- College of Chemistry, Chemical Engineering and Biotechnology, Donghua University, Shanghai, China
A large-area MnO2 stalagmite nanorod arrays (SNAs) growing vertically on flexible substrates were successfully fabricated by an easy heat-electrodeposition method. The large specific capacitance (646.4 F g−1 at 500 mA g−1) and excellent rate capability (42.3% retention with 40 times of increase) indicate that the prepared MnO2 SNAs flexible electrode has outstanding electrochemical performance. Furthermore, after 5,000 repetitions of CV tests, the overall specific capacitance could retain ~101.2% compared with the initial value meant a long cycling life. These outstanding properties could be ascribed to the effective conductive transport path between Ni substrate and MnO2 nanorods, and owing to the stalagmite like structure of MnO2 nanorods, the exposed sufficient active sites are beneficial to the electrolyte infiltration.
Introduction
The expanding requirement for energy consumption has stimulated the development of electrochemical energy storage devices (Simon and Gogotsi, 2008). Supercapacitors (SCs) occupy an important position in the field of energy storage devices due to their high power density, high-speed of charging and discharging and long cycle life (Burke, 2000). Nowadays, the development of supercapacitors to higher mechanical flexibility has become one of the important trends. It is shown that materials, such as graphene, transition metal dichalcogenides, MXenes (Han et al., 2018), and nanocellulose-graphene composites (Xing et al., 2019) could be used to construct nanomaterials-based flexible electrodes with high performance for supercapacitors, and there were relationships between structures and properties.
There are many different SCs electrode materials, such as carbon-based material (Li G. et al., 2018; Takeuchi et al., 2018) for the electric double layer capacitors (EDLCs), transition metal oxides, nitrides (Yi et al., 2018) and nickel based materials (Feng et al., 2014; Li et al., 2018) for the pseudocapacitors (PCs). Manganese dioxide (MnO2) is one of the rapid developed metal oxide electrode materials in recent years (Wang et al., 2015). α-MnO2 has a high specific capacitance among the various crystallographic structures of MnO2, which is mainly due to its largest tunnel (Sanger et al., 2016) that can store more foreign cations for charge balance. More importantly, the electrolytes used for MnO2 electrodes are non-corrosive neutral solutions, which is green and fit for environmental protection (Li et al., 2012).
As we know, the effects of active materials on the performance of SCs include morphology, structure, contact with collector plate and active sites, etc. Unfortunately, during the redox reaction, the electrons transport of MnO2 electrodes often restricted by its high electrical resistivity. In addition, the defect of the conventional powder electrode preparation is that the generated 'dead volume' by the bonding process of the active material to substrate could make a deterioration in the electrochemical properties of the electrodes (Liu et al., 2016). To solve the defects noted above, it could be a feasible way to manufacture binder-free MnO2 supporting on 3D conductive substrates. The benefits of the 3D binder-free electrode are the enhanced electron transport and sufficient free space between the nanostructures, which contribute to the generation of more active sites for Faradaic reaction. Li et al. prepared the Ni foam-based ultrafine MnO2 nanobelts with capacitance of 509 F g−1 at 0.2 A g−1 (Li et al., 2013). Davoglio et al. (2018) synthetized the α-MnO2 particles at the aqueous-organic interface with capacitance of 289 F g−1 at 0.5 A g−1. Although some achievements have been made in the preparation of MnO2 electrodes on conductive substrates, there is still great potential to develop easy methods for the preparation of high performance MnO2 nanowires with various forms.
In this paper, the heat-electrodeposition method was adopted to formulate a nanoarrays of stalagmite like MnO2 on the flexible substrates. The prepared electrode gets capacitance of 646.4 F g−1 (500 mA g−1) and 42.3% retention (current density increased 40 times) for a remarkable rate capability. And the total capacitance retention rate after 5,000 cycles is ~101.2%. Furthermore, to verify the generality of the synthesis method, another flexible activated carbon fiber (ACF) was also used as the substrate for the growth of MnO2 nanoarrays.
Experimental
Material Preparation
The preparing method in detail was: the heat-electrodeposition process carried on a 3D porous Ni foam. Before electrodeposition, the Ni foam was cut into ~ 3 × 1 cm2, and then immersed into a 5 mol/L HCl solutions along with supersonic wave treatment for 10 min to dissolve the NiO layer on the surface. The Ni foam obtained from the previous step was rinsed to neutral with distilled water, and then subjected to vacuum drying (60°C, 4 h). The heat-electrodeposition occurred in a cell with the water bath. The composition of the electrolyte was as follows: Mn(CH3COO)2 (0.01 M), CH3COONH4 (0.02 M) and dimethylsulfoxide (DMSO, 10 vol.%). The corresponding working electrode, the counter electrode and the reference electrode were the treated Ni foam, the Pt plate (1.5 × 1.5 cm2) and saturated calomel electrode (SCE), respectively. The heat-electrodeposition condition was applied at a constant current (0.5 mA cm−2) by the Autolab electrochemical workstation at ~ 80°C for 60 min. After that rinsed the obtained sample to neutral and placed it in a 60°C vacuum dryer for 4 h. Finally, the sample was calcined in N2 atmosphere (heating-up 0.5°C min−1, 250°C, 2 h). The weight gain of the sample after the deposition was the active matter weight.
Material Characterizations
In order to analyze the samples qualitatively, the X-ray diffractometer (XRD; Rigaku D/max-2550 PC, Cu-Kα radiation) spectrum was utilized. To observe the microstructures, scanning electron microscope (SEM, Hitachi S-4800) and transmission electron microscope (TEM, JEOL JEM-2100F) were adopted. Mass weighing (Mettler Toledo XS105DU, δ = 0.01 mg).
Electrochemical Measurements
The Electrochemical Workstation (Autolab PGSTAT302N, electrolyte 0.5 M Na2SO4) was used to measure electrochemical performance. In the test, the obtained MnO2 electrode (~1 cm2) was used as the working electrode. The counter and reference electrode were the same as mentioned above. Specific capacitance calculation (Guan et al., 2017):
where C (F g−1) is the specific capacitance, I (A) is the discharge current, Δt (s) is the discharge time consumed in the potential range of ΔV, ΔV (V) is the potential window, m (g) is the mass of the active materials.
The weight of the 1 cm2 MnO2 electrode was ~ 1.32 mg. The cyclic voltammetry (CV) potential window was −0.1 to 0.9 V. The scan rates increased from 1 to 100 mV s−1. The galvanostatic charge-discharge (GCD) curves were measured under current densities from 0.5 to 20 A g−1. The cycle life was obtained by CV test (50 mV s−1) with repetitions of 5,000.
Results and Discussions
Except for the two strong peaks of 3D Ni foam substrate, the XRD diffraction pattern in Figure 1 shows that other peaks at 12.8, 28.8, 36.7, 37.5, 56.4, 60.3, 65.1, and 69.7°, which are characteristic (110), (310), (400), (211), (600), (521), (002), and (541) reflections of α-MnO2 (JCPDS 44-0141, a = b = 9.785 Å and c = 2.863 Å), respectively.
An aligned and dense MnO2 nanoarray is presented in Figure 2a and there are no macroscopic defects among them. The enlarged SEM image of Figure 2b shows a highly open structure was formed by a vertical MnO2 arrays on the substrate, which is conducive to the full entry of electrolytes. The TEM characterization was performed in order to get more structural information of the MnO2 arrays. Figure 2c is a single stalagmite like MnO2 nanorod which appears to be truncated cone-shaped with burrs on its surface. The typical diameters are ~30 nm of the root and ~80 nm of the top, and the length is up to ~180 nm. HRTEM image of the single MnO2 nanorod edge (Figure 2d) suggests that the surface is clearly with uniform single crystal and the interplanar spacing of 0.28 nm matches the (001) lattice plane of α-MnO2 crystal. In addition, FFT diffraction pattern can also be associated with [100] zone axis of α-MnO2 crystal (inset of Figure 2d).
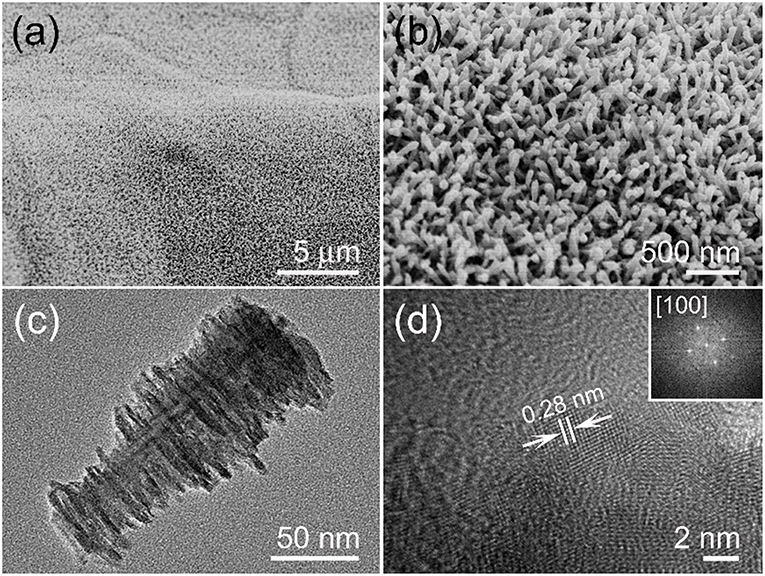
Figure 2. (a) Low and (b) enlarged SEM photos of MnO2 nanoarrays. (c) TEM image of the stalagmite MnO2 nanorod. (d) Lattice resolved HRTEM image of a MnO2 nanorod, an upper right inset showing its corresponding FFT pattern.
In order to understand how the unique structure of stalagmite MnO2 nanorod arrays (MnO2 SNAs) was formed, the time-dependent electrodepositing experiments were carried out through controlling the electrodeposit reaction time. Figure 3a shows that after deposition of 2 min, nanoparticles can be seen on the surface of the substrate with the diameters of 10–20 nm. When the reaction time increases to 20 min, lots of well-distributed cylindrical nanorods began to appear, as displayed in Figure 3b. After doubling the reaction time, some irregular burrs were observed around the increased nanorods (Figure 3c). As shown in Figure 3d, after 60 min of the electrodeposition, it is found that large-scale and uniform nanorod arrays were formed. The change of the morphology of the samples after different electrochemical deposition time could help us estimate the formation process of the MnO2 nanorod arrays. In the early stage of the reaction, the formation of the nucleus in the precursor solution and the 1D growth behavior of Mn2+ (Ding et al., 2011) occurs successively, which result in the deposition of some tiny nanorods randomly and fast all over the surface. As the deposition intensifies, nanoparticles develop into nanorods with burrs, similar to stalagmite. When the reaction time reached to 1 h, the nanorods presented a truncated cone-shaped and formed a high-density array. The formation process of MnO2 SNAs was elucidated in Figure 3e.
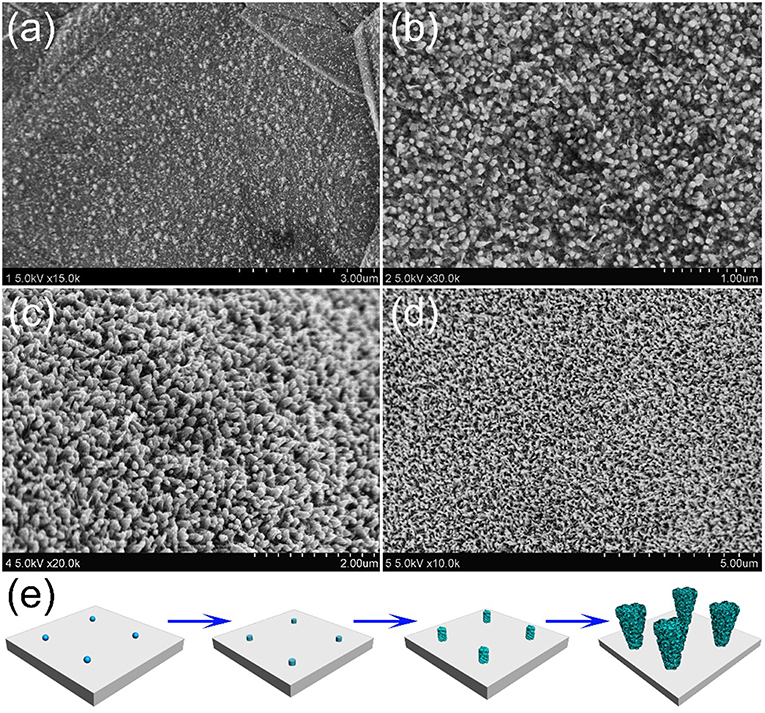
Figure 3. SEM images of the stalagmite MnO2 nanorod arrays for different electrodepositing time: (a) 2 min, (b) 20 min, (c) 40 min, and (d) 60 min; (e) Schematic diagram of the MnO2 SNAs formation.
Furthermore, to verify the generality of the synthesis method, another flexible ACF substrate was chosen to replace the Ni foam. Figure S1 shows that the dense needle-like MnO2 nanorod arrays were grown on the ACF, which proves that the heat-electrodeposition method could be used to fabricate electrodes with different flexible substrates.
Figure 4A shows that the MnO2 SNAs electrode CV with scanning rate from 1 to 100 mV s−1 were approximate to rectangle and symmetrical. All CV curves feature exhibits the pseudocapacitive nature (Figure S3) and a high-speed charge and discharge process (Hu et al., 2016). The possible reason for this phenomenon could be that the active sites in the microstructures of the stalagmite MnO2 SNAs were fully in contact with electrolytes. The symmetrical GCD curves of the MnO2 SNAs shown in Figure 4B revealed that the reversible redox reaction and good electrochemical capacitance characteristics of the system. In addition, by comparing the specific capacitance of the Ni foam substrate and the prepared MnO2 SNAs electrode in Figure S2, it is found that the substrate has little effect on the capacitance value.
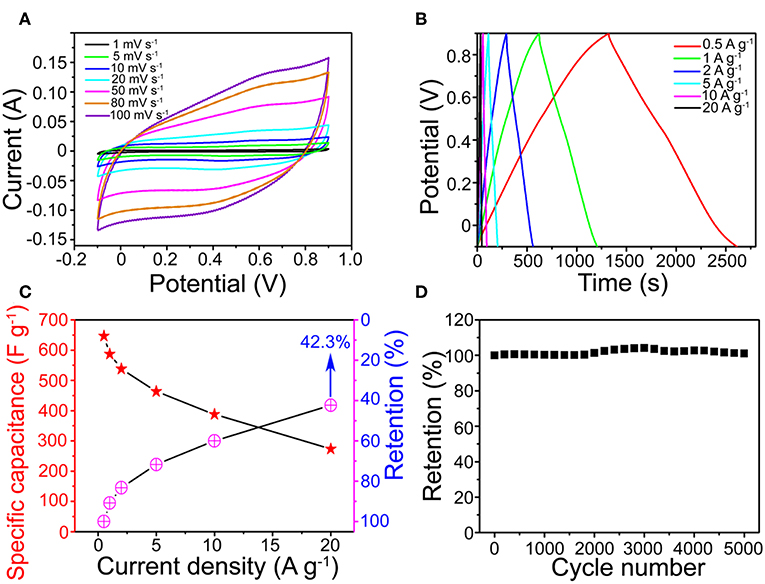
Figure 4. (A) CV and (B) GCD curves of the MnO2 SNAs electrode. (C) Specific capacitance and retention of the MnO2 SNAs electrode. (D) CV cycling performance.
In Figure 4C, the calculated specific capacitance of the electrode were 646.4, 587.1, 538.1, 463.6, 387.6, and 273.6 F g−1 (Red Star), respectively, which were higher than reported works with similar MnO2 structure (Li et al., 2013; Wang et al., 2017; Davoglio et al., 2018). Figure 4C also shows that the capacitance retention increased with the increase of current density. When it increased 40 times from 0.5 to 20 A g−1, the corresponding specific capacitance reduced from 646.4 to 273.6 F g−1 with the retention of 42.3%. The excellent rate capability indicates the potential of the MnO2 SNAs electrode in high-power applications, and it could be caused by the unique open structure. The advantages of the open structure are that it is favorable for electrolyte infiltration and it owns large electrolytic accessible area, which not only promote redox reaction but also facilitates intercalation and de-intercalation of active species.
Figure 4D shows that a beneficial cycle life of the electrode and the capacitances retention enlarged during the initial 3,000 loops. The possible explanation is that the initially inactive part of the MnO2 SNAs electrode was activated by the permeated electrolyte and then the specific capacitance is increased (Xia et al., 2012).
After 5,000 cycles, a high specific capacitance retention of 101.2% indicated that the cyclical stability of the MnO2 SNAs electrode was good. The strong contact between the active matters and the substrate facilitating the collection and enhancement of the electron participation reaction could be an important reason for the effective cycle stability. Thus, it is concluded that the electrode material of the as-synthesized MnO2 SNAs demonstrates an excellent cycle life.
Conclusion
In conclusion, the stalagmite MnO2 nanorod arrays successfully grew on the flexible substrate by heat-electrochemical deposition method. The prepared MnO2 SNAs electrode has the high specific capacitance, the outstanding rate capability and the long cycle life, all of which all suggest its excellent electrochemical performance. In addition, this approach could pave the way for a facile low-temperature heat synthetic route for generating a variety of metal oxides arrays flexible substrate electrode.
Data Availability
The raw data supporting the conclusions of this manuscript will be made available by the authors, without undue reservation, to any qualified researcher.
Author Contributions
YG did the experiments and described the images of figures. XW helping with writing. TZ was the supervisor of this research work. All authors participated in the analysis of experimental data and manuscript preparation.
Funding
This work was supported by National Key R&D Program (2017YFB0309100) from Ministry of Science and Technology of the People's Republic of China.
Conflict of Interest Statement
The authors declare that the research was conducted in the absence of any commercial or financial relationships that could be construed as a potential conflict of interest.
Supplementary Material
The Supplementary Material for this article can be found online at: https://www.frontiersin.org/articles/10.3389/fchem.2019.00338/full#supplementary-material
References
Burke, A. (2000). Ultracapacitors: why, how, and where is the technology. J. Power Sources 91, 37–50. doi: 10.1016/S0378-7753(00)00485-7
Davoglio, R. A., Cabello, G., Marco, J. F., and Biaggio, S. R. (2018). Synthesis and characterization of α-MnO2 nanoneedles for electrochemical supercapacitors. Electrochim. Acta 261, 428–435. doi: 10.1016/j.electacta.2017.12.118
Ding, S., Zhu, T., Chen, J. S., Wang, Z., Yuan, C., and Lou, X. W. (2011). Controlled synthesis of hierarchical NiO nanosheet hollow spheres with enhanced supercapacitive performance. J. Mater. Chem. 21, 6602–6606. doi: 10.1039/c1jm00017a
Feng, L., Zhu, Y., Ding, H., and Ni, C. (2014). Recent progress in nickel based materials for high performance pseudocapacitor electrodes. J. Power Sources 267, 430–444. doi: 10.1016/j.jpowsour.2014.05.092
Guan, B. Y., Yu, L., Wang, X., Song, S., and Lou, X. W. D. (2017). Formation of onion-like NiCo2S4 particles via sequential ion-exchange for hybrid supercapacitors. Adv. Mater. Deerfield Beach Fla 29:1605051. doi: 10.1002/adma.201605051
Han, Y., Ge, Y., Chao, Y., Wang, C., and Wallace, G. G. (2018). Recent progress in 2D materials for flexible supercapacitors. J. Energy Chem. 27, 57–72. doi: 10.1016/j.jechem.2017.10.033
Hu, J., Qian, F., Song, G., Li, W., and Wang, L. (2016). Ultrafine MnO2 nanowire arrays grown on carbon fibers for high-performance supercapacitors. Nanoscale Res. Lett. 11:469. doi: 10.1186/s11671-016-1693-1
Li, G., Gao, X., Wang, K., and Cheng, Z. (2018). Porous carbon nanospheres with high EDLC capacitance. Diam. Relat. Mater. 88, 12–17. doi: 10.1016/j.diamond.2018.06.010
Li, W., Liu, Q., Sun, Y., Sun, J., Zou, R., Li, G., et al. (2012). MnO2 ultralong nanowires with better electrical conductivity and enhanced supercapacitor performances. J. Mater. Chem. 22, 14864–14867. doi: 10.1039/c2jm33368f
Li, W., Xu, K., An, L., Jiang, F., Zhou, X., Yang, J., et al. (2013). Effect of temperature on the performance of ultrafine MnO2 nanobelt supercapacitors. J. Mater. Chem. A 2, 1443–1447. doi: 10.1039/C3TA14182A
Li, W., Zhang, B., Lin, R., Ho-Kimura, S., He, G., Zhou, X., et al. (2018). A dendritic nickel cobalt sulfide nanostructure for alkaline battery electrodes. Adv. Funct. Mater. 28:1705937. doi: 10.1002/adfm.201705937
Liu, X., Chen, G., Guan, H., Dong, C., Xiao, X., and Wang, Y. (2016). Binder-free NiO@MnO2 core-shell electrode: rod-like NiO core prepared through corrosion by oxalic acid and enhanced pseudocapacitance with sphere-like MnO2 shell. Electrochim. Acta 189, 83–92. doi: 10.1016/j.electacta.2015.12.076
Sanger, A., Kumar, A., Kumar, A., and Chandra, R. (2016). Highly sensitive and selective hydrogen gas sensor using sputtered grown Pd decorated MnO2 nanowalls. Sens. Actuators B Chem. 234, 8–14. doi: 10.1016/j.snb.2016.04.152
Simon, P., and Gogotsi, Y. (2008). Materials for electrochemical capacitors. Nat. Mater. 7, 845–854. doi: 10.1038/nmat2297
Takeuchi, K., Fujishige, M., Ishida, N., Kunieda, Y., Kato, Y., Tanaka, Y., et al. (2018). High porous bio-nanocarbons prepared by carbonization and NaOH activation of polysaccharides for electrode material of EDLC. J. Phys. Chem. Solids 118, 137–143. doi: 10.1016/j.jpcs.2018.02.050
Wang, J.-G., Kang, F., and Wei, B. (2015). Engineering of MnO2-based nanocomposites for high-performance supercapacitors. Prog. Mater. Sci. 74, 51–124. doi: 10.1016/j.pmatsci.2015.04.003
Wang, Y., Fu, A., Liu, X., Wang, Y., Li, Y., Guo, P., et al. (2017). Porous carbon directed growth of carbon modified MnO2 porous spheres for pseudocapacitor applications. J. Alloys Compd. 717, 341–349. doi: 10.1016/j.jallcom.2017.05.035
Xia, X., Tu, J., Zhang, Y., Wang, X., Gu, C., Zhao, X., et al. (2012). High-quality metal oxide core/shell nanowire arrays on conductive substrates for electrochemical energy storage. ACS Nano 6, 5531–5538. doi: 10.1021/nn301454q
Xing, J., Tao, P., Wu, Z., Xing, C., Liao, X., and Nie, S. (2019). Nanocellulose-graphene composites: a promising nanomaterial for flexible supercapacitors. Carbohydr. Polym. 207, 447–459. doi: 10.1016/j.carbpol.2018.12.010
Keywords: large-area, manganese dioxide, stalagmite nanorod arrays, supercapacitors, flexible electrode
Citation: Ge Y, Wang X and Zhao T (2019) Preparation of Flexible Substrate Electrode for Supercapacitor With High-Performance MnO2 Stalagmite Nanorod Arrays. Front. Chem. 7:338. doi: 10.3389/fchem.2019.00338
Received: 09 April 2019; Accepted: 25 April 2019;
Published: 14 May 2019.
Edited by:
Wenyao Li, Shanghai University of Engineering Sciences, ChinaReviewed by:
Bo Li, Shanghai Jiao Tong University, ChinaGuangjin Wang, Hubei Engineering University, China
Copyright © 2019 Ge, Wang and Zhao. This is an open-access article distributed under the terms of the Creative Commons Attribution License (CC BY). The use, distribution or reproduction in other forums is permitted, provided the original author(s) and the copyright owner(s) are credited and that the original publication in this journal is cited, in accordance with accepted academic practice. No use, distribution or reproduction is permitted which does not comply with these terms.
*Correspondence: Tao Zhao, dHpoYW9AZGh1LmVkdS5jbg==