- 1College of Physics and State Key Laboratory of Bio-Fibers and Eco-Textiles, Qingdao, China
- 2National Engineering Research Center for Intelligent Electrical Vehicle Power System, Power & Energy Storage System Research Center, Qingdao University, Qingdao, China
- 3School of Physics and Technology, University of Jinan, Jinan, China
- 41D Nanomaterials Group, National Institute for Materials Science, Tsukuba, Japan
- 5Key Laboratory of Microelectronic Devices & Integrated Technology, Institute of Microelectronics, Chinese Academy of Sciences, Beijing, China
Porous self-assembled α-Fe2O3 hollow microspheres were fabricated via an ionic liquid-assisted solvothermal reaction and sequential calcinations. The concentration of the ionic liquid (1-butyl-3-methylimidazolium tetrafluoroborate [C4Mim]BF4) was found to play a crucial role in the control of these α-Fe2O3 hollow structures. Trace amounts ionic liquid was used as the soft template to synthesize α-Fe2O3 hollow spheres with a large specific surface (up to 220 m2/g). Based on time-dependent experiments, the proposed formation mechanisms were presented. Under UV light irradiation, the as-synthesized α-Fe2O3 hollow spheres exhibited excellent photocatalysis in Rhodamine B (RhB) photodegradation and the rate constant was 2–3 times higher than α-Fe2O3 particles. The magnetic properties of α-Fe2O3 hollow structures were found to be closely associated with the shape anisotropy.
Introduction
Nanostructured oxides with a variety of useful functionalities are widely applied in photocatalysis, energy storage, etc. (Guo et al., 2017; Li et al., 2017; Zhao et al., 2017, 2018). In particular, hematite (α-Fe2O3), an environmentally-friendly magnetic photocatalyst (Eg = 2.2 eV), has been identified as an important material because of its potential for a wide range of practical applications (Brezesinski et al., 2011; Yang et al., 2014; Zhou et al., 2014; Ma et al., 2018). Recently, self-assembled hematite with highly specific hollow nano/micro-structures and unique properties has emerged as being of great interest to material scientists. To date, various self-assembled hollow α-Fe2O3 nano/micro-structures have been prepared by different synthetic techniques, including the two-step reaction process (Yu J. et al., 2009), hydrothermal (Xu et al., 2012), and solvothermal approaches (Song et al., 2012; Zhu et al., 2012), thermal oxidation at high temperature (Xie et al., 2010), etc. For example, Yu and co-workers successfully fabricated cage-like Fe2O3 hollow spheres and carbonaceous polysaccharide spheres were used as porous crystalline shell templates, followed by calcination at 500°C for 4 h. Song and co-workers proposed a hydrolysis route to synthesis Fe2O3 hierarchical hollow spheres, via a sodium dodecyl benzenesulfonate (SDBS)-assisted hydrolysis process. However, these template methods often suffer from a problem: it is difficult to remove the template and surface-active agent completely. Furthermore, template removal always leads to additional problems in sample morphology. Therefore, it is still necessary to develop a simple route to synthesizing assembled hollow α-Fe2O3 structures.
Ionic liquids (ILs), or “designer liquids,” are known for their superior properties such as recyclability and stable chemical properties. They are used extensively in catalysis, separations, electrochemistry, and especially nanochemistry (Welton, 1999; Wasserscheid and Keim, 2000; Seddon, 2003). The most important advantage of ILs is that they can form extended hydrogen bond systems in the liquid, thereby enabling highly-structured nanostructures (Mele et al., 2003). This special performance can be used as the “entropic driver” for synthesis of self-assembled nanostructures. It has been proved that ILs can be used not only as solvents, but also as templates for preparing nanomaterials with improved properties (Endres et al., 2003; Cooper et al., 2004; Liu et al., 2006). For instance, a variety of nano/micro-structures have been synthesized via ILs, especially assembled hollow structures such as Bi4O5Br2 and BiOBr spheres (Xia et al., 2011a; Mao et al., 2017) and α-Fe2O3 hollow polyhedral (Xu et al., 2013). These hollow structures may also exhibit unique properties for photocatalysis.
In this work, we report a simple and feasible ionic liquid-assisted solvothermal method to synthesize self-assembled α-Fe2O3 hollow microspheres. The ionic liquid [C4Mim]BF4 acts as a soft template can be easily removed by annealing in air. Compared with α-Fe2O3 particles, the as-synthesized α-Fe2O3 hollow spheres exhibit enhanced photocatalytic activity due to their porous self-assembly structure and higher surface area. As far as we known, α-Fe2O3 porous hollow microspheres with high surface area (above 200 m2/g) prepared via this ionic liquid-assisted solvothermal synthesis route is reported for the first time. Additionally, the possible formation mechanisms have also been proved. The as-prepared α-Fe2O3 samples exhibit ferromagnetic properties and can be recycled easily.
Experimental
Materials and Methods
Materials
Ferric chloride (FeCl3·6H2O, 99%) and ethylenediamine (EDA) were purchased from Tianjin Damao Chemical Co. The ionic liquid ([C4mim]BF4) was obtained from Beijing Solarbio Technology Co. Ethylene glycol (EG) was purchased from Tianjin Chemical Reagent Co. and distilled water was used throughout the experiment.
Synthesis
In a typical experimental process, FeCl3·6H2O (0.81 g) was dissolved into 60.0 mL of ethylene glycol (EG). Then, 0, 0.1, 0.2, 0.3, 0.4, and 0.5 mL of [C4Mim]BF4 were added to the above solution. After 2 h, 3 mL of ethylenediamine (EDA) was added into the mixture solution to form a well-distributed emulsion. After 1 h, the emulsion was transferred into a Teflon-sealed autoclave with a capacity of 100 mL, sealed and heated at 200°C for 20 h. After cooling to room temperature, the resultant precipitate was washed with deionized water and ethanol several times until the solution was neutral. After drying in a vacuum oven at 60°C for 6 h, the dried products were calcined in air at 250°C for 6 h. The reaction time was adjusted to study the mechanism.
Materials Characterization
X-ray diffraction (XRD) patterns were obtained with a Rigaku RINT 2500 diffractometer using a Cu-Kα radiation source (λ = 1.5406 Å). The morphology and microstructure of the as-prepared samples were examined with scanning electron microscopy (SEM, JEOL 6500F). TEM and HRTEM images were recorded with a Tecnai accelerating voltage of 120 and 200 kV, respectively. The nitrogen adsorption/desorption isotherms at 77.35 K were collected on an AUTOSORB iQ-MP instrument after heating the samples at 150°C for 2 h. The surface areas were estimated using the Brunauer-Emmett-Teller (BET) method in the relative pressure range of 0.05–0.3 Pore size distributions were analyzed using nitrogen adsorption data in a Barrett-Joyner-Halenda (BJH) model. Magnetic properties of the as-synthesized samples were measured with a physical property measurement system (PPMS-9T, ever cool II, USA).
Photocatalytic Reaction
20.0 mg of α-Fe2O3 photocatalyst catalyst and 50.0 mL of RhB dye aqueous solution (10.0 mg/L) were mixed in a Py”prex reactor and stirred in the dark for 30 min to reach a complete absorption/desorption equilibrium. Afterwards, the suspension was exposed to a 300 W Xenon lamp and stirred simultaneously. Subsequently, 3.0 mL of suspension was centrifuged at 5,000 rpm for 4 min to remove the photocatalyst. Finally, the concentration of RHB was monitored with a TU-1901 UV-vis spectrophotometer by monitoring the absorbance at 553 nm.
Results and Discussion
Structure and Morphology Characterization
The purity and crystallinity of the as-prepared powder samples are measured by XRD (Figure 1). The XRD shows that all diffraction peaks can be indexed in α-Fe2O3 (JCPDS 33–0664) with structural parameters of a = b = 5.038 Å, c = 13.749 Å, α = β = 90°, and γ = 120°. No other peaks are observed, demonstrating that the α-Fe2O3 products have high-purity and a single phase. Therefore, we can infer that anions and cations of the [C4Mim]BF4 are not doped into the α-Fe2O3 lattice. As the [C4Mim]BF4 addition increases, the peak intensities of α-Fe2O3 also gradually increase, indicating that sample (D) has the highest degree of crystallization. This result proves that the addition of ionic liquid can enhance the crystallization of as-synthesized materials. A similar phenomenon is also observed in the previous report (Xu et al., 2013).
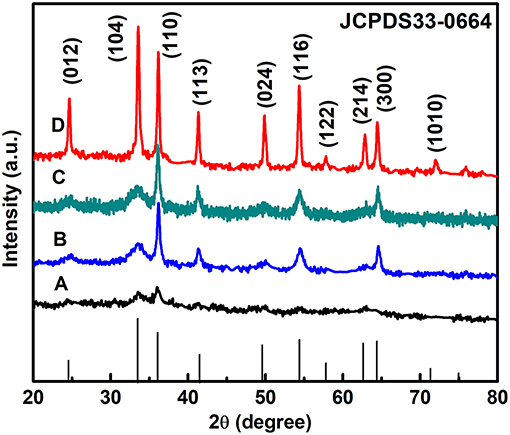
Figure 1. XRD patterns of the α-Fe2O3 with different amounts of [C4Mim]BF4: (A) 0 mL, (B) 0.1 mL, (C) 0.2 mL, and (D) 0.3 mL.
According to the full width at half-maximum (fwhm) of the diffraction peaks, the average crystallite size of the α-Fe2O3 nanoparticles and microspheres ([C4Mim]BF4 = 0.1, 0.2, 0.3 mL) can be estimated from the Scherrer equation to be about 40.2, 84.6, 101.2, and 198.5 nm, respectively.
where Dhkl is the particle size perpendicular to the normal line of (hkl) plane, K is a constant (it is 0.9), βhkl is the full width at half-maximum of the (hkl) diffraction peak, θhkl is the Bragg angle of (hkl) peak, and λ is the wavelength of X-ray.
The morphology and structure of the as-prepared samples are studied by SEM and TEM Figure 2 shows the typical SEM micrographs of the α-Fe2O3 samples prepared with varying [C4Mim]BF4 additions at 200°C for 20 h. In the absence of [C4Mim]BF4 (Figures 2A–C), only α-Fe2O3 pseudo cubic particles are formed. The morphology and size of α-Fe2O3 particles are non-uniform and the alignment is disordered. As the amount of [C4Mim]BF4 increased from 0.1 to 0.3 mL, the as-prepared α-Fe2O3 changed from irregular particles to the uniform microspheres.
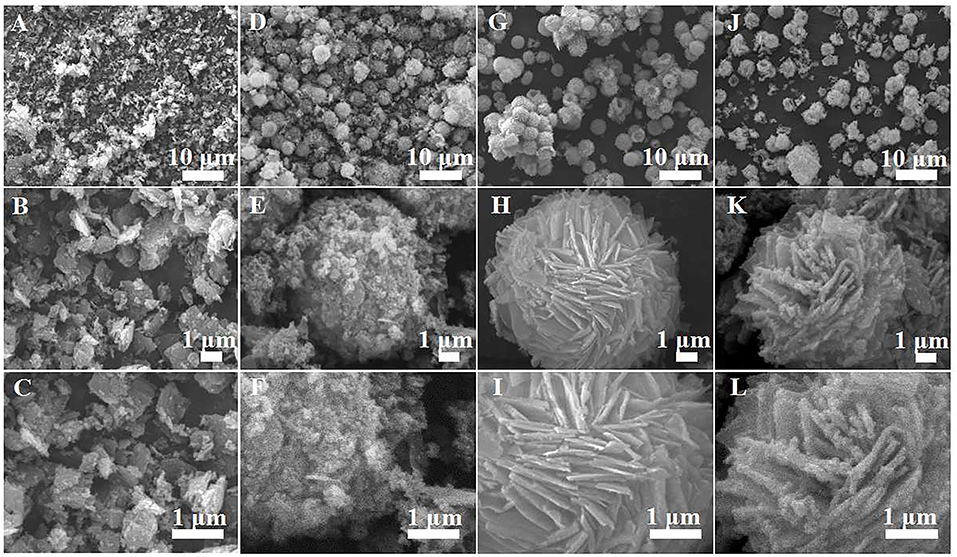
Figure 2. SEM images of α-Fe2O3 samples prepared with varying addition of [C4Mim]BF4 at 200°C for 20 h: (A–C) 0 mL, (D–F) 0.1 mL, (G–I) 0.2 mL, (J–L) 0.3 mL.
Figures 2D–F show the SEM image of [C4Mim]BF4 = 0.1 mL, which is composed of nanoparticles. When the addition of [C4Mim]BF4 is up to 0.2 mL, perfectly spherical α-Fe2O3 is formed. As shown in Figure 2G, some broken α-Fe2O3 microspheres reveal that the as-obtained α-Fe2O3 microspheres are of a hollow structure. Therefore, we can infer that [C4Mim]BF4 is used as a template in the process of α-Fe2O3 synthesis. The three-tiered organization of crystallites for these hollow dandelions is shown in the magnified image (Figure 2H). Numerous nanosheets are present on the surface of the dandelion spheres, with a puffy appearance and the average diameter being about 6.5 μm. From Figure 2I, we can see that individual nanosheets have an average size of about 100 nm, which is in agreement with the result calculated from Scherrer's formula. When the [C4Mim]BF4 amount increases to 0.3 mL (Figures 2J,H,L), the similar nanosheets stack up in three-dimensional ordered microspheres (5.0–7.0 μm) with a larger thickness (about 200 nm). Figure 3A shows the TEM image of an α-Fe2O3 microsphere. However, the structure in [C4Mim]BF4 = 0.3 mL demonstrates no internal void space. A high-resolution TEM (HRTEM) image (Figure 3B) exhibited lattice spacing of 0.252 nm, corresponding to the (110) lattice plane. Additionally, some examples of synthesizing nanostructured α-Fe2O3 using different kinds of ILs are given in Table 1.
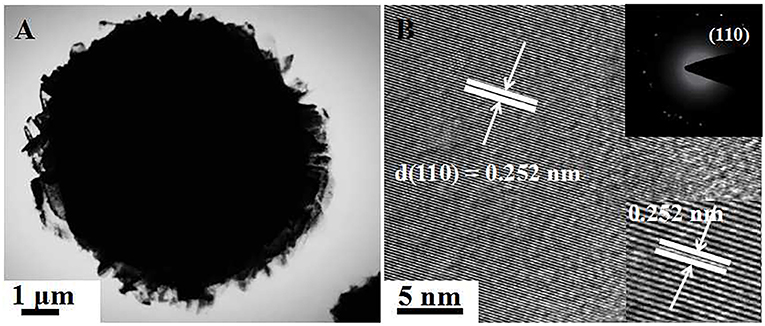
Figure 3. (A) TEM image of single sphere obtained at [C4Mim]BF4 = 0.3 mL, (B) HRTEM image. The inset shows the corresponding SAED pattern (up-right).
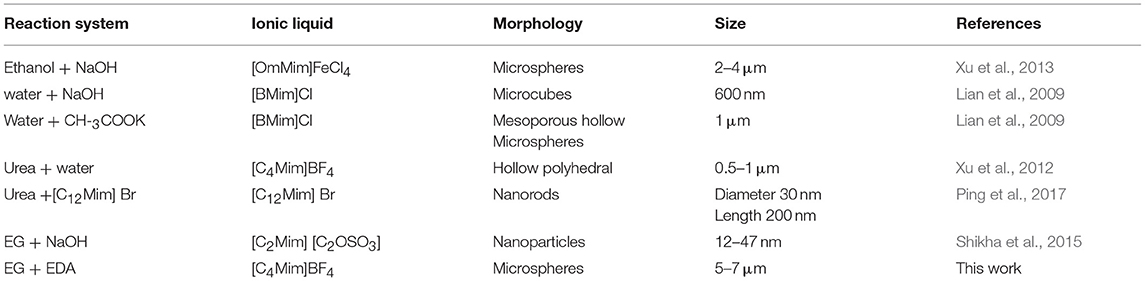
Table 1. Comparison of different α-Fe2O3 samples synthase by this work and others reported in previous references.
Additionally, the self-assembly micron spheres are made up of a bunch of nanosheets, indicating that the spheres are porous in structure. It is striking that when the addition of [C4Mim]BF4 reaches to 0.4 mL, precipitation is very sparse. Until 0.5 mL, no precipitation synthesized after reaction. This phenomenon can be explained as follows: EG and ionic liquids are not mutually soluble, so the two-phase interface is formed. With an increase in the addition of ionic liquids, the disorder of atoms at the two-phase interface increases, resulting in a growing of interfacial energy, which lifts the nucleation resistance. Owing to large resistance, crystal cannot nucleate.
Nitrogen Sorption
Figure 4 shows the nitrogen adsorption-desorption isotherms and pore size distributions of the as-prepared α-Fe2O3. The isotherms of α-Fe2O3 samples shown in Figure 4A are of type IV with a hysteresis loop from 0.5 to 1.0 (P/P0). The BET-specific surface areas of α-Fe2O3 hollow microspheres are calculated to be 183, 208, and 221 m2g−1, all larger than that of α-Fe2O3 synthesized without [C4Mim]BF4 (only 44 m2g−1). Correspondingly, the BJH pore size of the as-synthesized α-Fe2O3 ranges from 5.0 to 10.0 nm (Figure 4B). The smaller pore structures may arise from the crystal growth, and the larger pores ascribe to the stacking of the α-Fe2O3 nano-structures and the hollow structure. Additionally, the peaks of the pore sizes of the α-Fe2O3 move to the right after adding [C4Mim]BF4, showing that average pore size increases. From this result, we can infer that the crystallinity of the as-prepared α-Fe2O3 improved (Zhu et al., 2012), which is in agreement with the XRD analysis. Table 2 summarizes the mean pore size and SBET of the as-obtained α-Fe2O3. It shows that with increasing additions of [C4Mim]BF4, SBET increases dramatically. This result may be attributed to two factors: first, compared with irregular α-Fe2O3 nanoparticles or nanosheets, hollow assembly structures have more opportunities to participate in nitrogen adsorption. Without the [C4Mim]BF4, the specific surface area of α-Fe2O3 is only 44.0 m2g−1 (Xia et al., 2011b). Second, the presence of a large number of mesoporous structures (6–7 nm) leads to a large specific surface area.
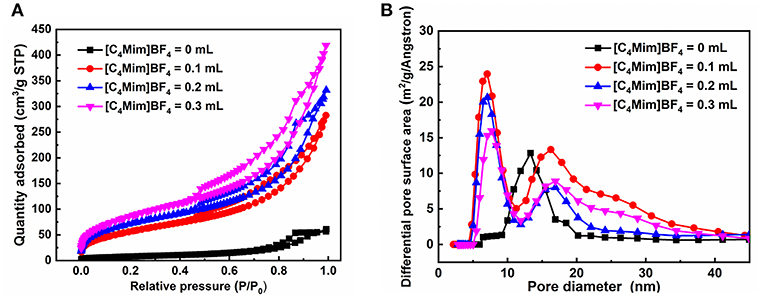
Figure 4. (A) Nitrogen adsorption-desorption isothermal, (B) the pore size distribution curve for the α-Fe2O3.
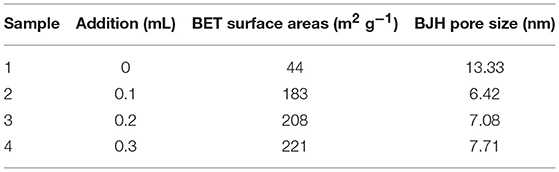
Table 2. Brumaire–Emmett–Teller (BET) surface area and mean pore diameters of the as-prepared samples.
Possible Formation Mechanism of α-Fe2O3 Hollow Spheres
To better understand the formation process of the α-Fe2O3 porous hollow spheres, time-dependent experiments were carried out. As shown in Figures 5A–D, representative SEM images at different time intervals are displayed. First, a hollow core-shell spherical structure was generated at 10 h. The mean diameter of the core is about 2.0 μm, which was further confirmed by the TEM image shown in Figure S1. Second, some microparticles were deposited on its surface at 14 h. Then, as the reaction time reached 16 h, some nanosheets began to cover the surface of the nuclei, indicating that deposition was still in progress. When the duration reached 20 h, a hollow sphere covered with a great number of nanosheets was obtained.
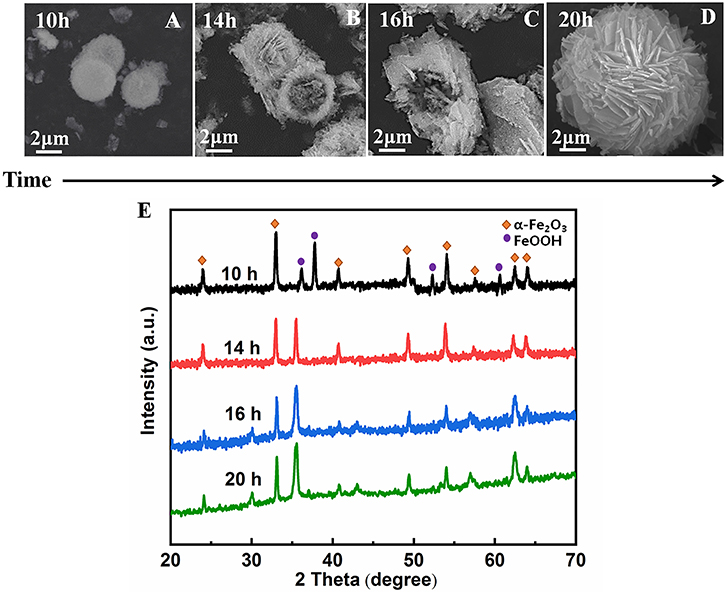
Figure 5. SEM images of α-Fe2O3 hollow microsphere structures synthesized in the presence of ionic liquid [C4Mim]BF4 = 0.2 mL at 200°C. (A)10 h, (B) 14 h, (C) 16 h, (D) 20 h. (E) XRD patterns of the α-Fe2O3 at different time.
Based on the above experimental results, the nucleation and growth mechanism for the microsphere in the two-phase system was proposed, as shown in Figure 6. First, Fe3+ reacted with EDA in EG-[C4Mim]BF4 solution to form a relatively stable complex ion of [Fe (EDA)3]3+ (Zhang et al., 2006). High temperature caused complex ions [Fe (EDA)3]3+ to decompose into FeOOH. Thus, FeOOH was first formed. Then, the FeOOH nanoparticles dissolved and reacted with the obtained Fe2+ in a [C4Mim]BF4 assisted system to form α-Fe2O3 nanoparticles. From Figure 5E, the peaks of FeOOH and α-Fe2O3 can be observed at 10 h. The formed α-Fe2O3 nanoparticles were unstable and had a tendency to form larger congeries, which may have been driven by the minimization of interfacial energy. That is why after 14 h, the sample only showed the crystal image of α-Fe2O3. However, EG solution has greater viscosity and fewer surface hydroxyls than the aqueous solution, resulting in kinetically slower nucleation and aggregation of nanocrystals, which lead to the formation of perfectly oriented assemblies by the adequate rotation to find the low-energy configuration interface (Zhu et al., 2013). A longer reaction time leads to directional alignment to generate spherical nuclei at 10 h. During the subsequent process, nanoparticles gradually assemble into nanosheets and stack on the surface of the template. The reason for the formation of nanosheets is that small particles gradually adhere to large particles to form thin sheets, which is commonly referred as Ostwald ripening. Finally, a specified morphology forms. Such a process is also observed in several other reports (Lou et al., 2006; Zhu et al., 2008; Yu X. et al., 2009). In this study, the adjacent primary ferric alkoxide nanocrystals have high activity due to their high surface energy. They further grow into nanosheets by directional aggregation, which greatly reduces the interface energy of small primary nanocrystals. Then, by directional attachment and self-assembly, the nanosheets gradually evolve into 3D flower-like superstructures. At the same time, it is combined with the mature process of Ostwald to form a favorable hollow structure.
In this formation process, the reaction time is one of the most important controlling factors. Apart from the reaction time, EDA is also a critical factor. EDA is used as a precipitant during this process. When a part of an EG molecule loses protons and coordinates with FeCl3 to form ferric alkoxide, H+ is produced in the reaction of EG with metal chloride. If H+ cannot be removed, the accumulation of H+ will inhibit the formation of further iron oxides. In this paper, EDA takes the lead to react with Fe3+ to form a stable complex and inhibit the formation of H+ in the reaction system.
Enhancement of Photocatalytic Activity
The photocatalytic degradation of RhB is monitored by measuring the absorption behavior of the solution at 553 nm. The evolutions of the spectrums are shown in Figure 7A for α-Fe2O3 synthesized without [C4Mim]BF4 and in Figure 7B for that synthesized with 0.3 mL [C4Mim]BF4. When the addition amount reaches 0.3 mL, 90% of RhB is decomposed after 90 min irradiation, while it is no more than 50% for the sample synthesized without [C4Mim]BF4. Thus, the use of [C4Mim]BF4 in the synthesis process effectively improves the photocatalytic performance. To clarify the effect of [C4mim]BF4, we figure out the rate constants of the degradation processes for the α-Fe2O3 synthesized with various concentrations of [C4Mim]BF4. To evaluate the reactivity, the apparent reaction rate constant (k) is calculated. Figure 7C shows ln (C/C0)—t plots for the α-Fe2O3 samples synthesized with different [C4Mim]BF4 amounts as well as the blank test. The rate constant k is 0.00756, 0.00927, 0.0183, 0.0212 min−1 for [C4Mim]BF4 = 0.0, 0.1, 0.2, and 0.3 mL, respectively, shown in Figure 7D. Thus, the photocatalytic performance is found to improve with increasing [C4Mim]BF4 addition amounts. As shown in Figure 7E, we carried out a coarse comparison of degradation efficiencies between this study and other studies based on the photodegradation of RhB by α-Fe2O3.
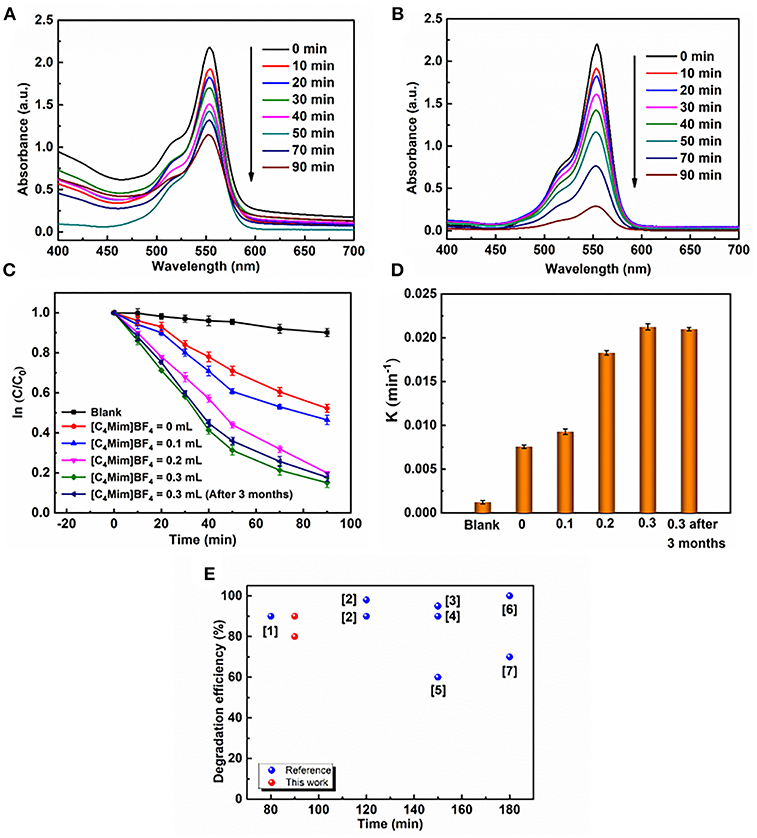
Figure 7. UV–vis absorption spectra during photocatalytic reaction of RhB over α-Fe2O3 with different [C4Mim]BF4 additions at different time intervals: (A) 0 mL, (B) 0.3 mL, (C) plots of ln(C/C0) against time t of various samples: without catalyst, the α-Fe2O3 hollow spheres prepared with addition = 0, 0.1, 0.2, and 0.3 mL, respectively. (D) Reaction kinetics of RhB degradation under visible light irradiation by α-Fe2O3. (E) Comparison of degradation efficiencies of different α-Fe2O3 samples during the RhB photodegradation driven by visible light. Red balls refer to the α-Fe2O3 samples prepared in this work and blue balls refer to the α-Fe2O3 samples reported in previous references. ([1] Xu et al., 2012; [2] (Pawar and Choi, 2015); [3] (Cai et al., 2014); [4] (Xu et al., 2012); [5] (Liang et al., 2014); [6] (Zhou et al., 2011); [7] (Wu et al., 2013)).
In addition to the high catalytic efficiency, the catalyst possessed robust stability. In our case, α-Fe2O3 samples with the amount of 0.3 mL [C4Mim]BF4 also have stable photoactivity. After 3 months, the degradation rate of RHB has little change in k value (Figure 7D). An SEM image of the sample after placement for 3 months is shown in Figure S2. It shows that the morphology and structure of the as-synthesized α-Fe2O3 have few changes, which is very important for maintaining the photocatalytic activity of samples.
The photocatalytic activities of the α-Fe2O3 are closely related to its energy band. Figure 8A shows UV-vis diffuse reflectance spectra of α-Fe2O3 with additions of [C4Mim]BF4 = 0 and 0.3 mL in the wavelengths of 200–800 nm. Although the α-Fe2O3 particles and spheres show similar optical properties, the microsphere with a [C4Mim]BF4 addition of 0.3 mL shows stronger absorption, from 600 to 800 nm, resulting in a larger surface area that can absorb more light (Wang et al., 2011). Therefore, the as-prepared α-Fe2O3 microspheres exhibit better photocatalytic performance. Figure 8B is the plot of (αhv)2 vs. the energy of the absorbed light for α-Fe2O3. From Figure 8B, the band gap is determined to be 2.05 eV for the α-Fe2O3, indicating that the oxygen vacancies do not change the band gaps of α-Fe2O3 microstructures significantly.
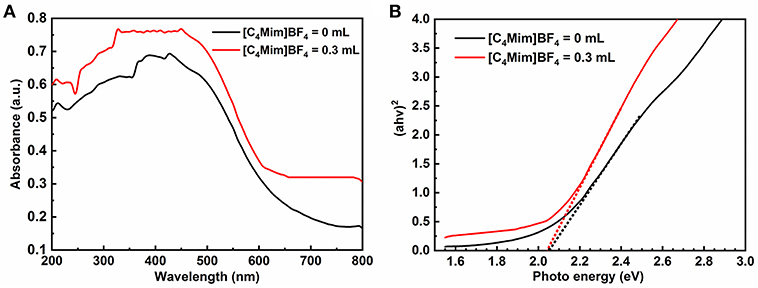
Figure 8. (A) UV-vis diffuse reflectance spectra and (B) plots of (αhv)2 vs. photon energy hv of the samples with addition = 0, 0.3 mL.
Furthermore, in the process of photocatalytic reaction, ·OH is considered to be another main reaction species leading to the oxidative decomposition of organic pollutants. However, it was revealed that ·OH could not be generated during irradiation for Fe2O3 (Xiang et al., 2011). Because the photo-generated holes and electrons on Fe2O3 could not react with OH−/H2O and O2 to form ·OH and O2−, respectively, no ·OH can be generated in Fe2O3 (Xiang et al., 2011). This indicates that reactive species other than ·OH are present. However, several research results suggest that photo-generated holes and electrons can directly give rise to photocatalytic oxidation. As shown in this study, the photocatalytic performances of these α-Fe2O3 samples strongly depend on the [C4Mim]BF4 concentrations in the synthesis process. Therefore, it is likely that the high photocatalytic activity results from the novel structure, large surface area and strong absorption of visible light. Generally, large specific surface area provides more unsaturated coordination, which helps to improve the efficiency of electron hole separation (Tang et al., 2004). Furthermore, the increase of unsaturated coordination sites may improve the surface electron transfer rate. The increase of the surface electron transfer rate leads to the reduction of the probability of recombination and, therefore, the photo-generated charge carriers could more easily transfer to the surface to degrade the adsorbed RhB.
Magnetic Properties
It is well-known that α-Fe2O3 exhibits ferromagnetism (Sun et al., 2010). Figure 9 and Figure S3 shows the room temperature magnetic hysteresis loops and the magnetic field sweeping from −10.0 to 10.0 k Oe. Table 3 collects the values of remnant magnetization (Mr) and coercivity (Hc) of the as-synthesized α-Fe2O3 samples. It can be determined from the shape of the hysteresis loop that the synthesized α-Fe2O3 sample shows ferromagnetism. Additionally, the hysteresis loop did not reach saturation up to the maximum applied magnetic field, because of the presence of large-shape anisotropy (Bharathi et al., 2010). It is also shown in Table 3 that the residual magnetization and coercivity of assembled microspheres of α-Fe2O3 are large than α-Fe2O3 nanoparticles. It is widely understood that the morphology and structure of the as-synthesized samples will greatly affect the magnetization of ferromagnetic materials (Sorescu et al., 1999). Therefore, the assembly of the nano-sized and oriented particles and sheets into non-random structure results in the change of the single domain to the multidomain, leading to higher remnant magnetization and coercivity (Park et al., 2000). In summary, the difference can be attributed to the high crystallization, single domain size, surface (Tong et al., 2015), structure, and shape.
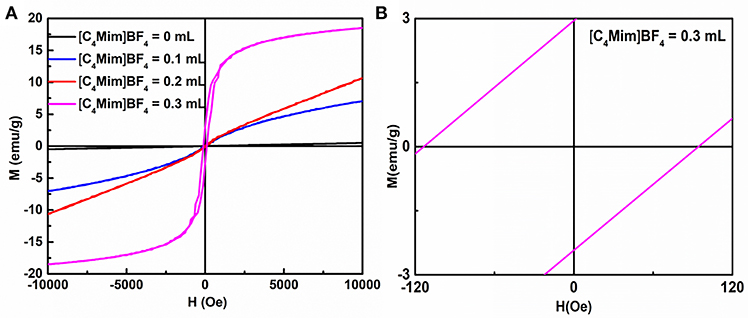
Figure 9. (A) Magnetization loops for α-Fe2O3 particles synthesized with different additions of [C4Mim]BF4. (B) A magnified view of curve.
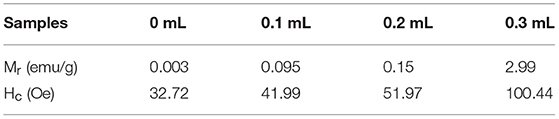
Table 3. The values of remnant magnetization (Mr) and coercivity (Hc) of the as-synthesized samples.
Conclusion
In summary, porous self-assembled α-Fe2O3 hollow microspheres were successfully prepared via a facile ionic liquid assistant synthesis approach. A nucleation–aggregation evacuation mechanism was the main formation of the porous hollow structures. EDA and [C4Mim]BF4 play significant roles on the formation of porous α-Fe2O3 hollow spheres. The increase in [C4Mim]BF4 promotes photocatalytic activities and α-Fe2O3 microspheres show higher photocatalytic activities. We believe that high specific surface area and porous hollow structure play an important role in improving the photocatalytic performance of as-prepared α-Fe2O3. Additionally, when the addition amount reaches 0.3 mL, the synthesized samples show ferromagnetism and can be easily recycled. The α-Fe2O3 hollow porous spheres prepared by our method have high photocatalytic activity, ideal ferromagnetic properties, and high specific surface area. They are expected to exhibit use as applications in sensors, catalysis, separation technology, environmental engineering, controlled drug delivery, and more.
Data Availability
All datasets generated for this study are included in the manuscript and/or the Supplementary Files.
Author Contributions
HY and YulZ partly designed the experiments and wrote the manuscript. QH, JZ, YuaZ, XX, and YL assisted in the analysis and interpretation of the data. JT and FW proposed the project and revised the manuscript.
Conflict of Interest Statement
The authors declare that the research was conducted in the absence of any commercial or financial relationships that could be construed as a potential conflict of interest.
Acknowledgments
The authors acknowledge financial support from the Taishan Outstanding Overseas Scholar Program of Shandong Province, China. The work was financially supported by the Natural Science Foundation of Shandong Province, China (Grant number: ZR2018JL021 and ZR2014EMQ011), the National Natural Science Foundation of China (Grant number: 51402160 and 51673103) and the Qingdao Postdoctoral Application Research Project. The work was also supported by the Opening Project of Key Laboratory of Microelectronic Devices & Integrated Technology, Institute of Microelectronics, Chinese Academy of Sciences, and the National Demonstration Center for Experimental Applied Physics Education (Qingdao University).
Supplementary Material
The Supplementary Material for this article can be found online at: https://www.frontiersin.org/articles/10.3389/fchem.2019.00058/full#supplementary-material
References
Bharathi, S., Nataraj, D., Seetha, M., Mangalaraj, D., Ponpandian, N., Masuda, Y., et al. (2010). Controlled growth of single-crystalline, nanostructured dendrites and snowflakes of α-Fe2O3: influence of the surfactant on the morphology and investigation of morphology dependent magnetic properties. CrystEngComm 12, 373–382. doi: 10.1039/B910550F
Brezesinski, K., Haetge, J., Wang, J., Mascotto, S., Reitz, C., Rein, A., et al. (2011). Ordered mesoporous α-Fe2O3 (hematite) thin-film electrodes for application in high rate rechargeable lithium batteries. Small 7, 407–414. doi: 10.1002/smll.201001333
Cai, J., Chen, S., Ji, M., Hu, J., Ma, Y., and Qi, L. (2014). Organic additive-free synthesis of mesocrystalline hematite nanoplates via two-dimensional oriented attachment. CrystEngComm 16, 1553–1559. doi: 10.1039/C3CE41716F
Cooper, E. R., Andrews, C. D., Wheatley, P. S., Webb, P. B., Wormald, P., and Morris, R. E. (2004). Ionic liquids and eutectic mixtures as solvent and template in synthesis of zeolite analogues. Nature 430:1012. doi: 10.1038/nature02860
Endres, F., Bukowski, M., Hempelmann, R., and Natter, H. (2003). Electrodeposition of nanocrystalline metals and alloys from ionic liquids. Angew. Chem. Int. Ed. 42, 3428–3430. doi: 10.1002/anie.200350912
Guo, X., Zheng, S., Zhang, G., Xiao, X., Li, X., Xu, Y., et al. (2017). Nanostructured graphene-based materials for flexible energy storage. Energy Storage Mater. 9, 150–169. doi: 10.1016/j.ensm.2017.07.006
Li, X., Xue, H., and Pang, H. (2017). Facile synthesis and shape evolution of well-defined phosphotungstic acid potassium nanocrystals as a highly efficient visible-light-driven photocatalyst. Nanoscale 9, 216–222. doi: 10.1039/C6NR07680G
Lian, J., Duan, X., Ma, J., Peng, P., Kim, T., and Zheng, W. (2009). Hematite (α-Fe2O3) with various morphologies: ionic liquid-assisted synthesis, formation mechanism, and properties. ACS Nano 3, 3749–3761. doi: 10.1021/nn900941e
Liang, H., Chen, W., Jiang, X., Xu, X., Xu, B., and Wang, Z. (2014). Synthesis of 2D hollow hematite microplatelets with tuneable porosity and their comparative photocatalytic activities. J. Mater. Chem. A 2, 4340–4346. doi: 10.1039/c3ta14476c
Liu, D. P., Li, G. D., Su, Y., and Chen, J. S. (2006). Highly luminescent ZnO nanocrystals stabilized by ionic-liquid components. Angew. Chem. 118, 7530–7533. doi: 10.1002/ange.200602429
Lou, X. W., Wang, Y., Yuan, C., Lee, J. Y., and Archer, L. A. (2006). Template-free synthesis of SnO2 hollow nanostructures with high lithium storage capacity. Adv. Mater. 18, 2325–2329. doi: 10.1002/adma.200600733
Ma, J., Guo, X., Yan, Y., Xue, H., and Pang, H. (2018). FeOx -based materials for electrochemical energy storage. Adv. Sci. 5:1700986. doi: 10.1002/advs.201700986
Mao, D., Ding, S., Meng, L., Dai, Y., Sun, C., Yang, S., et al. (2017). One-pot microemulsion-mediated synthesis of Bi-rich Bi4O5Br2 with controllable morphologies and excellent visible-light photocatalytic removal of pollutants. Appl. Catal. B Environ. 207, 153–165. doi: 10.1016/j.apcatb.2017.02.010
Mele, A., Tran, C. D., and De Paoli Lacerda, S. H. (2003). The structure of a room-temperature ionic liquid with and without trace amounts of water: the role of C–H···O and C–H···F interactions in 1-n-Butyl-3-Methylimidazolium tetrafluoroborate. Angew. Chem. 115, 4500–4502. doi: 10.1002/ange.200351783
Park, S. J., Kim, S., Lee, S., Khim, Z. G., Char, K., and Hyeon, T. (2000). Synthesis and magnetic studies of uniform iron nanorods and nanospheres. J. Am. Chem. Soc. 122, 8581–8582. doi: 10.1021/ja001628c
Pawar, P., and Choi, D. (2015). Reduced graphene oxide composites with MWCNTs and single crystalline hematite nanorhombohedra for applications in water purification. Int. J. Hydrogen Energy 40, 767–778. doi: 10.1016/j.ijhydene.2014.08.084
Ping, W., Zheng, Z., Cheng, X., Sui, L., Shan, G., Zhang, X. F., et al. (2017). Ionic liquid-assisted synthesis of α-Fe2O3 mesoporous nanorod arrays and their excellent trimethylamine gas-sensing properties for monitoring fish freshness. J. Mater. Chem. A 5, 19846–19856. doi: 10.1039/c7ta06392j
Shikha, P., Randhawa, B., and Kang, T. (2015). Greener synthetic route for superparamagnetic and Luminescent α-Fe2O3 nanoparticles in binary mixtures of ionic liquid and ethylene glycol. Rsc Adv. 5, 51158–51168. doi: 10.1039/C5RA07218B
Song, H. J., Jia, X. H., Qi, H., Yang, X. F., Tang, H., and Min, C. Y. (2012). Flexible morphology-controlled synthesis of monodisperse α-Fe2O3 hierarchical hollow microspheres and their gas-sensing properties. J. Mater. Chem. 22, 3508–3516. doi: 10.1039/c2jm13574d
Sorescu, M., Brand, R. A., Mihaila-Tarabasanu, D., and Diamandescu, L. (1999). The crucial role of particle morphology in the magnetic properties of haematite. J. Appl. Phys. 85, 5546–5548. doi: 10.1063/1.369890
Sun, Z., Yuan, H., Liu, Z., Han, B., and Zhang, X. (2010). A highly efficient chemical sensor material for H2S: α-Fe2O3 nanotubes fabricated using carbon nanotube templates. Adv. Mater. 17, 2993–2997. doi: 10.1002/adma.200501562
Tang, J., Zou, Z., and Ye, J. (2004). Effects of substituting Sr2+ and Ba2+ for Ca2+ on the structural properties and photocatalytic behaviors of CaIn2O4. Chem. Mater. 16, 1644–1649. doi: 10.1021/cm0353815
Tong, G., Liu, Y., Wu, T., Ye, Y., and Tong, C. (2015). High-quality elliptical iron glycolate nanosheets: selective synthesis and chemical conversion into FexOy nanorings, porous nanosheets, and nanochains with enhanced visible-light photocatalytic activity. Nanoscale 7, 16493–16503. doi: 10.1039/C5NR03689E
Wang, F. Y., Yang, Q. D., Xu, G., Lei, N. Y., Tsang, Y. K., Wong, N. B., et al. (2011). Highly active and enhanced photocatalytic silicon nanowire arrays. Nanoscale 3, 3269–3276. doi: 10.1039/c1nr10266d
Wasserscheid, P., and Keim, W. (2000). Ionic liquids—new “solutions” for transition metal catalysis. Cheminform 39, 3772–3789. doi: 10.1002/1521-3773
Welton, T. (1999). Room-temperature ionic liquids: solvents for synthesis and catalysis. Chem. Rev. 99, 2071–2084. doi: 10.1021/cr980032t
Wu, W., Hao, R., Liu, F., Su, X., and Hou, Y. (2013). Single-crystalline α-Fe2O3 nanostructures: controlled synthesis and high-index plane-enhanced photodegradation by visible light. J. Mater. Chem A 1, 6888–6894. doi: 10.1039/c3ta10886d
Xia, J., Yin, S., Li, H., Xu, H., Xu, L., and Xu, Y. (2011a). Improved visible light photocatalytic activity of sphere-like BiOBr hollow and porous structures synthesized via a reactable ionic liquid. Dalton Trans. 40, 5249–5258. doi: 10.1039/c0dt01511c
Xia, J., Yin, S., Li, H., Xu, H., Yan, Y., and Zhang, Q. (2011b). Self-assembly and enhanced photocatalytic properties of BiOI hollow microspheres via a reactable ionic liquid. Langmuir 27, 1200–1206. doi: 10.1021/la104054r
Xiang, Q., Yu, J., and Wong, P. K. (2011). Quantitative characterization of hydroxyl radicals produced by various photocatalysts. J. Colloid Inter. Sci. 357, 163–167. doi: 10.1016/j.jcis.2011.01.093
Xie, H., Li, Y., Jin, S., Han, J., and Zhao, X. (2010). Facile Fabrication of 3D-ordered macroporous nanocrystalline iron oxide films with highly efficient visible light induced photocatalytic Activity. J. Phys. Chem. C 114, 9706–9712. doi: 10.1021/jp102525y
Xu, B., Huang, B., Cheng, H., Wang, Z., Qin, X., Zhang, X., et al. (2012). α-Fe2O3 hollow structures: formation of single crystalline thin shells. Chem. Commun. 48, 6529–6531. doi: 10.1039/c2cc33032f
Xu, L., Xia, J., Wang, K., Wang, L., Li, H., Xu, H., et al. (2013). Ionic liquid assisted synthesis and photocatalytic properties of alpha-Fe2O3 hollow microspheres. Dalton Trans. 42, 6468–6477. doi: 10.1039/c3dt50137j
Yang, S., Song, X., Zhang, P., Sun, J., and Gao, L. (2014). Self-assembled α-Fe2O3 mesocrystals/graphene nanohybrid for enhanced electrochemical capacitors. Small 10, 2270–2279. doi: 10.1002/smll.201303922
Yu, J., Yu, X., Huang, B., Zhang, X., and Dai, Y. (2009). Hydrothermal synthesis and visible-light photocatalytic activity of novel cage-like ferric oxide hollow spheres. Crystal Growth Des. 9, 1474–1480. doi: 10.1021/cg800941d
Yu, X., Yu, J., Cheng, B., and Huang, B. (2009). One-pot template-free synthesis of monodisperse Zinc sulfide hollow spheres and their photocatalytic properties. Chem. A Eur. J. 15, 6731–6739. doi: 10.1002/chem.200900204
Zhang, D. E., Zhang, X. J., Ni, X. M., and Zheng, H. G. (2006). Preparation and characterization of α-FeOOH nanorods via an ethylenediamine-assisted route. Mater. Lett. 60, 1915–1917. doi: 10.1016/j.matlet.2005.12.053
Zhao, G., Cheng, Y., Wu, Y., Xu, X., and Hao, X. (2018). New 2D carbon nitride organic materials synthesis with huge-application prospects in CN photocatalyst. Small 14:e1704138. doi: 10.1002/smll.201704138
Zhao, G., Wang, T., Shao, Y., Wu, Y., Huang, B., and Hao, X. (2017). A novel mild phase-transition to prepare black phosphorus nanosheets with excellent energy applications. Small 13:201602243. doi: 10.1002/smll.201602243
Zhou, X., Lan, J., Liu, G., Deng, K., Yang, Y., Nie, G., et al. (2011). Facet-mediated photodegradation of organic dye over Hematite architectures by visible light. Angew. Chem. Int. Ed. 51, 178–182. doi: 10.1002/anie.201105028
Zhou, X., Xu, Q., Lei, W., Zhang, T., Qi, X., Liu, G., et al. (2014). Origin of tunable photocatalytic selectivity of well-defined α-Fe2O3 nanocrystals. Small 10, 674–679. doi: 10.1002/smll.201301870
Zhu, L. P., Bing, N. C., Wang, L. L., Jin, H. Y., Liao, G. H., and Wang, L. J. (2012). Self-assembled 3D porous flowerlike α-Fe2O3 hierarchical nanostructures: synthesis, growth mechanism, and their application in photocatalysis. Dalton Trans. 41, 2959–2965. doi: 10.1039/c2dt11822j
Zhu, L. P., Wang, L. L., Bing, N. C., Huang, C., Wang, L. J., and Liao, G. H. (2013). Porous fluorine-doped γ-Fe2O3 hollow spheres: synthesis, growth mechanism, and their application in photocatalysis. ACS Appl. Mater. Inter. 5, 12478–12487. doi: 10.1021/am403720r
Keywords: α-Fe2O3, assembly, porous materials, ionic liquid, magnetic properties, visible light, photocatalytic activity
Citation: Yin H, Zhao Y, Hua Q, Zhang J, Zhang Y, Xu X, Long Y, Tang J and Wang F (2019) Controlled Synthesis of Hollow α-Fe2O3 Microspheres Assembled With Ionic Liquid for Enhanced Visible-Light Photocatalytic Activity. Front. Chem. 7:58. doi: 10.3389/fchem.2019.00058
Received: 08 November 2018; Accepted: 21 January 2019;
Published: 27 February 2019.
Edited by:
Tianyi Ma, University of Newcastle, AustraliaReviewed by:
Aihua Xu, Wuhan Textile University, ChinaGang Zhao, University of Jinan, China
Huan Pang, Yangzhou University, China
Copyright © 2019 Yin, Zhao, Hua, Zhang, Zhang, Xu, Long, Tang and Wang. This is an open-access article distributed under the terms of the Creative Commons Attribution License (CC BY). The use, distribution or reproduction in other forums is permitted, provided the original author(s) and the copyright owner(s) are credited and that the original publication in this journal is cited, in accordance with accepted academic practice. No use, distribution or reproduction is permitted which does not comply with these terms.
*Correspondence: Jie Tang, dGFuZy5qaWVAbmltcy5nby5qcA==
Fengyun Wang, Znl3YW5nNzc3QDE2My5jb20=
†These authors have contributed equally to this work