Preparation of Renewable Epoxy-Amine Resins With Tunable Thermo-Mechanical Properties, Wettability and Degradation Abilities From Lignocellulose- and Plant Oils-Derived Components
- 1URD ABI, CEBB, AgroParisTech, Pomacle, France
- 2UMR GENIAL, AgroParisTech, INRA, Université Paris-Saclay, Massy, France
- 3Institut de Recherche en Cancérologie de Montpellier, Val d'Aurelle, Montpellier, France
- 4Department of Chemistry and Chemical Biology, Rensselaer Polytechnic Institute, Troy, NY, United States
One-hundred percent renewable triphenol—GTF—(glycerol trihydroferulate) and novel bisphenols—GDFx–(glycerol dihydroferulate) were prepared from lignocellulose-derived ferulic acid and vegetal oil components (fatty acids and glycerol) using highly selective lipase-catalyzed transesterifications. Estrogenic activity tests revealed no endocrine disruption for GDFx bisphenols. Triethyl-benzyl-ammonium chloride (TEBAC) mediated glycidylation of all bis/triphenols, afforded innocuous bio-based epoxy precursors GDFxEPO and GTF-EPO. GDFxEPO were then cured with conventional and renewable diamines, and some of them in presence of GTF-EPO. Thermo-mechanical analyses (TGA, DSC, and DMA) and degradation studies in acidic aqueous solutions of the resulting epoxy-amine resins showed excellent thermal stabilities (Td5% = 282–310°C), glass transition temperatures (Tg) ranging from 3 to 62°C, tunable tan α, and tunable degradability, respectively. It has been shown that the thermo-mechanical properties, wettability, and degradability of these epoxy-amine resins, can be finely tailored by judiciously selecting the diamine nature, the GTF-EPO content, and the fatty acid chain length.
Introduction
Thermoset polymers are widely used in industrial applications thanks to their versatile performance, good durability, and excellent chemical resistance provided by their highly cross-linked structure (Ellis, 1993; Auvergne et al., 2014; Ramon et al., 2018). It is therefore very common to find these polymers in a broad range of applications such as maintenance coating, adhesives for aerospace (Prolongo et al., 2009) and automobile (Holbery and Houston, 2006) industries, or binders in composites (Gojny et al., 2006). Epoxy resins are one of the most important thermoset materials and are usually synthesized by reacting (poly)phenolic compounds with epichlorohydrin under basic conditions (Bruins, 1968).
The thermoset polymers sector, and more generally the polymer industry, has adopted the current trend which consists in developing greener and more sustainable chemicals and technologies. For instance, Solvay or Dow Chemical have already initiated the transition with the commercialization of bio-based epichlorohydrin prepared from the chlorination of bio-based glycerol, a by-product of biodiesel production (Strebelle et al., 2001; Freddy, 2006). Furthermore, a number of renewable resources—including fatty acids (Biermann et al., 2000; Maisonneuve et al., 2013; Laurichesse et al., 2014), lignocellulosic biomass (Isikgor and Becer, 2015), and enzymatic products—have been reported as alternative feedstocks in polymers synthesis.
Bisphenol A (BPA) is commonly used to prepare commercial high-performance epoxy resins (aka DGEBA, DiGlycidylEther of Bisphenol A). Although DGEBA, upon curing with diamines, leads to cross-linked materials with exceptional properties such as strong adhesion, mechanical integrity and chemical resistance, BPA is recognized as an endocrine disruptive chemical and many studies have been conducted to replace it by innocuous bio-based chemicals. Lignans and lignin-derived chemicals that can be obtained through pyrolysis (Celikbag et al., 2017; Barde et al., 2018) or controlled depolymerization (Pandey and Kim, 2011; Shuai et al., 2016) are the most used biomass-derived chemical platforms for the design of bio-based BPA-substitutes, for the preparation of sustainable thermosets with high thermo-mechanical properties. Indeed, the aromatic moieties present in these bio-based chemicals confer rigidity to the resulting polymers (Wang et al., 2017; Feghali et al., 2018). For example, there have been many reports on epoxy resins from eugenol (Wan et al., 2016), vanillin (Fache et al., 2015a,b; Hernandez et al., 2016; Mauck et al., 2017; Nicastro et al., 2018; Savonnet et al., 2018; Zhao et al., 2018), ferulic and sinapic acids (Maiorana et al., 2016; Janvier et al., 2017; Ménard et al., 2017), guaiacols (Maiorana et al., 2015), and creosol (Meylemans et al., 2011) with promising performances capable of competing with current DGEBA-based materials. However, few researchers have considered the potential toxicity of these BPA substitutes, especially endocrine disruption, which remains a crucial health issue for both plastic sector workers and consumers (Jiang et al., 2018). The degradability of the resulting materials and their behavior regarding water are two other aspects that are also under-investigated. In fact, in most of the studies described above, the thermo-mechanical (e.g., Tg) and physico-chemical (e.g., wettability) properties of the materials cannot be finely tuned because of the lack of apolar moieties in the bio-based aromatic-epoxies.
Plant oil-derived fatty acids are highly valuable building blocks and are frequently used as renewable resources for the synthesis of thermoplastics/thermosets (Meier, 2018). Their long aliphatic chain and their double bond(s) allow a wide number of chemical modifications and provide materials with tunable properties, such as glass transition temperature (Tg), chemical resistance, (bio)degradability, and adhesion strength. Combining the rigidity of bio-based aromatic building blocks and the apolar moiety of the alkyl chain of a fatty acid in a single BPA-substitute thus appears as an interesting approach to finely tune the Tg, the wettability and the degradability of the resulting epoxy-amine resins, after curing with diamines.
In this present work, we aim at developing a series of bio-based epoxy resins, from naturally occurring ferulic acid and plant oils-derived components (i.e., glycerol and fatty acids), to access epoxy-amine thermoset materials with tunable thermo-mechanical properties, wettability and chemical degradation ability. Through highly selective chemo-enzymatic pathways, several polyphenolic compounds, named GDFx and GTF, have been prepared with tunable flexibility and degree of functionality (i.e., 2 or 3). The determination of the toxicity of these compounds has been carried out by determining their binding to human hormone receptors (ERα, PXR, and AR) and was benchmarked against that of commercial and controversial BPA. GDFx and GTF were then converted to their corresponding glycidyl ether by TEBAC-mediated glycidylation in presence of epichlorohydrin. Characterization studies were then performed on the corresponding epoxy resins formulations (i.e., molecular weight distribution and EEW). The latter were finally cured with three crosslinkers (i.e., IPDA, DA10, and DIFFA) and the thermo-mechanical properties as well as wettability and chemical degradation abilities of the resulting epoxy-amine resins were investigated.
Experimental
Material
Ferulic acid, lauric acid, palmitic acid, stearic acid, benzyl bromide, N,N-Dimethylpyridin-4-amine (DMAP), N,N-Diisopropylcarbodiimide (DIC), benzyltriethyl ammonium chloride (TEBAC), and palladium supported on carbon, were supplied by Sigma-Aldrich. Glycerol was purchased from Alfa Aesar. Epichlorohydrin was purchased from Acros Organics. Isophorone diamine (IPDA) and Decane diamine (DA10) were purchased from TCI. Candida antarctica Lipase B (CAL-B) immobilized on resin (LC200291, 10,000 propyl laurate units.g−1) was obtained from Novozyme. All reactants were used as received. All solvents were bought either from ThermoFisher Scientific or VWR. Deuterated chloroform (CDCl3) was purchased from Euriso-top.
Methods
Purification
Column chromatographies were carried out with an automated flash chromatography (PuriFlash 4100, Interchim) and pre-packed INTERCHIM PF-30SI-HP (30 μm silica gel) columns using a gradient of cyclohexane and ethyl acetate for the elution.
Characterization
FT-IR analyses were performed on Cary 630 FT-IR with ATR. NMR analyses were recorded on a Bruker Fourier 300. 1H NMR spectra of samples were recorded in CDCl3 at 300 MHz, chemicals shifts were reported in parts per million (CDCl3 residual signal at δ = 7.26 ppm). 13C NMR spectra of samples were recorded at 75 MHz (CDCl3 signal at δ = 77.16 ppm). HRMS were recorded by the PLANET platform at URCA on a Micromass GC-TOF.
Gel Permeation Chromatography (GPC)
Gel permeation chromatography (GPC) was performed at 40°C on an Infinity 1260 system from Agilent Technologies with PL-gel 5 μM MIXED-D column in THF (flow rate 1 mL.min−1) using conventional PS calibration and UV detection at 280 nm.
Thermo-Gravimetric Analyses (TGA)
Thermo-gravimetric analyses (TGA) were recorded on a Q500, from TA. About 10 mg of each sample was heated at 10°C.min−1 from 30 to 500°C under nitrogen or oxygen flow (60 mL.min−1).
Differential Scanning Calorimetry (DSC)
Differential scanning calorimetry (DSC) thermograms were obtained using a DSC TA Q20, under inert atmosphere (N2), with a calibration using indium, n-octadecane and n-octane standards. For each sample, about 10 mg was weighed in a pan which was then sealed and submitted to three heat/cool/heat cycles: heating from 30 to 200°C at 10°C.min−1, cooling from 200°C to −50°C at 20°C.min−1. Glass transition temperatures (Tg) were determined at the inflection value in the heat capacity jump.
Dynamical Mechanical Analyses (DMA)
Dynamical Mechanical Analyses (DMA) were performed on a DMA Q800 from the TA Instrument. The DMA samples had a rectangular geometry (length: 40 mm, width: 8 mm, thickness: 1.5 mm). Uniaxial stretching of samples was performed while heating at a rate of 3°C.min−1 from 30 to 200°C, keeping frequency at 1 Hz. Deformation was kept at 0.001% (amplitude of 7 μm) to stay in the linear viscoelastic region. The storage modulus (E') and tan δ curves as a function of the temperature, were recorded and analyzed using the TA Instruments Universal Analysis 2000 software. The temperatures Tα (°C) were determined as the temperatures at the peak maximum of the tan δ curves.
Synthesis of Resin Epoxy Precursors
Scheme 1 presents the complete synthetic pathway using the following molecules: ferulic acid (a), ethyl dihydroferulate (b), benzylated ethyl ferulate (c), benzylated glycerol diferulate (GDFoBn) (d), glycerol tri-dihydroferulate (GTF) (e) and lipophilic glycerol dihydroferulates (GDFx) (f, g and h). Compound syntheses were carried out following procedures previously described in the literature (Ménard et al., 2017; Hollande and Domenek, 2018). Full characterizations and detailed procedures for new compounds are given in the Electronic Supplementary Information (ESI). 1H & 13C NMR spectra, FTIR spectrum as well as TGA trace of GTF-EPO can be found in a previous published work (Ménard et al., 2017).
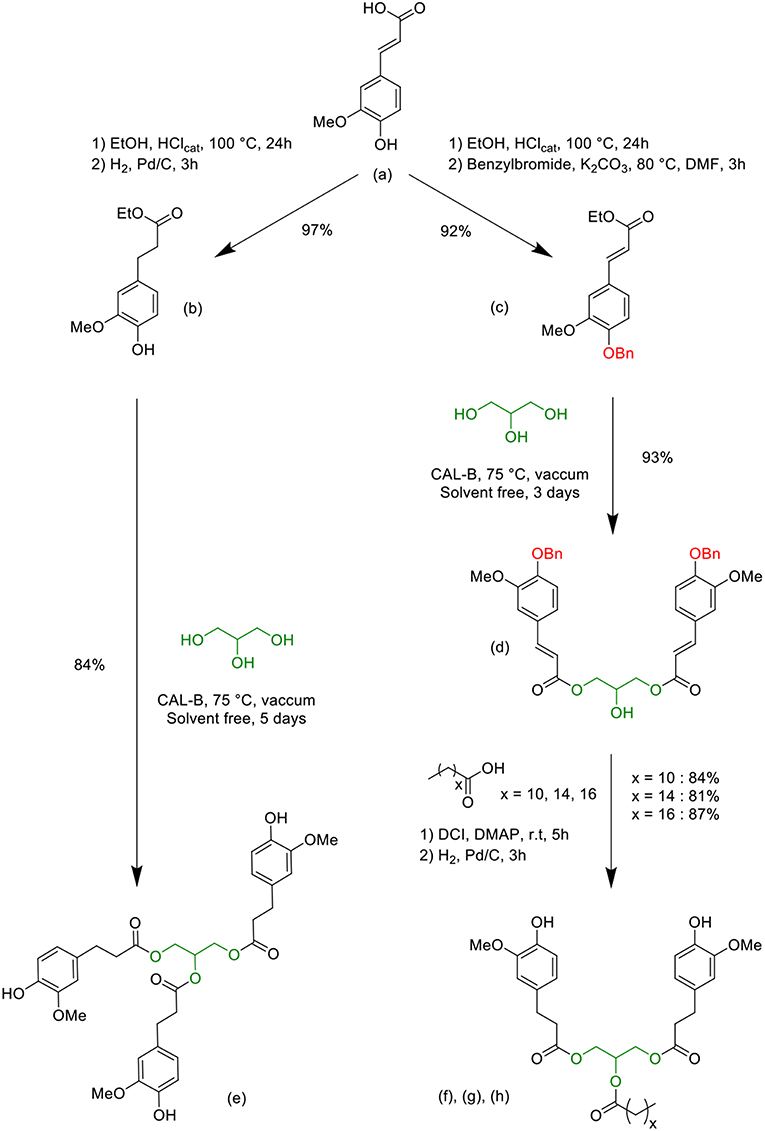
Scheme 1. Syntheses of the bio-based bisphenols (GDFx) (f), (g), (h), and triphenol (GTF) (e) precursors from ferulic acid, glycerol and fatty acids.
Glycidylation
Phenolic precursors (GDFx or GTF; 1 eq) were dissolved in epichlorohydrin (10 eq/OH). Triethyl benzyl chloride (TEBAC) (0.2 eq) was added and the suspension was stirred for 2 h at 80°C. The reaction medium was cooled down to room temperature and NaOH (5M, 2 eq/OH) was added. The biphasic solution was vigorously stirred for 4 h at room temperature then extracted with ethyl acetate (3 × 100 mL). The organic layers were washed with brine (80 mL), dried over anhydrous MgSO4, filtered and concentrated in vacuo.
Epoxide Equivalent Weight (EEW)
Experimental values of EEW for the epoxide resins synthesized herein were determined by ASTM D1652. Samples were dissolved in dichloromethane (DCM) with tetraethylammonium bromide and titrated in triplicate, using perchloric acid solution in acetic acid (1 N). Crystal violet was used as an indicator and the end point was determined when the solution turned from blue to green for longer than 30 s. Detailed results are provided in the ESI.
Preparation of Cured Epoxy Materials
This method considers that the epoxy resins prepared herein contained small amounts of higher molecular weights fractions. The weight of the resins and cross-linkers were determined by using the EEW values and amine hydrogen equivalent weight (AHEW). Weights of reaction components for the formulated resin systems were determined using equation (1). Equations (2) and (3) were used to calculate the AHEW and to obtain the parts by weight of diamine per hundred parts resin (phr).
For instance, if phr = 20, then 20 g of diamine would be needed for 100 g of epoxy resin. Epoxy resins were vigorously mixed with the appropriate amount of crosslinker at room temperature in a disposable aluminum pan. In the case of the solid diamine DA10, an additional stirring time at 120°C was necessary to obtain a complete dissolution of the amine in the epoxy precursor. Mixtures were then transferred into rectangular stainless steel molds (length: 40 mm, width: 8 mm, thickness: 1.5 mm). The method of curing was adapted from a literature procedure (Janvier et al., 2017). In summary, the resins were maintained under ambient conditions in the molds for 2 h and then for 2 h at 60°C, for 20 h at 110°C and for 1 h at 150°C.
Hydrolytic Degradation Assays
Cured resin samples with dimensions about 4 × 8 × 1.5 mm, with weights ranging from 75 to 95 mg, were placed in a 10 mL sealed tube. An aqueous solution of HCl (3M) was added. The vials were heated at 60°C for 40 min for equilibration. Incubations were then continued for 5, 20, 26, 43, and 50 h. The residual solid was then washed with deionized water, dried then weighed to determine the mass loss.
Measurement of Contact Angle
The contact angle (θ) of liquid water against the cured epoxy resins was measured by the sessile drop method at room temperature with a drop shape analysis system (DIGIDROP and Visiodrop software). The contact angle was measured at three different locations for each thermoset and the average value was reported.
Estrogen Binding Affinity Testing
The agonistic and antagonistic potentials of bisphenols were analyzed following a literature method (Delfosse et al., 2012) in which ERα, PXR, and AR transcriptional activities were monitored by using corresponding reporter cells HELN ERα, U2OS AR, and HG5LN PXR cells, respectively. Activities were measured in relative light units (RLU) and 100% activities were assigned to the RLU value obtained with 10 nM agonist control (estradiol (E2), R 1881, SR 12813). The vehicle (DMSO) was tested as a control without any compound.
Results and Discussion
Synthesis of the Bio-Based Epoxy Resin
Chemo-Enzymatic Synthesis
Chemical esterification of p-hydroxycinnamic acids with alcohol is unselective and side reactions generally lead to an unwanted product that needs to be removed by purification steps, generating waste to dispose of. In opposition, the lipase-mediated enzymatic synthesis offers some advantages for synthesizing esters, such as milder reaction conditions and selectivity. Hence, the selective synthesis of the bis/tri-ferulate resin epoxy precursors e, f, g and h, involving glycerol linker group, was based on chemo-enzymatic methods recently published (Scheme 1) (Pion et al., 2013; Hollande and Domenek, 2018).
Ferulic acid was first transformed either in ethyl dihydroferulate (Scheme 1, b) or in benzylated ethyl ferulate (Scheme 1, c), through a two-step one-pot process. Afterwards, a specific affinity of Candida antarctica Lipase B (CAL-B) toward glycerol allowed controlling the degree of functionalization of the latter, giving access to the symmetric bisphenol (Scheme 1, d) or to the fully functionalized glycerol triferulate (Scheme 1, e) in excellent yields and purities. Fatty acids were then grafted onto the available secondary alcohol of the intermediary compound d, immediately followed by a palladium-catalyzed hydrogenation to simultaneously reduce unsaturation and cleave the benzyl group, thus providing the GDFx compounds (Scheme 1, f, g, h. It is noteworthy to mention that all the syntheses described in Scheme 1 have been performed at the multi-gram scale. Moreover, in a recent work, we have not only demonstrated the feasibility of the lipase-mediated transesterification of dihydroferulate ethyl ester at the kilo-scale (Teixeira et al., 2017), but also devised a sustainable and industrially relevant membrane-based technique, allowing both the purification of the targets and the recycling of solvents and unreacted reagents.
Endocrine Receptor Activities of GDFx Bisphenols
Nowadays, the development of new platform chemicals is deeply regulated, especially for bisphenolic compounds—such as BPA—and other controversial endocrine disrupting chemicals (EDCs). Herein, endocrine activities of newly created GDFx bisphenols were investigated by evaluating their ability to interact with three types of receptors ERα, PXR, and AR. ERα is a member of the nuclear hormone receptors family, and its activity is regulated by the steroid estrogen sex hormone 17β-estradiol (E2). PXR is a member of the steroid and xenobiotic sensing nuclear receptors family and AR is a nuclear type androgen receptor that is activated by binding with any of the androgenic hormones. In Figure 1A, sex hormone E2 induces a 100% ERα activity at 5.10−10 M, which plateaus at higher concentrations. For BPA, estrogenic activity was observed at molarities of 10−7 and slightly above 60% at concentrations of 10−5. In contrast, all newly created GDFx bisphenols, GDF10, GDF14, and GDF16, exhibited an activity similar to that of the control, even with increasing concentrations. In addition, Figure 1B reveals that the highest concentration tested for GDFx family (i.e., 10−5 M) did not induce any abnormal receptor activities on both PXR and AR. Finally, an endocrine disruption assay (ESI p22) indicated that phenolic structures were neither agonist nor antagonist ligands. Based on these results, that demonstrated the innocuousness of these newly created GDFx bisphenols, we were encouraged to further explore their use for the production of epoxies and epoxy-amine resins.
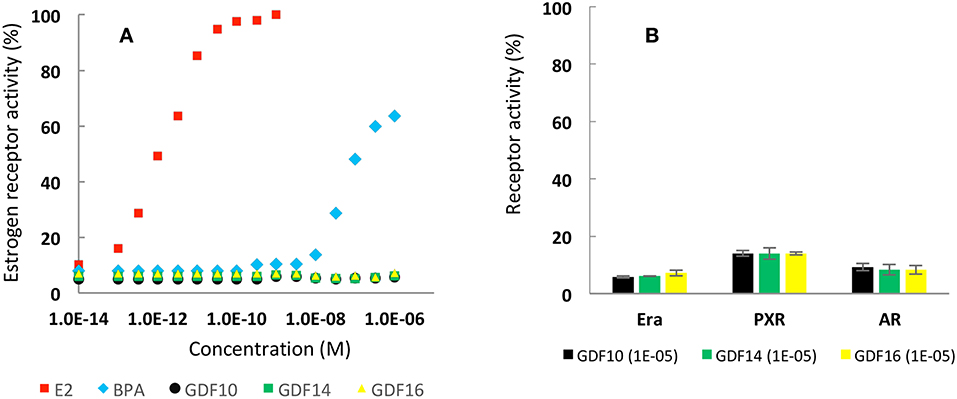
Figure 1. (A) Estrogenic activity (%) as function of concentration of E2, BPA and synthesized bisphenols. (B) Activities (%) of Erα, PXR and AR induced by synthesized bisphenol at 10−5 molarity.
Glycidylation
Epoxy resins synthesis consists in the glycidylation of the different GDFx bisphenols, using the classical procedure, i.e., large excess of epichlorohydrin under alkaline conditions. However, to prevent the partial hydrolysis of the internal ester moieties observed by Maiorana et al. (2016) during classical glycidylation of bisferulate esters, milder temperatures, and shorter reaction times were applied. Thus, to offset this restriction, a catalytic amount of triethylbenzyl ammonium chloride (TEBAC) as phase transfer catalyst—well known for allowing higher epoxy functionality—was used (Fache et al., 2015a). Under such conditions, the phenolate formed is able to attack each one of the carbons of epichlorohydrin (Scheme 2), two of them, (b) and (c), leading to the epoxy ring opening. Thus, a basic treatment is necessary to close the open forms. Yet, not all the resulting species are diepoxies resins. Side reactions can occur, the most important being (b) the oxetane formation or (d) the formation of branched molecules due to the oligomerization of chlorinated intermediate (Ellis, 1993).
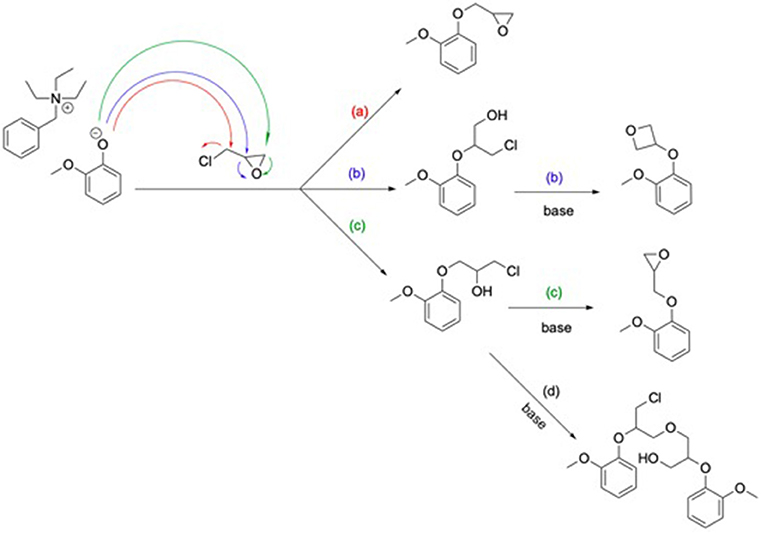
Scheme 2. Glycidylation mechanism by SN2 (a) or epoxy ring opening (c) and oxetane (b) or oligomeric product (d) formation consequent to a basic treatment.
Resulting crude di/triglycidyl ether bis/triferulate epoxy resins, named GDFxEPO, and GTF-EPO, respectively, were obtained in high isolated yields (>85%). All the resins prepared herein (see ESI, p18) showed the appearance of an epoxy vibrational band at 912 cm−1 characteristic of oxirane C-O group on their FT-IR spectra. 1H NMR analyses showed the expected chemical shifts for the proton on the tertiary carbon of the oxirane ring at 3.40 ppm (see ESI, p17). However, signals were also observed between 3.75 and 3.95 ppm, which could be attributed to the oxetane or the oligomeric production epoxies pictured in Scheme 2 (b) and (d). In order to investigate the potential side formation of such oligomeric epoxies, molecular weight distributions of GDFxEPO and GTF-EPO resins were measured by gel permeation chromatography. The results are shown in Figure 2 and the ESI (p20-21).
The distribution of molecular species (Mn) in epoxy resins was analogous for each sample. Experimentally determined values (GPC) described the normalized weight distribution of epoxy systems in two monodisperse fractions according to the Mw/Mn values closed to 1.0. The first corresponds to the monomeric glycidyl ether of GDF10, GDF14, GDF16, and GTF, with Mn of 840, 970, 1,070, and 860 g/mol, respectively, whereas the second corresponds to Mn above 2,000 g/mol, suggesting the formation of oligomeric by-products through the dimerization of chlorinated intermediate depicted in Scheme 2. Nevertheless, the contribution of the high molecular weight tail to the cumulative molecular weight distribution is <5%. Considering the relatively low concentration of by-products estimated through 1H NRM and GPC analyses, we decided to use these epoxy resins directly without further purification. Table 1 displays values of experimental and theoretical epoxide equivalent weights (EEW) where the latter assumes 100% conversion to the corresponding monomeric glycidyl ferulate structures. Experimental EEWs values are 11 to 15% greater than that of the corresponding theoretical values. This result is attributed to the formation of dimers which results in the increase of the EEW of the synthesized resins above the theoretical value. Finally, Table 1 also reports on the thermal behaviors of uncured resins. TGA analysis revealed thermostability (Td 5%) in the range of 311–340°C under an inert atmosphere, and 304–321°C under an oxidative atmosphere. Furthermore, the alkyl chain length does not significantly impact the degradation temperature.
Thermosets Synthesis
The chemical structures of all amine hardeners or curing agents are reported in Figure 3. Two bio-based (DA10 and DIFFA) and one extensively used fossil-based (IPDA), presenting different rigidities, were selected.
To ensure that epoxy resin has a good reactivity toward diamines, a DSC analysis of an equimolar mixture of resins and diamines was carried out (Figure 4). An exothermic peak was observed around 100°C corresponding to the epoxy ring-opening reaction to form the crosslinked polymer. In order to ensure chain mobility during gelation and to obtain optimal crosslinking content, a multistep temperature program was elaborated. Mixtures were first left for 2 h at room temperature followed by two additional hours at 60°C. Formulations were then cured at T ≈ TDSCcrosslinkingpeak (20 h at 110°C), then at T > TDSCcrosslinkingpeak (1 h at 150°C). Each difunctionalized GDFxEPO epoxy precursor was cured in presence of each curing agent leading to 9 formulations (Table 2, thermosets 1–9). In order to tune the properties of these thermosets, and as the formulations with diamines and difunctionalized GDFxEPO (i.e., thermosets 1–9) did not provide materials rigid enough to perform DMA analyses, 1 formulation with GTF-EPO (Table 2, thermoset 15) as well as 5 co-formulations with IPDA, in variable ratios of difunctionalized (GDF14EPO) and tri-functionalized (GTF-EPO) epoxy resin (Table 2, thermosets 10–14) were also prepared.
Characterization of the Thermosets
Thermogravimetric Analyses (TGA)
The thermal stability of cured thermosets was studied by thermal gravimetric analysis (TGA) under nitrogen flow. Td5% was defined as the temperature at which the thermosets lost 5% of its initial mass, Tdmax as the temperature at which the kinetic degradation of the thermoset occurs at the maximum rate and W%char corresponds to the relative amount of stable residue at a high temperature. Table 2 sums up the values for the thermosets prepared and all thermograms are displayed in ESI p23-25. The lowest Td5% was obtained with DIFFA (Table 2; thermosets 4–6), which might be explained by the initiation of the degradation by rupture of the curing agent segment, as reported by Ménard et al. (2017). Overall, higher Td5% and Tdmaxwere measured with IPDA- and DA10-containing resins. Concerning the high temperature stable char content (W%char), values were around 10% for GDFxEPO resins; moreover, the higher the GTF-EPO ratio in epoxy resin system, the higher the char content. This parameter would be particularly interesting for the development of flame-retardant materials.
Differential Scanning Calorimetric Analyses (DSC)
The degree of cure of the resins and glass transition temperatures were both determined through DSC. Analyses of post-cured resins showed no residual exothermic peak above or below the glass transition corresponding to further curing reactions (ESI, p26-28). These results indicate that if additional cure ever occurred during DSC heating, it is below the instrument level of detection. Therefore, consumption of epoxy moieties and concurrent events of cross-linking during the curing cycle adopted herein results in a degree of cure that closely approaches its upper limit. Furthermore, the curing of difunctionalized resins (Table 2, entries 1–9 and 15) with hardeners presenting various rigidities (DA10 < DIFFA < IPDA due to their aliphatic, aromatic, and cyclic configuration), respectively, lead to a restricted range of Tg values (from 3 to 23°C). Hence, the most flexible amine (DA10) provided the lowest Tg values, while the IPDA-containing thermosets led to the highest Tg. Nevertheless, for a given diamine, thermosets exhibit quite similar Tg, meaning that the impact of the diamine on the rigidity of the network outweighs that of the alkyl chain length (Figure 5A), Entries 1–3; 4–6; 7–9).
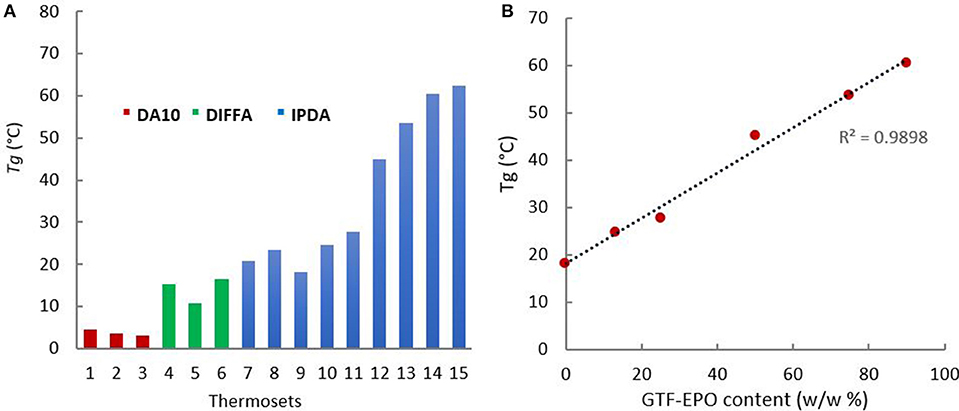
Figure 5. (A) Tg for thermosets formulated with (red) DA10, (green) DIFFA, and (blue) IPDA; (B) Tg as a function of GTF-EPO resin content.
When IPDA reacted with tri-functionalized resins, i.e., GTF-EPO, the Tg value increased up to 60°C due to the higher epoxy functionally (2 vs. 3) and higher cross-link density (Figure 5A, 15). Moreover, as shown in Figure 5B, it is noteworthy to mention that the Tg increased linearly with the tri-functionalized resin GTF-EPO content (R2 = 0.9898).
Dynamic Mechanical Analyses (DMA)
Mechanical properties of the cured resins were evaluated by DMA on each sample with a glass transition upwards of 30°C (thermoset 12–15). Figure 6 and Table 2 provide (a) tan δ values as a function of temperature and (b) storage modulus values at the glassy state at 30°C. Similar to the previous linear relationship observed for Tg and GTF-EPOwt%, the same trend was observed for tan δ that increased with the increasing incorporation of the GTF-EPO to the GDF14EPO system. As discussed previously, the thermal behaviors observed with the addition of GTF-EPO was a consequence of the decrease of EEWs of the co-formulated systems relative to the GDFxEPO resin. In other words, co-formulated resins will lead to thermosets with relatively higher crosslink densities. On the other hand, when the ratio of GDF14EPO in GTF-EPO does not exceed 25 wt%, thermosets exhibit a higher storage modulus than that of the GTF-EPO resin (Figure 6B; entries 13 and 14 vs. 15). It is worth mentioning that the storage modulus at 30°C of epoxy systems which contain 10 wt% of GDF14EPO is 50% higher than that of the GTF-EPO thermoset (2,523 vs. 1,685 MPa). This rather unexpected outcome may be explained by the more efficient interaction/distribution of lipophilic GTFxEPO epoxies during the gelation/curing induced by their “surfactant” properties.
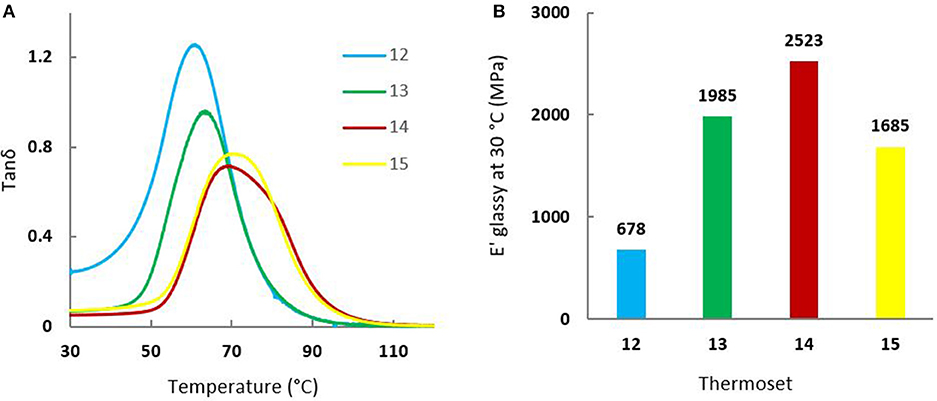
Figure 6. (A) Tan δ curves of (blue) 12, (green) 13, (red) 14, and (yellow) 15 thermosets; (B) Storage modulus (E'glassy) at 30°C of (blue) 12, (green) 13, (red) 14, and (yellow) 15 thermosets.
Degradation and Wettability Behavior
To gain further insight on how surface hydrophobicity varies as a function of the alkyl chain length, contact angles were measured (Table 3). Whatever the curing agent, increasing the alkyl chain length from 12 to 18 carbons (i.e., lauric acid -> palmitic acid -> stearic acid) resulted in an increase of the contact angle (Table 3; thermosets 1 vs. 3, 4 vs. 6, and 7 vs. 9). However, it is worth mentioning that the nature of the curing agent does not significantly impact the hydrophilicity of thermosets as for a same resin GDFxEPO cured with the three different curing agents (i.e., DA10, DIFFA, and IPDA) contact angles differ by < ±7°.
In addition, thermosets weight loss, determined as a function of the incubation time in acidic aqueous solutions, was plotted (Figure 7). Data showed a decrease in mass upon incubation with linear behavior, according to the R2 values for weight loss plots (Table 3). Finally, it is noteworthy to mention that the length of the fatty acid chain grafted onto GDFx epoxies had a significant effect on the hydrolytic degradation rate of cured thermosets. Indeed, an increase in hydrophobicity of the thermoset, by increasing the length of the fatty acids graft, was consistent with a corresponding decrease of the degradation rate measured in mg.h−1 and the susceptibility of that surface to hydrolysis.
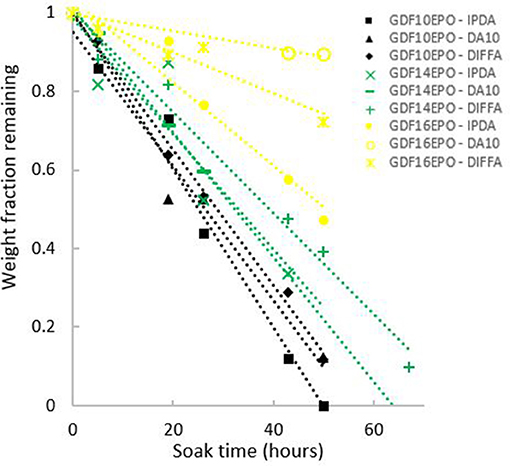
Figure 7. Analyses of thermoset weight loss as function of incubation time at 60°C in acid aqueous solutions.
Conclusion
Herein, the chemo-enzymatic synthesis, thermo-mechanical properties, wettability and acidic hydrolysis of 100% renewable ferulic-, glycerol- and fatty acids-based bis- and triphenols - GDFx and GTF - are described. The estrogenic activity of GDFx with fatty acid chain lengths of 12, 16, and 18-carbons was quantified and compared to bisphenol A and 17β-estradiol and showed no significant activity for ERα, PXR, and AR receptors. Bio-based GDFx and GTF bis/triphenols were then successfully converted to their corresponding di- and triglycidyl ether epoxy resins (i.e., GDFxEPO and GTF-EPO, respectively) through a TEBAC-mediated glycidylation. GDFxEPO was then cured with DIFFA, DA10 and IPDA diamines; GDF14EPO was also co-formulated with tri-functionalized GTF-EPO and IPDA. All the resulting epoxy-amines exhibited relatively high thermostability with Td5% ranging from 282 to 310°C and Tg between 3 and 62°C. Surprisingly, in the case of GDFxEPO-diamine resins, for a given diamine, the chain length of the fatty acid moiety did not significantly impact the Tg. However, one can tailor the Tg by playing with the diamine nature and achieve Tg up to 23°C with IPDA and GDF14EPO. To further tailor the Tg and to reach higher values, GTF-EPO must be added to the formulation. In such formulations, DSC and DMA analyses showed that the Tg and the storage modulus can also be modulated by finely adjusting the GTF-EPO content. Finally, with regards to wettability and degradability, the chain length of the fatty acid was found to provide a simple but powerful approach to tailor the wettability and the susceptibility to hydrolysis of the GDFxEPO-based epoxy-amine resins. Indeed, the shortest fatty acid provided the highest wetting and hydrolysis rate, and vice-versa. This study therefore demonstrates the great potential of combining ferulic acid, glycerol and fatty acids using chemo-enzymatic processes for the preparation of epoxies and epoxy-amine networks with tunable properties.
Author Contributions
FA and RG conceived the research. FA and SD managed the research. LH and ID performed the chemo-enzymatic reactions and the characterizations. PB performed the endocrine disruption assays. FA and LH provided the technical guidelines, reviewed the results, wrote, and drafted the article. FA, RG, and SD reviewed and approved the article.
Conflict of Interest Statement
The authors declare that the research was conducted in the absence of any commercial or financial relationships that could be construed as a potential conflict of interest.
Acknowledgments
The authors are grateful to Région Grand Est, Conseil Départemental de la Marne and Grand Reims for financial support.
Supplementary Material
The Supplementary Material for this article can be found online at: https://www.frontiersin.org/articles/10.3389/fchem.2019.00159/full#supplementary-material
References
Auvergne, R., Caillol, S., David, G., Boutevin, B., and Pascault, J.-P. (2014). Biobased thermosetting epoxy: present and future. Chem. Rev. 114, 1082–1115. doi: 10.1021/cr3001274
Barde, M., Adhikari, S., Via, B. K., and Auad, M. L. (2018). Synthesis and characterization of epoxy resins from fast pyrolysis bio-oil. Green Mater. 6, 76–84. doi: 10.1680/jgrma.17.00038
Biermann, U., Friedt, W., Lang, S., Lühs, W., Machmüller, G., Metzger, J. O., et al. (2000). New syntheses with oils and fats as renewable raw materials for the chemical industry. Angew. Chemie Int. Ed. 39, 2206–2224. doi: 10.1002/1521-3773(20000703)39:13<2206::AID-ANIE2206>3.0.CO;2-P
Bruins, P. F. (1968). “Epoxy resin technology,” in Interscience, ed P. F. Bruins (New York, NY), 275.
Celikbag, Y., Meadows, S., Barde, M., Adhikari, S., Buschle-Diller, G., Auad, M. L., et al. (2017). Synthesis and characterization of bio-oil-based self-curing epoxy resin. Ind. Eng. Chem. Res. 56, 9389–9400. doi: 10.1021/acs.iecr.7b02123
Delfosse, V., Grimaldi, M., Pons, J.-L., Boulahtouf, A., le Maire, A., Cavailles, V., et al. (2012). Structural and mechanistic insights into bisphenols action provide guidelines for risk assessment and discovery of bisphenol A substitutes. Proc. Natl. Acad. Sci. U.S.A. 109, 14930–14935. doi: 10.1073/pnas.1203574109
Fache, M., Auvergne, R., Boutevin, B., and Caillol, S. (2015a). New vanillin-derived diepoxy monomers for the synthesis of biobased thermosets. Eur. Polym. J. 67, 527–538. doi: 10.1016/j.eurpolymj.2014.10.011
Fache, M., Boutevin, B., and Caillol, S. (2015b). Vanillin production from lignin and its use as a renewable chemical. ACS Sustain. Chem. Eng. 4, 35–46. doi: 10.1021/acssuschemeng.5b01344
Feghali, E., Torr, K. M., van de Pas, D. J., Ortiz, P., Vanbroekhoven, K., Eevers, W., et al. (2018). Thermosetting polymers from lignin model compounds and depolymerized lignins. Top. Curr. Chem. 376, 1–25. doi: 10.1007/s41061-018-0211-6
Gojny, F. H., Wichmann, M. H. G., Fiedler, B., Kinloch, I. A., Bauhofer, W., Windle, A. H., et al. (2006). Evaluation and identification of electrical and thermal conduction mechanisms in carbon nanotube/epoxy composites. Polymer 47, 2036–2045. doi: 10.1016/j.polymer.2006.01.029
Hernandez, E. D., Bassett, A. W., Sadler, J. M., La Scala, J. J., and Stanzione, J. F. (2016). synthesis and characterization of bio-based epoxy resins derived from vanillyl alcohol. ACS Sustain. Chem. Eng. 4, 4328–4339. doi: 10.1021/acssuschemeng.6b00835
Holbery, J., and Houston, D. (2006). Natural-fiber-reinforced polymer composites in automotive applications. JOM 58, 80–86. doi: 10.1007/s11837-006-0234-2
Hollande, L., and Domenek, S. (2018). Chemo-enzymatic synthesis of renewable sterically-hindered phenolic antioxidants with tunable polarity from lignocellulose and vegetal oil components. Int. J. Mol. Sci. 19:3358. doi: 10.3390/ijms19113358
Isikgor, F. H., and Becer, C. R. (2015). Lignocellulosic biomass: a sustainable platform for the production of bio-based chemicals and polymers. Polym. Chem. 6, 4497–4559. doi: 10.1039/C5PY00263J
Janvier, M., Hollande, L., Jaufurally, A. S., Pernes, M., Ménard, R., Grimaldi, M., et al. (2017). Syringaresinol: a renewable and safer alternative to bisphenol A for epoxy-amine resins. ChemSusChem 10, 738–746. doi: 10.1002/cssc.201601595
Jiang, H., Sun, L., Zhang, Y., Meng, F., Zhang, W., and Zhao, C. (2018). Estrogenic activity research of a novel fluorinated bisphenol and preparation of an epoxy resin as alternative to bisphenol A epoxy resin. Eur. Polym. J. 108, 507–516. doi: 10.1016/j.eurpolymj.2018.09.020
Laurichesse, S., Huillet, C., and Avérous, L. (2014). Original polyols based on organosolv lignin and fatty acids: new bio-based building blocks for segmented polyurethane synthesis. Green Chem. 16, 3958–3970. doi: 10.1039/C4GC00596A
Maiorana, A., Reano, A. F., Centore, R., Grimaldi, M., Balaguer, P., Allais, F., et al. (2016). Structure property relationships of biobased: N -alkyl bisferulate epoxy resins. Green Chem. 18, 4961–4973. doi: 10.1039/C6GC01308B
Maiorana, A., Spinella, S., and Gross, R. A. (2015). Bio-based alternative to the diglycidyl ether of bisphenol A with controlled materials properties. Biomacromolecules 16, 1021–1031. doi: 10.1021/acs.biomac.5b00014
Maisonneuve, L., Lebarbé, T., Grau, E., and Cramail, H. (2013). Structure–properties relationship of fatty acid-based thermoplastics as synthetic polymer mimics. Polym. Chem. 4, 5472–5517. doi: 10.1039/c3py00791j
Mauck, J. R., Yadav, S. K., Sadler, J. M., La Scala, J. J., Palmese, G. R., Schmalbach, K. M., et al. (2017). Preparation and characterization of highly bio-based epoxy amine thermosets derived from lignocellulosics. Macromol. Chem. Phys. 218:1700013. doi: 10.1002/macp.201700013
Meier, M. A. R. (2018). Plant-oil-based polyamides and polyurethanes: toward sustainable nitrogen-containing thermoplastic materials. Macromol. Rapid Commun. 40:1800524. doi: 10.1002/marc.201800524
Ménard, R., Caillol, S., and Allais, F. (2017). Ferulic acid-based renewable esters and amides-containing epoxy thermosets from wheat bran and beetroot pulp: chemo-enzymatic synthesis and thermo-mechanical properties characterization. Ind. Crops Prod. 95, 83–95. doi: 10.1016/j.indcrop.2016.10.016
Meylemans, H. A., Groshens, T. J., and Harvey, B. G. (2011). Synthesis of renewable bisphenols from creosol. ChemSusChem 5, 206–210. doi: 10.1002/cssc.201100402
Nicastro, K. H., Kloxin, C. J., and Epps, T. H. (2018). Potential lignin-derived alternatives to bisphenol a in diamine-hardened epoxy resins. ACS Sustain. Chem. Eng. 6, 14812–14819. doi: 10.1021/acssuschemeng.8b03340
Pandey, M. P., and Kim, C. S. (2011). Lignin depolymerization and conversion: a review of thermochemical methods. Chem. Eng. Technol. 34, 29–41. doi: 10.1002/ceat.201000270
Pion, F., Reano, A. F., Ducrot, P.-H., and Allais, F. (2013). Chemo-enzymatic preparation of new bio-based bis- and trisphenols: new versatile building blocks for polymer chemistry. RSC Adv. 3:8988. doi: 10.1039/c3ra41247d
Prolongo, S. G., Gude, M. R., Sanchez, J., and Ureña, A. (2009). Nanoreinforced epoxy adhesives for aerospace industry. J. Adhes. 85, 180–199. doi: 10.1080/00218460902881766
Ramon, E., Sguazzo, C., and Moreira, P. (2018). A review of recent research on bio-based epoxy systems for engineering applications and potentialities in the aviation sector. Aerospace 5:110. doi: 10.3390/aerospace5040110
Savonnet, E., Grau, E., Grelier, S., Defoort, B., and Cramail, H. (2018). Divanillin-based epoxy precursors as DGEBA substitutes for biobased epoxy thermosets. ACS Sustain. Chem. Eng. 6, 11008–11017. doi: 10.1021/acssuschemeng.8b02419
Shuai, L., Amiri, M. T., Questell-Santiago, Y. M., Héroguel, F., Li, Y., Kim, H., et al. (2016). Formaldehyde stabilization facilitates lignin monomer production during biomass depolymerization. Science 354, 329–333. doi: 10.1126/science.aaf7810
Strebelle, M., Gilbeau, P., and Catinat, J.-P. (2001). Epichlorohydrin-Based Product and Process for Manufacturing This Product.
Teixeira, A. R. S., Willig, G., Couvreur, J., Flourat, A. L., Peru, A. A. M., Ferchaud, P., et al. (2017). From bench scale to kilolab production of renewable ferulic acid-based bsiphenols: optimisation and evaluation of different purification approaches towards a technical feasibility and process environmental sustainability. React. Chem. Eng. 2, 406–419. doi: 10.1039/C7RE00017K
Wan, J., Gan, B., Li, C., Molina-Aldareguia, J., Kalali, E. N., Wang, X., et al. (2016). A sustainable, eugenol-derived epoxy resin with high biobased content, modulus, hardness and low flammability: synthesis, curing kinetics and structure–property relationship. Chem. Eng. J. 284, 1080–1093. doi: 10.1016/j.cej.2015.09.031
Wang, S., Bassett, A. W., Wieber, G. V., Stanzione, J. F., and Epps, T. H. (2017). Effect of methoxy substituent position on thermal properties and solvent resistance of lignin-inspired poly(dimethoxyphenyl methacrylate)s. ACS Macro Lett. 6, 802–807. doi: 10.1021/acsmacrolett.7b00381
Keywords: ferulic acid, glycerol, lipase, bio-based thermosets, wettability, degradability
Citation: Hollande L, Do Marcolino I, Balaguer P, Domenek S, Gross RA and Allais F (2019) Preparation of Renewable Epoxy-Amine Resins With Tunable Thermo-Mechanical Properties, Wettability and Degradation Abilities From Lignocellulose- and Plant Oils-Derived Components. Front. Chem. 7:159. doi: 10.3389/fchem.2019.00159
Received: 28 November 2018; Accepted: 04 March 2019;
Published: 27 March 2019.
Edited by:
Gil Garnier, Bioresource Processing Institute of Australia (BioPRIA), AustraliaReviewed by:
Guillermo Javier Copello, Consejo Nacional de Investigaciones Científicas y Técnicas (CONICET), ArgentinaJuan Carlos Serrano-Ruiz, Universidad Loyola Andalucía, Spain
Copyright © 2019 Hollande, Do Marcolino, Balaguer, Domenek, Gross and Allais. This is an open-access article distributed under the terms of the Creative Commons Attribution License (CC BY). The use, distribution or reproduction in other forums is permitted, provided the original author(s) and the copyright owner(s) are credited and that the original publication in this journal is cited, in accordance with accepted academic practice. No use, distribution or reproduction is permitted which does not comply with these terms.
*Correspondence: Florent Allais, florent.allais@agroparistech.fr