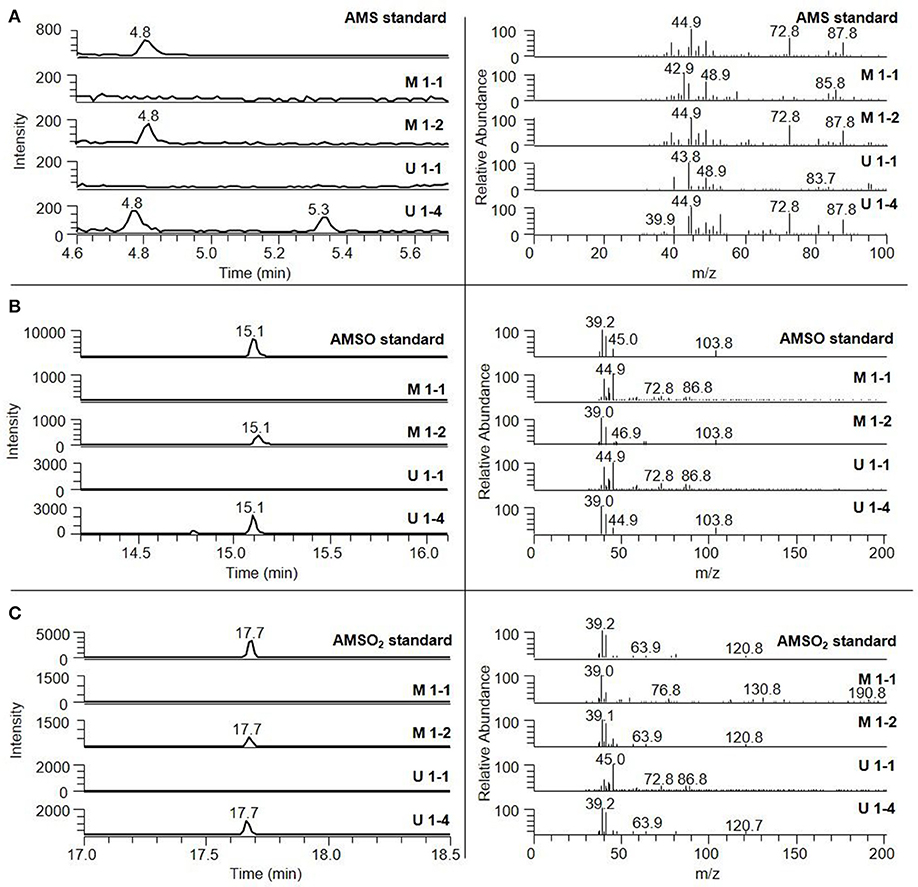
Figure 2. Identification of ramson-derived components in human milk and human urine. The milk samples were collected 10 min before (M 2-1) and 2 h 25 min (M 2-2) after ramson consumption. Urine samples were gathered 5 min before (U 1-1) and 2 h (U 1-4) after ramson consumption. (A) AMS, (B) AMSO, (C) AMSO2. The respective mass spectra are shown on the right side; they correspond to the elution time of the respective standard compound.
Quantification and Time Dependency of Excretion of Ramson-Derived Metabolites
Quantitative Analysis of Ramson-Derived Metabolites in Human Milk
The quantification of ramson-derived metabolites in human milk was carried out on nine milk sets, each comprising four milk samples. The exact sampling times as well as volume and weight of the samples are provided in Table S1.
In the majority of cases the ramson-derived metabolites AMS, AMSO, and AMSO2 were only detected in those milk samples that were obtained after ramson consumption. Only in case of milk set V and VI small amounts of these metabolites were observed in the samples gathered prior to ramson consumption. According to the recorded food protocols, the respective test persons consumed bratwurst and kohlrabi 1 day prior to the respective testing days. Bratwurst can comprise multiple spices, amongst others garlic. Garlic, as well as kohlrabi are rich in sulfur-containing compounds, which might explain the detected metabolites in the samples collected prior to ramson consumption. Despite these small amounts in the control samples, distinct increases in the metabolite concentrations were observed in the subsequently collected milk samples. The highest observed AMS concentrations in milk samples obtained after ramson consumption were found to be quite consistent, with values in the range between 1.7 and 2.0 μg/kg milk. AMSO and AMSO2 reached values between 38.4 and 89.6 μg/kg milk and 53.0 and 98.6 μg/kg milk, respectively. Commonly, the maxima were either detected in the first or in the second milk sample after ramson consumption which corresponds to a time interval between 1.5 to 5 h after ramson ingestion. Thereby, the AMSO maximum tended to appear slightly earlier than the AMSO2 maximum. In most cases the highest concentrations of the metabolites AMS and AMSO were reached within 3 h after ramson consumption (as was the case for seven out of nine milk sets). The highest amount of AMSO2 was mostly observed between 3 and 5 h (as was the case for six out of nine milk sets). Afterwards, a decline in metabolite concentration was observed which was pronounced in case of AMSO but slow in case of AMSO2. This trend is visualized in Figure 3.
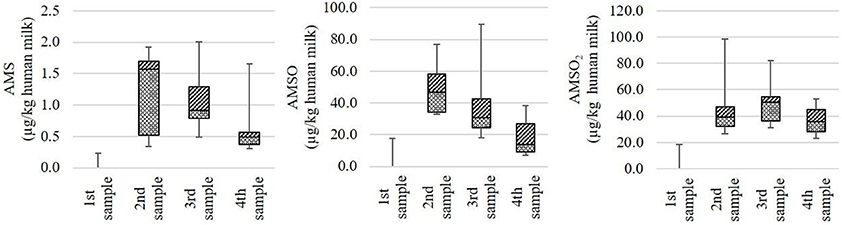
Figure 3. Box plot (mean value, markers at minimum and maximum metabolite concentration, box perc. 25–75%) of concentrations of ramson metabolites (AMS, AMSO, and AMSO2) in human milk, expressed as μg/kg human milk. Data comprise nine milk sets each being composed of four milk samples: one sample was collected before (“1st sample”) and three samples after (“2nd sample” to “4th sample”) ramson ingestion.
The metabolite concentrations for each milk sample, arranged according to milk sets, are provided in Table S1.
The temporal monitoring of the excreted metabolite concentrations revealed some inter-individual variation between test persons. For better visualization, representative time-resolved metabolite profiles are displayed in Figure 4.
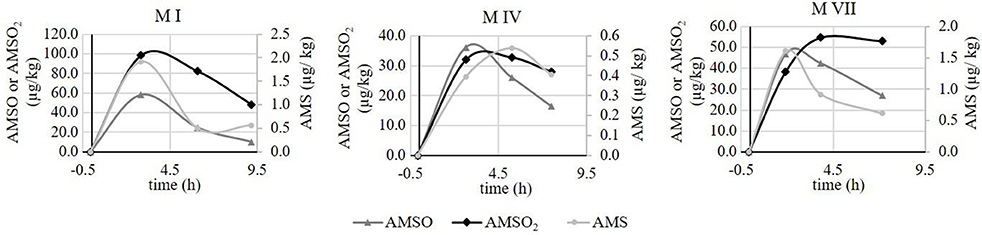
Figure 4. Time-resolved metabolite profiles of AMS, AMSO and AMSO2. Profiles are exemplarily shown for milk sets M I, M IV, and M VII. • AMS,▴ AMSO, ♦ AMSO2. Time 0 h corresponds to the time of ramson consumption.
Generally, differences were observed both in the excreted metabolite concentrations, as described above, as well as in the time dependency of excretion. It is important to note that, however, all test persons consumed the same amount of fresh ramson following the same consumption protocol. The time-resolved metabolite profiles revealed that for some milk samples the maxima of all three metabolites coincided in the first milk sample gathered after ramson consumption (e.g., M I). On the other hand, there were also milk sets where the maximum of AMSO2 was detected after the maximum of AMSO (e.g., M VII), and other cases, where the maximum of AMS appeared in the milk sample following the maximum of AMSO (e.g., M IV). To further clarify whether the maxima of the metabolites coincide or are excreted with a time delay, a larger sample set should be analyzed with shorter time intervals between the samples. However, the present study aimed to investigate the concentrations of ramson-derived metabolites in human milk in a real-life situation which included that the mothers kept their normal feeding habits.
Quantitative Analysis of Ramson-Derived Metabolites in Human Urine
The quantification of ramson-derived metabolites in human urine was likewise carried out on nine different urine sets, each comprising eight urine samples. The exact sampling times as well as volume, weight and creatinine content of each sample are provided in Table S2.
The metabolites AMS, AMSO, and AMSO2 were only detected in urine samples gathered after ramson consumption with the sole exception of set U IX. In this urine set small amounts of the respective metabolites were detected in the sample collected before ramson intervention. According to the food protocol the respective test person consumed chicken escalope with mustard the day prior to the experiment. Mustard is rich in glucosinolates (Belitz et al., 2008), compounds that are sulfur-containing and could therefore be responsible for the respective derivatives in the first urine sample. Although the presence of AMS, AMSO, and AMSO2 could not be excluded in this case, distinct increases were observed after ramson consumption. Likewise, AMS, AMSO, and AMSO2 showed a rapid onset in all other urine sets after ramson consumption. The maximum AMS concentrations in the respective urine sets were found to be in the range between 0.4 and 0.9 μg/kg urine, whereas the maximum AMSO and AMSO2 concentrations ranged between 46.3 and 81.2 μg/kg urine and 40.8 and 87.1 μg/kg urine, respectively. However, relative metabolite concentrations in urine strongly depend on the water intake of the test persons. This discontinuous variability can be taken account of by normalizing the metabolite concentrations to the creatinine content of the respective urine sample. As a result, urine samples within one urine set as well as urine sets from different test persons can be compared with each other, and allow a better comparison of the temporal excretion processes in different subjects. Overall, the highest normalized AMS and AMSO concentrations were observed between 1 and 2 h after ramson consumption, and varied, in case of AMS, between 0.3 and 0.7 μg/mmol creatinine, and, in case of AMSO between 32.9 and 106.9 μg/mmol creatinine. The highest amounts of AMSO2 in urine generally varied between 27.5 and 97.7 μg/mmol creatinine. The AMSO2 maximum was either detected temporally coinciding with the AMSO maximum (as was the case for four urine sets) or with a temporal delay of about 1 to 2 h (as was the case for five urine sets). In most cases the AMSO2 maxima were observed about 2 h after ramson consumption. Only in case of U IX, a maximum was observed at 1 h, and in case of U VII at 4 h after ramson consumption. A high concentration of AMSO2 commonly correlated with high amount of AMSO and AMS in either case. In some urine sets a second increase in metabolite concentration could be observed about 6 to 8 h after ramson consumption (e.g., U III and U V). However, this second increase was not as distinct as the first one, commonly reaching about 10–70% of the total intensity of the first maximum. In Figure 5 the quantitative analyses of all urine samples are summarized as a box plot diagram. The metabolite concentrations for each urine sample, arranged according to urine sets, are additionally provided in Table S2.
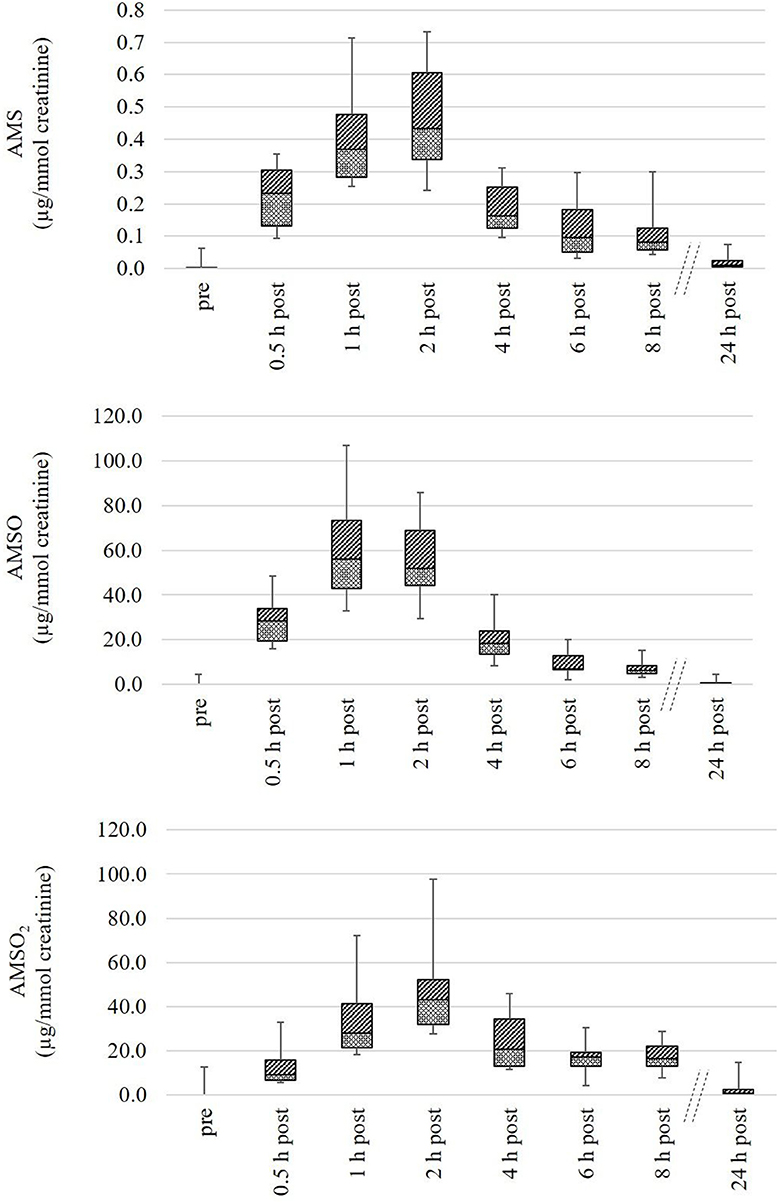
Figure 5. Box plot (mean value, markers at minimum and maximum metabolite concentration, box perc. 25–75%) of concentrations of ramson metabolites (AMS, AMSO, and AMSO2) in human urine, expressed as μg/mmol creatinine. Data comprise nine urine sets each being composed of eight urine samples: one sample was collected before (“pre”) and seven samples after (“0.5 h post” to “24 h post”) ramson ingestion.
We additionally observed some variance in the metabolite excretion patterns for the same individuals when test persons provided urine sets at different days. In Figure 6, time-resolved metabolite profiles of three urine sets, (U I, U IV, and U VIII) provided by the same test person, are exemplarily displayed. The temporal excretion pattern between the testing days was comparable; in each case the highest metabolites concentrations were observed between 1 and 2 h for each of the investigated metabolites. However, the amounts of excreted metabolites differed between the testing days. In U I the maximal concentration of AMSO and AMSO2 was about 100 μg/mmol creatinine (106.9 and 97.7 μg/mmol creatinine, respectively). In U IV the highest amount of these metabolites was about 50 μg/mmol creatinine (56.2 and 48.3 μg/mmol creatinine) and in U VIII a maximum concentration of 76.6 μg/mmol creatinine AMSO and 69.1 μg/mmol creatinine AMSO2 was detected. Likewise, the maximum concentration of AMS differed between the urine sets, albeit to a lesser extent: AMS concentrations of 0.7, 0.4 and 0.6 μg/mmol creatinine were observed in U I, U IV, and U VIII, respectively.
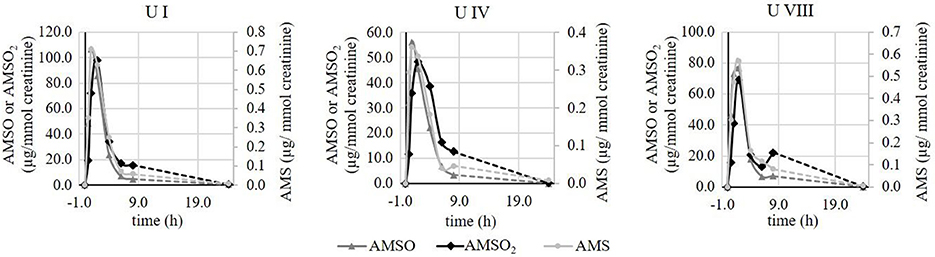
Figure 6. Time-resolved metabolite profiles of ramson-derived metabolites (AMS, AMSO, and AMSO2) of three urine sets (U I, U IV, and U VIII) provided by the same test person at different days. Time 0 h corresponds to the time of ramson consumption. • AMS, ▴ AMSO, ♦ AMSO2. Broken line: time interval, when no sample was collected.
To exclude that these differences were due to different ramson samples and correspondingly natural differences in the respective plant material, additional experiments were conducted testing a total of four subjects on the same day. Panelists were asked to consume the same aliquots of the same ramson sample. The time-resolved metabolite profiles of the respective test persons obtained during this testing day were recorded and data are shown in Figure 7.
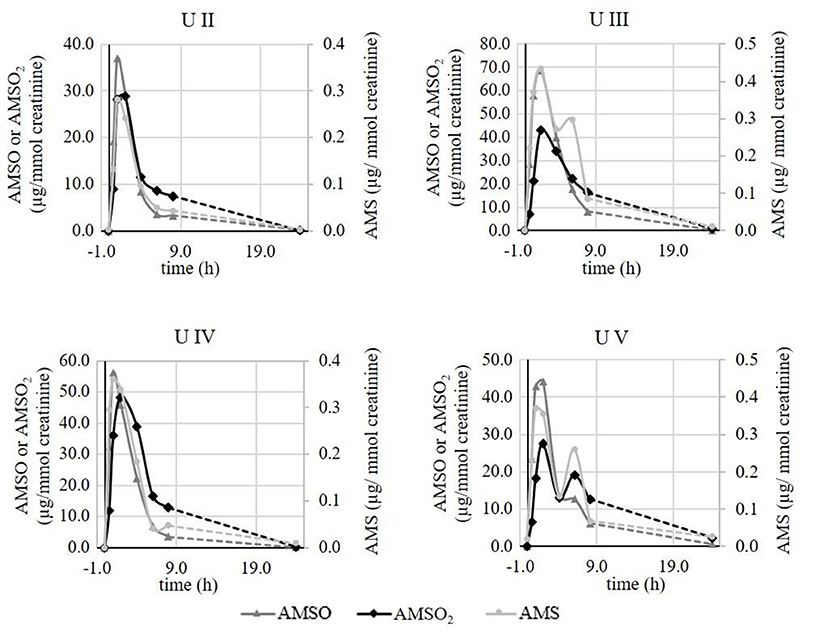
Figure 7. Time-resolved metabolite profiles of ramson-derived metabolites (AMS, AMSO, and AMSO2) of four urine sets (U II, U III, U IV, U V). All four test persons consumed ramson aliquots from the same ramson sample. Time 0 h corresponds to the time of ramson consumption. • AMS, ▴ AMSO, ♦ AMSO2. Broken line: time interval, when no sample was collected.
The analyses revealed that there were still differences in the respective metabolite profiles, although all four test persons consumed 10 g aliquots from the same ramson sample. All profiles revealed a metabolite increase within 2 h after ramson consumption. However, in case of test person V a second increase was observed after about 6 h for all three ramson-derived metabolites. Likewise, a second increase was monitored in urine set U III for AMS. In all other cases we observed only one maximum. Furthermore, there were differences in the metabolite concentrations excreted after ramson consumption comparable to those that were observed when subjects were tested at different days. While the maximum AMS concentration showed only minor differences, with values between 0.3 and 0.4 μg/mmol creatinine, AMSO maxima varied between 36.9 and 68.8 μg/mmol creatinine and in case of AMSO2 between 27.5 and 48.3 μg/mmol creatinine. These observations are limited to four test persons. It provides a first insight into the metabolism and excretion processes of ramson-derived metabolites. However, the differences between test persons might be more or less pronounced if a larger test group is tested.
Discussion
Aroma profile analyses, conducted via orthonasal evaluation, revealed a change in the human milk odor after consumption of about 10 g of ramson which was most pronounced about 2 to 5 h after ramson consumption. As confirmed by our expert sensory panel this change comprised a garlic-/cabbage-like odor which was only perceived in milk samples collected after ramson consumption. Such change was already described in milk samples that were obtained after garlic consumption (Scheffler et al., 2016b). However, whereas a clear aroma change was already observed after consumption of 3 g of raw garlic, in case of ramson about 10 g had to be consumed. Hence, garlic seems to have a higher impact on the human body odor compared to ramson. This is in line with the general observation that garlic consumption influences the body odor, e.g., breath, to a higher extent than ramson consumption (Borrelli et al., 2007). However, it still needs to be kept in mind, that all observed sensory attributes, including the attribute garlic-/cabbage-like, were rated in an orthonasal evaluation as being just detectable (intensity 1 or lower). Nevertheless, this does not rule out that the effect might have been more pronounced during tasting, i.e., retronasal evaluation. However, due to work safety consideration this type of evaluation was not carried out by the expert panel.
In relation to ramson consumption three metabolites were identified in human urine and milk, namely AMS, AMSO, and AMSO2. Of these, AMS had a garlic-/cabbage-like odor whereas the other two compounds were odorless. We found that AMS is related to the aroma change in the milk samples after ramson consumption.
Generally, the transfer of food components originating from the maternal diet into breast milk are not yet fully understood but it has been postulated that compounds that are relevant to the specific flavors of foods and beverages may affect the sensory quality of breast milk, as has been reported in the case of carrots or alcohol (Mennella and Beauchamp, 1991, 1999). On the other hand, studies on the potential influence of herbal tea or encapsulated fish oil products contradict these findings, as they ruled out any sensory or chemical changes in human milk composition (Sandgruber et al., 2011; Denzer et al., 2015). Here, we report a condiment that has the potential of affecting the milk composition of nursing mothers. In most cases, irrespective of the consumed amount of ramson and the investigated excretion product, AMS, AMSO, and AMSO2, were only detected in samples after ramson consumption, demonstrating that they clearly derived from ramson consumption. In previous studies we were able to identify the same metabolites in urine and milk samples obtained after garlic consumption (Scheffler et al., 2016a,b). It can be assumed that AMS, AMSO, and AMSO2 are not ramson-specific metabolites but may be rather linked to several sulfur constituents-bearing foods. On the other hand, further sulfury and/or odorous compounds of ramson such as 2-vinyl-4H-1,3-dithiin, 3-vinyl-4H-1,2-dithiin, AMDS, APDS, APS, DADS, DAS, DATS, DMDS, DMS, DPDS, DPTS, MPDS, and MPTS were proven to be absent at levels as low as 1–10 μg/mL, both in samples collected before as well as after ramson consumption. This leads to the conclusion that ramson constituents are heavily metabolized and eliminated via other physiological routes.
The highest observed AMSO concentration in human milk ranged between 38 and 90 μg/mL human milk; the highest observed AMSO2 concentration ranged between 53 and 99 μg/mL human milk. Similar concentrations of the respective metabolites were observed in human milk after garlic consumption. However, the amount of consumed ramson was more than three times as high as the consumed garlic amount (10 vs. 3 g) (Scheffler et al., 2018). The same applies when ramson- and garlic-derived metabolites in urine are compared. These differences are likely related to the different compositions of garlic and ramson. Both plants contain high amounts of S-alk(en)ylcysteine sulfoxides. The quantitatively dominating S-alk(en)ylcystein sulfoxide in garlic is alliin [S-allylcysteine sulfoxide, 481–1,140 mg/100 g fresh weight (fw)] followed by methiin (S-allylcysteine sulfoxide 50–126 mg/100 g fw) (Kubec et al., 1999) whereas in ramson it is methiin (60 mg/100 g fw) followed by alliin (40 mg/100 g fw) (Kubec et al., 2000). Accordingly, garlic and ramson comprise the same sulfurous compounds that are odorless themselves, but are precursors for odor-active compounds. These precursors are present at different ratios and concentrations, with garlic containing about ten times the quantity of S-alk(en)ylcysteine sulfoxides compared to ramson. Upon rapture various aroma active compounds are formed. The degradation process of the S-alk(en)ylcysteine sulfoxides is exemplarily shown for alliin in Figure 8. Upon ingestion a mixture of sulfur-containing compounds is taken in. To the best of our knowledge, there have been no studies regarding the metabolism of ramson in the human body. However, consumption of ramson and garlic leads to excretion of the same volatile compounds, both via urine and milk. It is conceivable that the metabolism of ramson is related to the metabolism of garlic and follows the same routes.
As discussed in our previous publication the sulfurous compounds containing a dithioallyl-group, e.g. DADS, are transformed to allyl mercaptan which is subsequently methylated to AMS (Germain et al., 2002; Lawson and Wang, 2005). The formation of AMS after garlic consumption was previously confirmed by our group (Scheffler et al., 2016a,b). Now, we can also confirm the presence of AMS in human milk and urine after ramson consumption. Likewise, we demonstrated that AMSO and AMSO2 are metabolites of garlic as well as of ramson constituents. Importantly, the pronounced differences in the amounts of S-alk(en)yl sulfoxides in the garlic and ramson plant were reflected in the detected quantities of AMS, AMSO, and AMSO2. Moreover, we observed differences between test persons that consumed aliquots of the same ramson sample. Accordingly, these inter-individual differences are most likely related to the individual metabolic conversion steps and respective enzyme activities due to genetic polymorphisms of enzymes, as has already been reported for drugs (Evans and Relling, 1999). Furthermore, the physiological status of each individual such as body mass index may influence the metabolism of ramson components (Bachour et al., 2012). In case of the milk samples, lactation period might be another influencing factor since the milk composition changes continuously throughout lactation and is adapted to the age of the infant.
Conclusion
The impact of ramson consumption on human milk and urine composition was investigated. Samples were collected after ramson consumption and were investigated in comparison to control samples obtained prior to ramson consumption by means of GC-MS/O and GC-GC-MS/O. The analyses revealed three ramson-derived metabolites, namely AMS, AMSO, and AMSO2. Furthermore, the excretion profiles of these metabolites in human milk and urine were investigated. Therefore samples were collected over a time interval of about 24 h in case of urine samples and up to 9 h in case of milk samples. In the milk sets we observed the maximum concentrations of the metabolites within 3 h after ramson consumption. Likewise, the highest AMS and AMSO concentrations in urine sets were observed within 2 h after ramson consumption. The maximum concentration of AMSO2 was reached about 2 to 4 h after ramson ingestion. Overall, the present study revealed that ramson constituents are excreted correspondingly to garlic constituents albeit at lower concentration levels, correspondingly to lower content of the respective precursor substances in ramson.
Data Availability Statement
All datasets generated for this study are included in the manuscript and the supplementary files.
Author Contributions
LS, CS, and AB conceived and designed the experiments. LS performed the experiments. TA contributed with analyses of ramson samples. LS analyzed the data. AB contributed reagents materials analysis tools. LS and CS conceived the publication that was approved by AB. All authors have read and approved the final manuscript.
Funding
The authors gratefully acknowledge funding from the German Academic Scholarship Foundation (Studienstiftung des Deutschen Volkes) for Laura Scheffler, and funding of instrumentation used in this study granted by the German research foundation (DFG) in the frame of grant INST 90/979-1 FUGG.
Conflict of Interest Statement
The authors declare that the research was conducted in the absence of any commercial or financial relationships that could be construed as a potential conflict of interest.
Acknowledgments
We are grateful to Yvonne Sauermann for skillfull technical assistance. We thank Prof. Pischetsrieder for providing access to the photometer used within this study. We are also grateful to all volunteers who participated in this study.
Supplementary Material
The Supplementary Material for this article can be found online at: https://www.frontiersin.org/articles/10.3389/fchem.2018.00410/full#supplementary-material
Abbreviations
AMDS, allyl methyl disulfide; AMS, allyl methyl sulfide; AMSO, allyl methyl sulfoxide; AMSO2, allyl methyl sulfone; APDS, allyl propyl disulfide; APS, allyl propyl sulfide; DADS, diallyl disulfide; DAS, diallyl sulfide; DASO, diallyl sulfoxide; DASO2, diallyl sulfone; DATS, diallyl trisulfide; DCM, dichloromethane; DMDS, dimethyl disulfide; DMTS, dimethyl trisulfide; DPDS, dipropyl disulfide; DPTS, dipropyl trisulfide; FID, flame ionization detector; HRGC-GC-MS/O, two-dimensional high-resolution gas chromatography-mass spectrometry/olfactometry; HRGC-MS, high-resolution gas chromatography-mass spectrometry; HRGC-O, high-resolution gas chromatography-olfactometry; MPDS, methyl propyl disulfide; MPTS, methyl propyl trisulfide; RI, (linear) retention index; SAFE, solvent assisted flavor evaporation; SIDA, stable isotope dilution assay; SIM, selected ion monitoring.
References
Abu-Lafi, S., Dembicki, J. W., Goldshlag, P., Hanus, L. O., and Dembitsky, V. M. (2004). The use of the 'Cryogenic' GC/MS and on-column injection for study of organosulfur compounds of the Allium sativum. J. Food Composition Anal. 17, 235–245. doi: 10.1016/j.jfca.2003.09.002
Bachour, P., Yafawi, R., Jaber, F., Choueiri, E., and Abdel-Razzak, Z. (2012). Effects of smoking, mother's age, body mass index, and parity number on lipid, protein, and secretory immunoglobulin A concentrations of human milk. Breastfeed Med. 7, 179–188. doi: 10.1089/bfm.2011.0038
Belitz, H. D., Grosch, W., and Schieberle, P. (2008). Lehrbuch der Lebensmittelchemie. Berlin; Heidelberg: Springer-Verlag.
Benkeblia, N., and Lanzotti, V. (2007). Allium thiosulfinates: chemistry, biological properties and their potential utilization in food preservation. Food 1, 193–201.
Blazewicz-Wozniak, M., and Michowska, A. (2011). The growth, flowering and chemical composition of leaves of three ecotypes of Allium ursinum L. Acta Agrobotanica 64, 171–180. doi: 10.5586/aa.2011.058
Borrelli, F., Capasso, R., and Izzo, A. A. (2007). Garlic (Allium sativum L.): adverse effects and drug interactions in humans. Mol. Nutri. Food Res. 51, 1386–1397. doi: 10.1002/mnfr.200700072
Brady, J. F., Ishizaki, H., Fukuto, J. M., Lin, M. C., Fadel, A., Gapac, J. M., et al. (1991). Inhibition of cytochrome P-450 2E1 by diallyl sulfide and its metabolites. Chem. Res. Toxicol. 4, 642–647. doi: 10.1021/tx00024a008
Buhr, K., Eisgruber, K., Kiefl, J., and Schieberle, P. (2009). “Garlic breath sampling and monitoring by proton transfer reaction-mass spectrometry,” in Proceedings of Fourth PTR-MS Conference (Innsbruck).
Cai, X. J., Block, E., Uden, P. C., Quimby, B. D., and Sullivan, J. J. (1995). Allium chemistry - Identification of natural-abundance organoselenium compounds in human breath after ingestion of garlic using gas-chromatography with atomic-emission detection. J. Agric. Food Chem. 43, 1751–1753. doi: 10.1021/jf00055a001
Calvo-Gómez, O., Morales-Lopez, J., and Lopez, M. G. (2004). Solid-phase microextraction-gas chromatographic-mass spectrometric analysis of garlic oil obtained by hydrodistillation. J. Chromatogr. A 1036, 91–93. doi: 10.1016/j.chroma.2004.02.072
Choudhary, P. R., Jani, R. D., and Sharma, M. S. (2017). Effect of raw crushed garlic (Allium sativum L.) on components of metabolic syndrome. J. Diet. Suppl. 15, 499–506. doi: 10.1080/19390211.2017.1358233
Denzer, M. Y., Kirsch, F., and Buettner, A. (2015). Are odorant constituents of herbal tea transferred into human milk? J. Agric. Food Chem. 63, 104–111. doi: 10.1021/jf504073d
Engel, W., Bahr, W., and Schieberle, P. (1999). Solvent assisted flavour evaporation – a new and versatile technique for the careful and direct isolation of aroma compounds from complex food matrices. Eur. Food Res. Technol. 209, 237–241. doi: 10.1007/s002170050486
Evans, W. E., and Relling, M. V. (1999). Pharmacogenomics: translating functional genomics into rational therapeutics. Science 286, 487–491. doi: 10.1126/science.286.5439.487
Galoburda, R., Bodniece, K., and Talou, T. (2013). Allium sativum flavor compounds as an indicator for garlic identity and quality determination. J. Food Sci. Eng. 3, 226–234. doi: 10.17265/2159-5828/2013.05.002
Germain, E., Auger, J., Ginies, C., Siess, M. H., and Teyssier, C. (2002). In vivo metabolism of diallyl disulphide in the rat: identification of two new metabolites. Xenobiotica 32, 1127–1138. doi: 10.1080/0049825021000017902
Godevac, D., Vujisić, L., Mojović, M., Ignjatović, A., Spasojević, I., and Vajs, V. (2008). Evaluation of antioxidant capacity of Allium ursinum L. Volatile oil and its effect on membrane fluidity. Food Chem. 107, 1692–1700. doi: 10.1016/j.foodchem.2007.10.017
Hansanugrum, A., and Barringer, S. A. (2010). Effect of milk on the deodorization of malodorous breath after garlic ingestion. J. Food Sci. 75, C549–C558. doi: 10.1111/j.1750-3841.2010.01715.x
Humbert, J. A., Hammond, K. B., and Hathaway, W. E. (1970). Trimethylaminuria: the fish-odour syndrome. Lancet 2, 770–771.
Kimbaris, A. C., Siatis, N. G., Daferera, D. J., Tarantilis, P. A., Pappas, C. S., and Polissiou, M. G. (2006). Comparison of distillation and ultrasound-assisted extraction methods for the isolation of sensitive aroma compounds from garlic (Allium sativum). Ultrasonics Sonochem. 13, 54–60. doi: 10.1016/j.ultsonch.2004.12.003
Kubec, R., Svobodová, M., and Velišek, J. (1999). Gas chromatographic determination of S-alk(en)ylcysteine sulfoxides. J. Chromatogr. A 862, 85–94. doi: 10.1016/S0021-9673(99)00902-4
Kubec, R., Svobodova, M., and Velísek, J. (2000). Distribution of S-alk(en)ylcysteine sulfoxides in some Allium species. Identification of a new flavor precursor: S-ethylcysteine sulfoxide (Ethiin). J. Agric. Food Chem. 48, 428–433. doi: 10.1021/jf990938f
Lawson, L. D., and Wang, Z. J. (2005). Allicin and allicin-derived garlic compounds increase breath acetone through allyl methyl sulfide: use in measuring allicin bioavailability. J. Agric. Food Chem. 53, 1974–1983. doi: 10.1021/jf048323s
Lee, S. N., Kim, N. S., and Lee, D. S. (2003). Comparative study of extraction techniques for determination of garlic flavor components by gas chromatography-mass spectrometry. Anal. Bioanal. Chem. 377, 749–756. doi: 10.1007/s00216-003-2163-z
Mazza, G., Ciaravolo, S., Chiricosta, G., and Celli, S. (1992). Volatile flavour components from ripening and mature garlic bulbs. Flavour Fragrance J. 7, 111–116. doi: 10.1002/ffj.2730070303
Mennella, J. A., and Beauchamp, G. K. (1991). The transfer of alcohol to human milk. New Engl. J. Med. 325, 981–985. doi: 10.1056/NEJM199110033251401
Mennella, J. A., and Beauchamp, G. K. (1999). Experience with a flavor in mother's milk modifies the infant's acceptance of flavored cereal. Dev. Psychobiol. 35, 197–203. doi: 10.1002/(SICI)1098-2302(199911)35:3<197::AID-DEV4>3.0.CO;2-J
Mennella, J. A., Jagnow, C. P., and Beauchamp, G. K. (2001). Prenatal and postnatal flavor learning by human infants. Pediatrics 107:e88. doi: 10.1542/peds.107.6.e88
Mondy, N., Naudin, A., Christides, J. P., Mandon, N., and Auger, J. (2001). Comparison of GC-MS and HPLC for the analysis of allium volatiles. Chromatographia 53, S356–S360. doi: 10.1007/BF02490356
Munch, R., and Barringer, S. A. (2014). Deodorization of garlic breath volatiles by food and food components. J. Food Sci. 79, C526–C533. doi: 10.1111/1750-3841.12394
Pelchat, M. L., Bykowski, C., Duke, F. F., and Reed, D. R. (2011). Excretion and perception of a characteristic odor in urine after asparagus ingestion: a psychophysical and genetic study. Chem. Senses 36, 9–17. doi: 10.1093/chemse/bjq081
Radulović, N. S., Miltojević, A. B., Stojković, M. B., and Blagojević, P. D. (2015). New volatile sulfur-containing compounds from wild garlic (Allium ursinum L., Liliaceae). Food Res. Int. 78, 1–10. doi: 10.1016/j.foodres.2015.11.019
Reinhart, K. M., Coleman, C. I., Teevan, C., Vachhani, P., and White, C. M. (2008). Effects of garlic on blood pressure in patients with and without systolic hypertension: a meta-analysis. Ann. Pharmacother. 42, 1766–1771. doi: 10.1345/aph.1L319
Ried, K. (2016). Garlic lowers blood pressure in hypertensive individuals, regulates serum cholesterol, and stimulates immunity: an updated meta-analysis and review. J. Nutri. 146, 389S−396S. doi: 10.3945/jn.114.202192
Rosen, R. T., Hiserodt, R. D., Fukuda, E. K., Ruiz, R. J., Zhou, Z. Y., Lech, J., et al. (2000). The determination of metabolites of garlic preparations in breath and human plasma. Biofactors 13, 241–249. doi: 10.1002/biof.5520130137
Sabha, D., Hiyasat, B., Grötzinger, K., Hennig, L., Schlegel, F., Mohr, F. W., et al. (2012). Allium ursinum L.: bioassay-guided isolation and identification of a galactolipid and a phytosterol exerting antiaggregatory effects. Pharmacology 89, 260–269. doi: 10.1159/000337380
Sandgruber, S., Much, D., Amann-Gassner, U., Hauner, H., and Buettner, A. (2011). Sensory and molecular characterisation of human milk odour profiles after maternal fish oil supplementation during pregnancy and breastfeeding. Food Chem. 128, 485–494. doi: 10.1016/j.foodchem.2011.03.058
Scheffler, L., Sauermann, Y., Heinlein, A., Sharapa, C., and Buettner, A. (2016a). Detection of volatile metabolites derived from garlic (Allium sativum) in human urine. Metabolites 6:E43. doi: 10.3390/metabo6040043
Scheffler, L., Sauermann, Y., Zeh, G., Hauf, K., Heinlein, A., Sharapa, C., et al. (2016b). Detection of volatile metabolites of garlic in human breast milk. Metabolites 6:E18. doi: 10.3390/metabo6020018
Schmitt, B., Schulz, H., Storsberg, J., and Keusgen, M. (2005). Chemical characterization of allium ursinum L. Depending on harvesting time. J. Agric. Food Chem. 53, 7288–7294. doi: 10.1021/jf0504768
Sendl, A., Elbl, G., Steinke, B., Redl, K., Breu, W., and Wagner, H. (1992). Comparative pharmacological investigations of Allium ursinum and Allium sativum. Planta Med. 58, 1–7. doi: 10.1055/s-2006-961378
Sobolewska, D., Podolak, I., and Makowska-Was, J. (2013). Allium ursinum: botanical, phytochemical and pharmacological overview. Phytochem. Rev. 14, 81–97. doi: 10.1007/s11101-013-9334-0
Suarez, F., Springfield, J., Furne, J., and Levitt, M. (1999). Differentiation of mouth versus gut as site of origin of odoriferous breath gases after garlic ingestion. Am. J. Physiol. Gastrointest. Liver Physiol. 276, G425–G430. doi: 10.1152/ajpgi.1999.276.2.G425
Taucher, J., Hansel, A., Jordan, A., and Lindinger, W. (1996). Analysis of compounds in human breath after ingestion of garlic using proton-transfer-reaction mass spectrometry. J. Agric. Food Chem. 44, 3778–3782. doi: 10.1021/jf960640e
Tokarska, B., and Karwowska, K. (1983). The role of sulfur-compounds in evaluation of flavoring value of some plant raw-materials. Nahrung Food 27, 443–447. doi: 10.1002/food.19830270514
Keywords: human milk, human urine, gas chromatography mass-spectrometry/olfactometry, allyl methyl sulfide, allyl methyl sulfoxide, allyl methyl sulfone, stable isotope dilution analysis (SIDA)
Citation: Scheffler L, Sharapa C, Amar T and Buettner A (2018) Identification and Quantification of Volatile Ramson-Derived Metabolites in Humans. Front. Chem. 6:410. doi: 10.3389/fchem.2018.00410
Received: 03 June 2018; Accepted: 20 August 2018;
Published: 11 September 2018.
Edited by:
Mirko Bunzel, Karlsruher Institut für Technologie (KIT), GermanyReviewed by:
Jorry Dharmawan, Singapore Institute of Technology, SingaporeSusana P. Alves, Faculdade de Medicina Veterinária, Universidade de Lisboa, Portugal
Zuobing Xiao, Shanghai Institute of Technology, China
Copyright © 2018 Scheffler, Sharapa, Amar and Buettner. This is an open-access article distributed under the terms of the Creative Commons Attribution License (CC BY). The use, distribution or reproduction in other forums is permitted, provided the original author(s) and the copyright owner(s) are credited and that the original publication in this journal is cited, in accordance with accepted academic practice. No use, distribution or reproduction is permitted which does not comply with these terms.
*Correspondence: Andrea Buettner, YW5kcmVhLmJ1ZXR0bmVyQGZhdS5kZQ==