- Department of Chemistry, School of Science, Tokyo Institute of Technology, Tokyo, Japan
The visible-light responsive Cu(I)-complex photosensitizers were developed by introducing various aromatic substituents at the 4,7-positions of a 2,9-dimethyl-1,10-phenanthroline (dmp) ligand in a heteroleptic CuI(dmp)(DPEphos)+-type complexes (DPEphos = [2-(diphenylphosphino)phenyl]ether) for photocatalytic CO2 reduction. Introducing biphenyl groups (Bp-) on the dmp ligand enhanced the molar extinction coefficient (ε) of the metal-to-ligand charge transfer (MLCT) band in the visible region (ε = 7,500 M−1cm−1) compared to that of the phenyl (Ph-)-containing analog (ε = 5,700 M−1cm−1 at λmax = 388 nm). However, introducing 4-R-Ph- groups (R = the electron-withdrawing groups NC-, or NO2-) led to a red shift in the band to λmax = 390, 400, and 401 nm, respectively. Single-crystal X-ray analysis showed the Ph- groups were twisted because of the steric repulsion between the 2,6-protons of the Ph- groups and 5,6-protons of the dmp ligand. The result strongly indicated that the π-conjugation effect of the 4-R-Ph- groups is so weak that the lowering of the energy of the dmp π* orbitals is small. However, when 4-R-ph- was substituted by a 5-membered heterorings, there was a larger red shift, leading to an increase in the ε value of the MLCT absorption band. Thus, the substitution to 2-benzofuranyl- groups resulted in visible-light absorption up to 500 nm and a shoulder peak at around 420 nm (ε = 12,300 M−1cm−1) due to the expansion of π-conjugation over the substituted dmp ligand. The photocatalytic reaction for CO2 reduction was tested using the obtained CuI complexes as photosensitizers in the presence of a Fe(dmp)2(NCS)2 catalyst and 1,3-dimethyl-2-phenyl-2,3-dihydro-1H-benzo[d]imidazole as a sacrificial reductant, which showed improved CO generation.
Introduction
As one of the most important components of artificial photosynthesis, photocatalytic CO2 reduction is attracting much attention. Although metal complexes are key players in CO2 reduction due to their promising photosensitizing and/or catalytic ability, the metals used are often limited to low abundance ones, such as Ru, Re, Os, or Ir (Yamazaki et al., 2015). Thus, substituting these metals with more abundant elements is now being widely focused on in many groups, using the first row transition metals such as Mn, Fe, Co, and Ni to produce a multi-electron catalyst for CO2 reduction (Takeda et al., 2017). However, such the attempts, especially to produce a redox photosensitizer (which functions as a light absorber to transfer an excited electron in its excited state to the catalyst), are limited; the excited state deactivates quickly because of low lying d-d excited state.
Thus, the heteroleptic CuI phenanthroline complexes, such as [CuI(dmp)(P)2]+ (dmp = 2,9-dimethyl-1,10-phenanthroline, P = phosphine ligand), are gaining popularity, because of their long lifetimes, showing strong metal-to-ligand (MLCT) excited-state emission even in a solution at room temperature due to the CuI center's d10 configuration (Ruthkosky et al., 1998). Although homoleptic-type [CuI(dmp)2]+ has the advantage as a photosensitizer in terms of visible-light utilization because it can absorb longer wavelength light (Khnayzer et al., 2013), in the excited state, its oxidation power is less and lifetime shorter than those of a heteroleptic complex. Thus, heteroleptic-type of Cu complexes are now being used as redox photosensitizers to construct many photocatalytic systems (Lazorski and Castellano, 2014). Since these Cu complexes had previously been reported to have excellent emissive properties by McMillin et al. (Breddels et al., 1982), recent developments by Beller et al. on the catalytic H2 evolution reaction using such complexes (Luo et al., 2013; Mejía et al., 2013; Rosas-Hernández et al., 2017) have opened up the way in this area.
However, when using heteroleptic Cu complexes as redox photosensitizers in photocatalytic reactions, some issues remain, such as their stability and light-absorption in the visible region. The first issue, stability, has previously been tackled by our group, where the stability of the CuI complex photosensitizer was improved by connecting phenanthroline phosphine ligands together using –C4H8– alkyl groups (Takeda et al., 2016). Because the tetrahedral coordination around the CuI center was maintained in this molecule, the complex has a long lifetime in the excited state, exhibiting strong emission. Thus, the resulting CuI dimer complexes were much more stable, not only against thermal ligand exchanges in the ground state (Kaeser et al., 2013; Lennox et al., 2016) but also against “exciplex” deactivation of the excited state, even when CH3CN was used as a coordinating solvent (McMillin et al., 1985) and a Cu0 metal particle formed via ligand dissociation in the one-electron reduced state (Eggleston et al., 1997). Thus, utilizing this CuI complex as a redox photosensitizer in the photocatalytic CO2 reduction, we could obtain the best photocatalytic performances for CO2 reduction and clarified the photosensitizing scheme, which forms the corresponding one-electron reduced species through reductive quenching by a reductant such as BIH (1,3-dimethyl-2-phenyl-2,3-dihydro-1H-benzo[d]imidazole) in the excited state, donating the added electrons to CO2 reduction catalyst (Takeda et al., 2018).
So far, many groups have attempted to red shift the 1MLCT absorption band by expanding the dmp ligand's π-conjugation. These attempts are reasonable because the lowest excited state of the CuI complexes can be mainly attributed to the MLCT state, in which a charge on the CuI center transfers to the phenanthroline ligand's low-lying π* orbitals. Tsubomura et al. introduced phenyl groups at the 4,7-positions of dmp, which is known neocuproine, to produce a ligand called bathocuproine (bcp), enhancing the molar extinction coefficient of the resulting CuI complex, thus causing a red shift in the absorption maximum of the MLCT band (Tsubomura et al., 2015). The CuI complexes having a pyrazine- or phenazine-fused dmp ligand have been also reported. However, the resulting Cu complexes showed absorption maxima at around 380 nm (Xu et al., 2015; Heberle et al., 2017). The same group also reported introducing (thiophen-2-yl)vinyl groups at the 2,9-positions of the phenanthroline, but the MLCT absorption band maximum of the Cu complex was still only observed at 388 nm (Chen et al., 2017). Although introducing 4-benzoic acid or thiophene-3-carboxylic acid at the 4,7-positions shifts the absorption band to a longer wavelength, the absorption maxima are limited to 394 and 392 nm, respectively (Chen et al., 2017). Introducing sulfonate groups at the 5,6-positions of bcp also limits the absorption maximum to 390 nm (Rockstroh et al., 2014). Recently, Giereth et al. reported a Cu complex having anthracene-fused dmp, which shows absorption at a longer wavelength at around 430 nm (Giereth et al., 2019). McCullough et al. also reported a unique Cu complex incorporating 2,2′-biquinoline as an α-diimine and applied it as a photocatalytic system for H2 evolution (McCullough et al., 2018). This type of complex showed a surprising red shift in its maximum to 455 nm (Zhang et al., 2007). However, the first-reduction potential of these Cu complexes underwent a positive shift from −2 V for the dmp complex to −1.19 V vs. Fc+/Fc in CH3CN for the anthracene-fused dmp complex and −1.69 V vs. Fc+/Fc in CH2Cl2 for the biquinoline complex, that are more positive than that of the common redox-photosensitizer (dmb = 4,4′-dimethyl-2,2′-bipyridine). Thus, further study of these types of CuI complexes is required so that they can be used as visible-light absorbing redox-photosensitizers.
In this study, CuI complexes are synthesized with new bcp-based phenanthroline ligands, with electron-withdrawing groups on the phenyl groups, or various different aromatic groups such as heterorings, instead of the phenyl groups of the bcp ligand. The aim is improving these systems' visible-light absorption. The CuI complexes' UV-Vis absorption spectra, photophysical properties, and electrochemical properties were examined, providing evidence for a significant red shift in the MLCT absorption band. The redox-photosensitizing ability for photocatalytic CO2 reduction of these CuI complexes was also tested using Fe(dmp)2(NCS)2 as a catalyst in the presence of a reductant.
Results and Discussion
The structures of newly synthesized CuI complexes' structures are shown in Figure 1 alongside the reported CuI complexes Cu(H) (Cuttell et al., 2002) and Cu(ph) (Luo et al., 2013). Two types of substituents were introduced at the 4,7-positions of the dmp ligand, (a) 4-substituted phenyl groups (Figure 1A) and (b) heteroring aryl groups (Figure 1B). In the type (a) substituents, Cu(Bph) is an expanded form of Cu(ph) with an increased number of the phenyl groups, while Cu(NCph) and Cu(NO2ph) have π-electron withdrawing cyano and nitro substituents on the phenyl groups, respectively. In the (b) substituents, the ligands have aryl groups that are smaller in size, i.e., 5-membered heterorings containing O or S atoms at 2- or 3-position, than the phenyl groups adjacent to the dmp ligand. Because S and O atoms have different electronegativities of 3.44 and 2.58, respectively, and van der Waals radii (Bondi, 1964) of 1.52 and 1.80 Å, respectively, the electronic properties of the CuI complexes should be different.
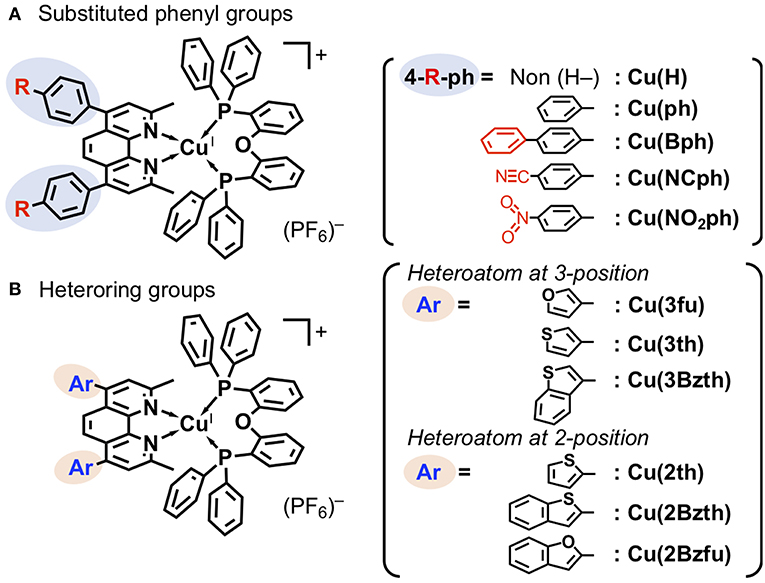
Figure 1. CuI complexes with an aryl-substituted dmp [aryl = substituted phenyl (A) and heteroring (B) groups] and a DPEphos ligands.
The CuI complexes' UV-Vis absorption spectra in a non-coordinating solvent, such as CH2Cl2, are shown in Figure 2, and the data are summarized in Table 1. As a representative system, Cu(ph) exhibited a 1MLCT transition band at 350–500 nm that was moderate in intensity (molar extinction coefficient (ε) ~6000 M−1s−1) and an intraligand π-π* transition band in the shorter-wavelength range with stronger intensity (ε ~50000 M−1s−1), as shown in Figures 2A–C (dotted line). Because the 1MLCT transition is the lowest in energy from the spectrum, the lowest excited state of this complex is strongly indicated to be the MLCT state. Although the π-π* excited state which is higher in energy can proceed thermal relaxation to populate the lowest state, direct excitation of the lowest MLCT transition in the visible region can be assumed to be the most significant in a redox-photosensitizing reaction. Thus, we focused 1MLCT band of the CuI complexes, referring the π-π* band as an indicator of π-system expansion over the aryl-substituted diimine ligands.
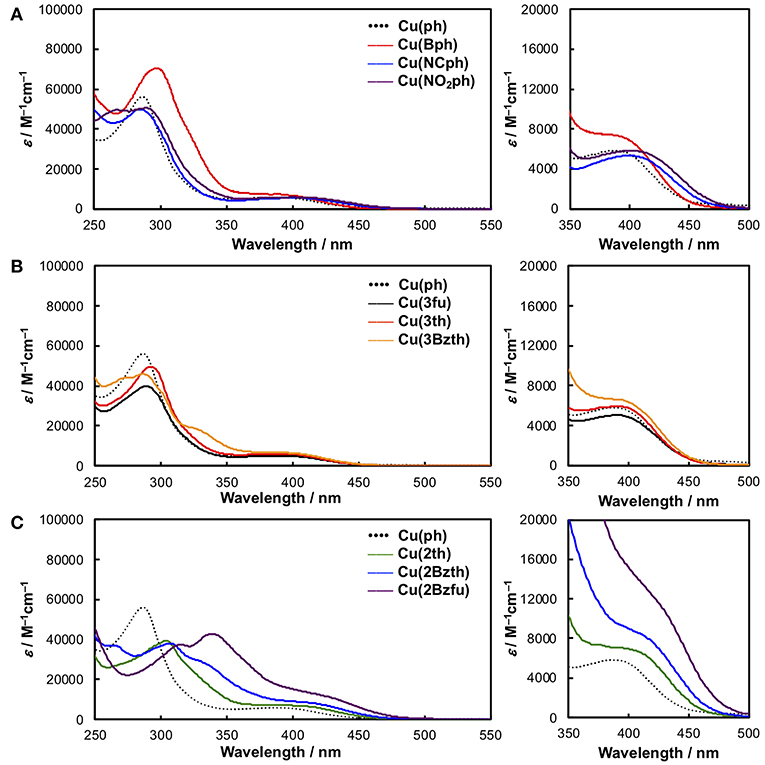
Figure 2. UV-Vis absorption spectra of the CuI complexes in CH2Cl2 alongside the spectrum of Cu(ph) (dotted black line) as a reference. (A) Cu(ph) (dotted black line), Cu(Bph) (red), Cu(NCph) (blue), and Cu(NO2ph) (purple). (B) Cu(3fu) (solid black line), Cu(3th) (red), and Cu(3Bzth) (yellow). (C) Cu(2th) (green), Cu(2Bzth) (blue), and Cu(2Bzfu) (purple). Right panels on each spectrum show magnified section of the spectra, zoomed in on the 1MLCT absorption.
In the case of Cu(Bph) (Figure 2A, red), the intensities of both the 1MLCT and π-π* bands were enhanced to 7500 and ~70000 M−1cm−1, respectively, with no shift observed for the 1MLCT band and a red-shift observed for the π-π* band. Reports indicate that, for the similar types of heteroleptic CuI complexes, adding phenyl groups at the 4,7-positions of the dmp ligand, as in Cu(ph), enhances the 1MLCT absorption band intensity. This enhancement results in a red shifts of the band to λabs = 388 nm (ε = 5700 M−1cm−1) from that of Cu(H) (λabs = 380 nm, ε = 2700 M−1cm−1) due to the enhancement in the corresponding transition dipole moment and stabilization of the π* orbitals in the dmp ligand (Tsubomura et al., 2015). In our case, Cu(Bph) also retained this tendency, where the phenyl groups were modified from those in Cu(ph), but to a much lesser degree.
Introducing NC- groups or NO2- groups at the 4-position of the two phenyl groups, as in Cu(NCph) and Cu(NO2ph) (Figure 2A, blue and purple), respectively, resulted in significant red shift in the 1MLCT absorption bands to 400 and 401 nm, with almost no changes observed in their intensities. Because these substituents are known as π-electron-withdrawing substituents, these red shifts indicate that the π*-orbitals on the dmp ligand were stabilized by these substituents through the π-systems containing the phenyl groups.
Such the red shifts of the 1MLCT bands were also observed for the CuI complexes featuring aryl substituents (Figure 2B). In the case of Cu(3fu) and Cu(3th) (Figure 2B, black and red, respectively), both 1MLCT bands underwent slight red shifts to 390 and 391 nm, respectively, while retaining their intensities, compared to Cu(ph). This result indicates the different electronegativities of the O and S atoms made only negligible effect on the absorption spectra. Cu(3Bzth) also underwent a further red shift of the 1MLCT band than the other complexes in the form of a shoulder at 395 nm (Figure 2B, yellow). In this case, the π-π* absorption band was also red shifted to 330 nm, indicating destabilized π orbitals over the substituted dmp ligand due to the expansion of the π-systems.
Compared to these aryl groups, substituting the 2-positions of the 5-membered rings with heteroatoms resulted in much larger red shifts in both the 1MLCT and π-π* absorption bands. For Cu(2th) (Figure 2C, green), Cu(2Bzth), and Cu(Bz2fu) (Figure 2C, blue and purple, respectively) gradual shifts in the 1MLCT bands of these complexes to longer wavelengths (400, 410, and 420 nm, respectively) occurred alongside an increase in the ε values to 6800, 8200, and 12300 M−1cm−1, respectively. Additionally, the π-π* bands were also red shifted largely up to 350 nm. Thus, the π-conjugation over the substituted dmp ligand was effectively observed, especially for the complex featuring 2-benzofuryl substituents [Cu(Bzfu)]. These trends were almost similar in CH3CN solutions (Figure S1 and Table 2), although undesired generation of the corresponding homoleptic-type CuI complexes with 1MLCT absorption bands at over 450 nm obscured the original heteroleptic complexes.
Then, the single-crystal structures of these CuI complexes were determined to obtain information about the planarity of the substituted dmp ligand, which determines the overlap of the π* orbitals between the aryl substituents and dmp. Here, we could obtain four types of single crystals of CuI complexes, Cu(ph), Cu(NCph), Cu(2Bzth), and Cu(2Bzfu). The structures of these are shown in Figure 3 and full analytical details are in the Supplementary Information (Figures S44–47 and Tables S2–6). All of these complexes have tetrahedral CuI center with an almost right angle between NCuN and PCuP planes (88.6, 89.0, 88.6, and 86.8°, respectively), a configuration required for these types of CuI complexes to exhibit strong emission. There are no remarkable differences in the complexes' residual parts, other than in the substituted dmp ligand, as follows.
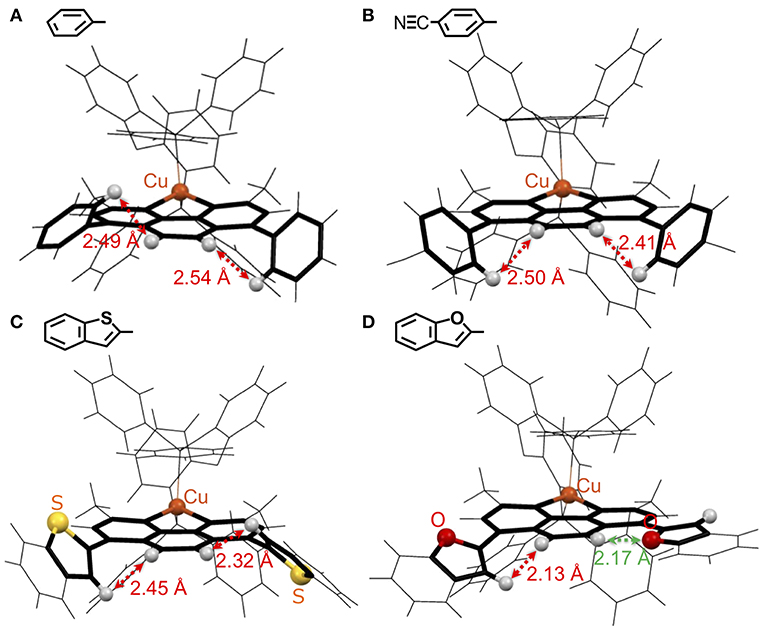
Figure 3. Wire model representations of the single-crystal CuI-complexes: (A) Cu(ph), (B) Cu(NCph), (C) Cu(2Bzth), and (D) Cu(2Bzfu): the phen ligand and adjacent aryl substituents at the 4,7-positions are highlighted. The gray, yellow, red, and orange balls represent H atoms on the phen ligand and on the adjacent positions in the aryl rings, and the S, and O atoms on the aryl substituents, and Cu atoms, respectively.
The twisted angles between the phen and ph planes in the Cu(ph) crystal were found to be 60.2 and 53.9°. Because the H atoms at the 5,6-positions of the phen ligand and the 2,6-positions of the ph groups are in close contact, these ph groups cannot lie in the same plane as that of the phen ligand. Actually, the shortest distances between these H atoms were measured as 2.49 and 2.54 Å. These values are closing to the limitation of van der Waals radius of the H atom, at 1.2 Å (Bondi, 1964). Thus, the steric repulsion between these H atoms hinders the planarity of the ph-phen plane, resulting in a small expansion of the π-conjugation over the substituted dmp ligand. Even when π-electron withdrawing NC- groups were introduced at the 4-position of ph as for Cu(NCph), the twist angles and shortest distances between the H atoms were 49.4 and 53.9°, and 2.50 and 2.41 Å, respectively. Thus, the steric hindrance of the H atoms should still play a role in hindering the NCph-phen bonds' planarity.
Complexes with 5-membered, heteroring substituents exhibited decreased twist angles. In the case of Cu(2Bzth), the angles were 51.1 and 46.3°, maintaining the distances between the H atoms of 2.45 and 2.32 Å. However, the angles in Cu(2Bzfu) drastically decreased to 32.2 and 7.1°, shortening the H–H distance to 2.13 Å and changing the direction of O atom in another 2-benzofuryl substituent to neighbor to the 5-H atom of phen with the shorter distance of 2.17 Å. This means the substituents were able to lie in the same plane as the phen moiety. Because the van der Waals radius of the O atom is 1.52 Å, which is smaller than the 1.80 Å radius of the S atom (Bondi, 1964), the steric hindrance of the planarity over the substituted dmp ligand is not strong enough to result in a planner structure. Thus, the π-systems over the substituted dmp should be the strongest for Cu(2Bzfu) among the CuI complexes in this work. This structural accessibility of a planner structure should lower the π* orbitals over the substituted dmp ligand, resulting in a red shift in the 1MLCT absorption band (Figure 2C, purple).
Figure 4 shows the emission spectra and time-dependences of the emission intensity, and the data are summarized in Table 1. All of the CuI complexes showed strong emission, apart from Cu(NO2ph). In the case of Cu(Bph) (Figures 4A,B, red), the spectrum was almost the same as that of Cu(ph), with a maximum (λem) at 577 nm; however, its quantum yield (Φr) of 0.60 was greater than that of Cu(ph), which was 0.52. This is reasonable when considering the increased oscillator strength for the MLCT transition, as observed in the increased ε value of the 1MLCT absorption band in the UV-Vis spectrum. Thus, the radiative transition rate (kr) of the excited state should be enhanced, increasing the Φr because this value is defined in the context of kr in all of the deactivation paths of the excited state (kr + knr in this case). The increased, excited-state lifetime [τem = 1/(kr + knr)] for Cu(Bph) of 22.5 μs from that of Cu(ph) (19.4 μs) indicates that the knr value of Cu(Bph) is lower than that of Cu(ph). This should be due to the increased mixing of the π-π* excited state in the lowest MLCT state, which is indicated by a red shift in the intraligand 1π-π* absorption band close to the 1MLCT band. Because these CuI complexes exhibit delayed fluorescence, the actual deactivation rates of kr and knr could not be obtained in these measurements.
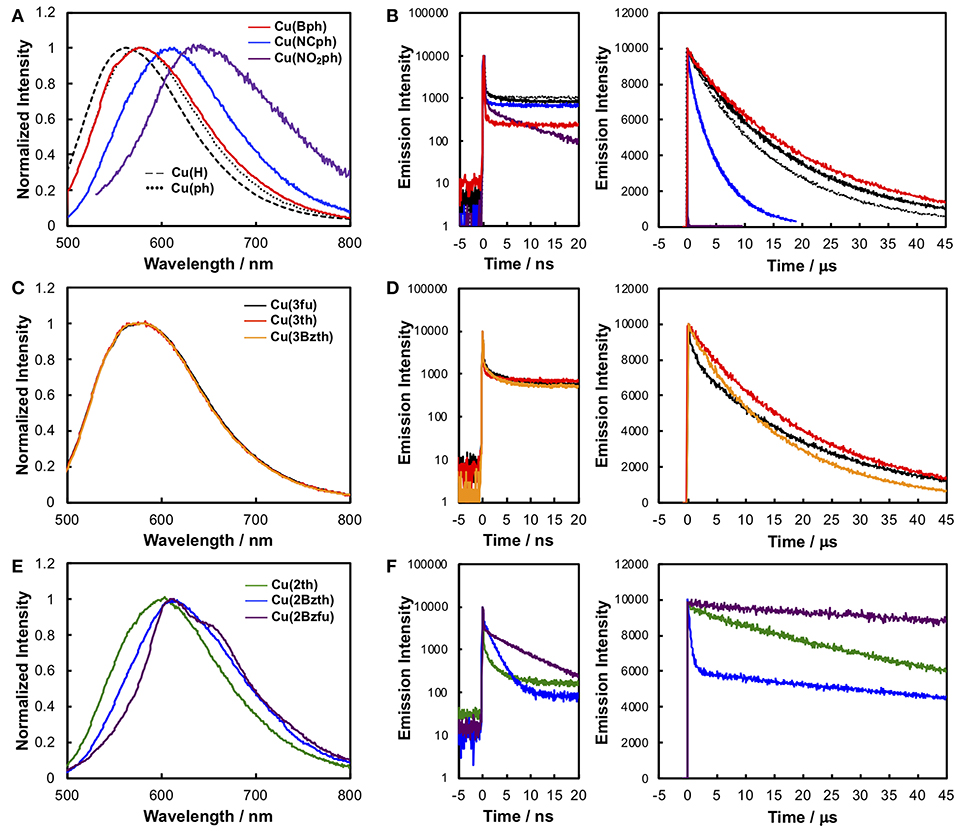
Figure 4. The corrected emission spectra (A, C, and E) and time-dependence data of the emission intensity (B, D, and F) of the CuI complexes in CH2Cl2 at RT. (A) Emission spectra and (B) time-dependence data of the intensity of Cu(H) (broken black line), Cu(ph) (dotted black line), Cu(Bph) (red), Cu(NCph) (blue), and Cu(NO2ph) (purple). (C) Emission spectra and (D) time-dependence data of the intensity of Cu(3fu) (black line), Cu(3th) (red), and Cu(3Bzth) (yellow). (E) Emission spectra and (F) time-dependence data of the intensity of Cu(2th) (green), Cu(2Bzth) (blue), and Cu(2Bzfu) (purple). Left panels on (B, D, and F) were observed from a shorter range of each right panel. The samples were degassed using the freeze–pump–thaw method. Table S1 summarizes the excitation and monitored wavelengths.
In the cases of Cu(NCph) and Cu(NO2ph) (Figures 4A,B, blue and purple, respectively), the emission maxima were red shifted to 609 and 640 nm, respectively, manifesting as red shifts in the corresponding 1MLCT absorption bands. In both cases, the Φr and τem values were significantly decreased [Φr = 0.16 and τem = 5.6 μs for Cu(NCph)], and Cu(NO2ph) showed almost no emission (Φr < 0.00, τem = 0.01 μs). These can be reasonably explained by the energy-gap law similarly to the substituent effects on the emission behaviors of α-diimine complexes of Ru and Re (Cook et al., 1984; Worl et al., 1991). The lowered energy of the MLCT transition resulted in a lower energy gap between the excited state and the ground state, thus leading to an increased knr value. Cu(NO2ph) exhibited almost no emissions, which can be attributed to charge localization on the strong, electron-withdrawing NO2 groups in the excited state, leading to a charge-separated radical-like state (Prei et al., 2015). This, in turn, should lead to an increase in the knr value.
The CuI complexes, substituted with smaller aryl groups, showed two different sets of behavior. The emission spectra of the Cu(3fu), Cu(3th), and Cu(3Bzth) complexes containing 5-membered ring systems with heteroatoms at 3-position (Figures 4C,D, black, red, and yellow, respectively), showed almost the same features as those found in the Cu(ph) spectrum. All of these complexes showed strong emissions with Φr values of 0.24–0.45 and τem values of ca. 20 μs, values that are almost the same as those of Cu(ph). In these cases, changing the heteroatoms from O to S in the aryl rings appeared to have no great effect on the complexes' transition energies, but Φr value increased from 0.24 for Cu(3fu) to 0.35 for Cu(3th), which further increased in Cu(3Bzth) (Φr = 0.45).
However, in systems where the heteroatoms featured at the 2-position of the rings, as in Cu(2th), Cu(2Bzth), and Cu(2Bzfu) (Figures 4E,F, green, blue, and purple for Cu(2th), Cu(2Bzth), and Cu(2Bzfu), respectively), the emissions were drastically different from those of the other complexes. The emission spectra were observed to gradually red shift in the complexes in the aforementioned order and their shapes changed to vibronic shapes, particularly for Cu(2Bzfu). Because the excitation spectrum from which this emission was monitored was in accordance with the UV-Vis absorption spectrum of Cu(2Bzfu) (Figure S3A), this emission could be confirmed to arise from the excited state of Cu(2Bzfu).
This emission of Cu(2Bzfu) was found to be strongly air sensitive. If the emission spectrum was recorded for a sample prepared under an Ar atmosphere instead of a vacuum-degassed sample, the spectrum was observed to drastically change, resulting in a mismatch between the excitation and absorption spectra of Cu(2Bzfu) in CH2Cl2 (Figure S3B). However, this was not the case for the data recorded in a CH3CN solution, which showed no remarkable changes in the spectral features, even for a sample prepared under an Ar atmosphere (Figure S12C) compared to under vacuum conditions (Figure S3C).
Interestingly, these CuI complexes' τem values increased to over 100 μs, with a corresponding decrease in the Φr values. These results strongly indicate that the emissive lowest excited state changed from the MLCT state to another state, possibly the intraligand π-π* excited state. This is a reasonable assumption, considering the red shift in the π-π* transition band, as observed in the UV-Vis absorption spectra, from the π-expansion of the dmp ligand. Thus, in these complexes, the emission from the lowest excited state should arise from the delayed fluorescence of the intraligand 3π-π* state.
The spectral feature depended strongly on the heteroatoms on the 2-positions of the aryl substituents. In the case of Cu(2th) and Cu(2Bzth), the emission spectra were much broadened than that of Cu(2Bzfu), although the lifetimes were still long. These results strongly indicate that the accessibility of the planar structure over the aryl substituted dmp enhances the π-conjugation over the dmp ligand. The similar phenomena about the long excited-state lifetime caused by the planarity between aryl substituents and α-diimine ligand has been discussed for emissive Ru complexes, in which the delocalization of negative charge over the substituted bpy (bpy = 2,2′-bipyridine) in the 3MLCT excited state lowers their structural changes from the ground state, leading to decreasing knr (Damrauer et al., 1997; Majewski et al., 2016). It is also reported that the CuI(dmp) complex has the close-lying 3π-π* state near the lowest 3MLCT excited state as for CuI(dmp)(PPh3), which showed the vibronic emission spectrum at 77 K as a long-lifetime component as τem ~ 10 ms (Rader et al., 1981). Therefore, the long lifetime emission of Cu(2th), Cu(2Bzth), and Cu(2Bzfu) can be reasonably attributed to the participation of 3π-π* excited state.
All of these tendencies were also valid even in CH3CN as a photocatalytic reaction solvent, except that the emission was significantly quenched compared to that observed in CH2Cl2 solutions due to “exciplex” formation, in which a solvent molecule coordinates to the Cu center as a fifth ligand in the excited-state CuI complexes (Figure S2 and Table 2). This type of quenching is characteristic of tetrahedral CuI complexes as the d10 CuI center decreases its charges upon closing to a d9 configuration in the MLCT excited state, changing the structure to planer one because of the Jahn-Teller effect (McMillin et al., 1985).
Thus, the quenching all of the CuI complexes studied in CH3CN indicates that they have MLCT character in their excited state to some extent, even for Cu(2th), Cu(2Bzth), and Cu(2Bzfu), in which the excited state was mainly the π-π* state. The extents of the MLCT character should increase especially in CH3CN because this state has CT in character, that are stabilized in a polarized solvent such as CH3CN more strongly than the intraligand π-π* excited state. Actually, such the stabilizations were seen as red-shift of emission maxima in CH3CN compared to those in CH2Cl2. In the case of Cu(NO2ph), the emission behavior completely changed in CH3CN, possibly as a result of photodecomposition, manifesting in a different excitation spectrum than Cu(NO2ph)'s original absorption spectrum.
Photocatalytic CO2 reduction reactions were performed using these newly designed CuI complexes as redox photosensitizers mixed with FeII(dmp)2(NCS)2 (0.05 mM) as a catalyst and BIH (10 mM) as a reductant under a 436 nm monochromic light in a mixed solution of CH3CN–triethanolamine [TEOA, 5:1 (v/v)]. The Cu photosensitizer's concentration was fixed at 0.5 mM, showing almost no transparency at 436 nm in reaction cells with a 1-cm optical path length. Figure 5 shows the photocatalytic reaction results. The products were found to be only CO and H2, without the formation of any HCOOH as reported in a previous study performed under similar conditions (Takeda et al., 2016), and these compounds were linearly generated against the photoirradiation time for up to 2 h.
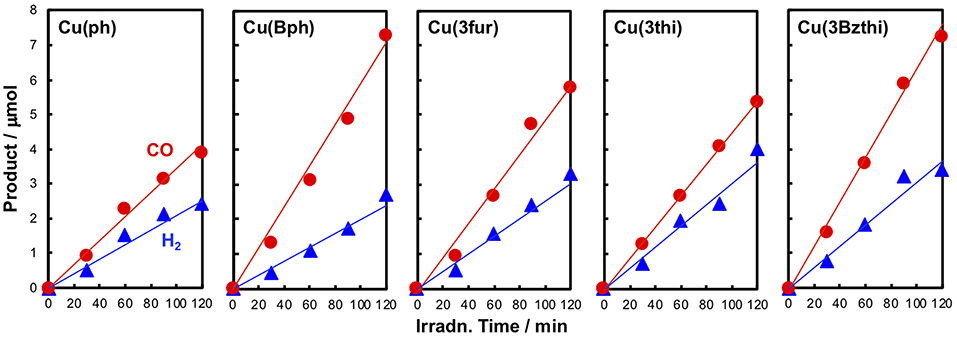
Figure 5. Time-course data of CO (red) and H2 (blue) evolution during the photocatalytic reactions using the CuI complexes as redox photosensitizers. A 4 ml CH3CN-TEOA (5:1 v/v) solution containing a CuI complex (0.5 mM), Fe(dmp)2(NCS)2 (0.05 mM), and BIH (10 mM) was irradiated using the 436 nm monochromatic light (intensity: 3 × 10−8 einstein s−1) of a Xe lamp under a CO2 atmosphere at 25°C.
The photocatalytic properties were highly dependent on the Cu complex photosensitizer used (Table 3). When Cu(Bph) was used, the efficiencies were enhanced to Φr = 3.9 with H2 formation (Φr = 1.4). Take into account the low results for Cu(H) [Φr = 1.1 (Takeda et al., 2016)] and Cu(ph) (Φr = 2.3), increasing the number of the ph groups at the 4,7-positions of dmp tended to enhance the photosensitizing ability of these complexes for CO2 reduction.
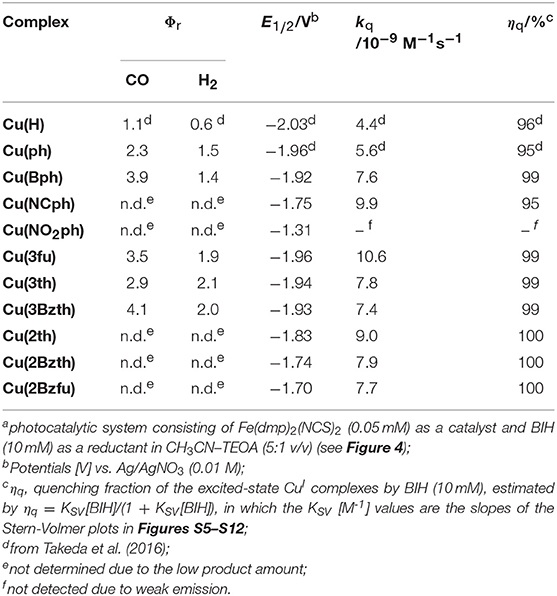
Table 3. Quantum Yields of the Photocatalytic CO2 Reduction (Φr) Using the CuI Complexes as Redox-Photosensitizer,a Reduction Potentials (E1/2), and Excited-State Quenching Rate (kq) by BIH of the CuI Complexes.
Such the enhancement in the photosensitizing ability was also confirmed in the cases of complexes featuring 5-membered ring systems with heteroatoms at the 3-position, such as Cu(3fu), Cu(3th), and Cu(3Bzth). The most efficient case was Cu(3Bzth), which showed Φr values of 4.1 and 2.0 for CO and H2 evolution, respectively. Even in the cases of Cu(3fu) and Cu(3th), the efficiencies for the CO2 reduction were improved to Φr (CO) = 3.5 and 2.9, respectively, over those of Cu(ph).
However, the CuI complexes containing 5-membered ring systems with heteroatoms at 2-position, such as Cu(2th), Cu(2Bzth), and Cu(2Bzfu), showed no any photosensitizing ability in this reaction system. These complexes may have lower oxidative power in their excited state because their highest occupied molecular orbital (HOMO) energies should now increase since the π orbitals in the substituted dmp, as well as the d orbitals on the CuI center, are destabilized. Actually, the first oxidation wave in the cyclic voltammetry (CV) measurements, an indicator of the HOMO energy, showed positive shifts for Cu(2th), Cu(2Bzth), and Cu(2Bzfu) (shoulder waves around 0.9 V vs. Ag/AgNO3) compared to that of Cu(ph) (Ep = 0.92 V vs. Ag/AgNO3) (Figure S4).
The excited states of these CuI complexes were effectively quenched in the presence of BIH, except for that of Cu(NO2ph), which means that all of these complexes might generate the corresponding one-electron reduced state through the reductive quenching of their excited states by BIH during photoirradiation (Tamaki et al., 2013; Takeda et al., 2018). The quenching rate constants (kq) were estimated to be ~1010 M−1s−1, corresponding to quantitative quenching (ηq) under photocatalytic conditions with 10 mM of BIH (Table 3). Because the quenching behavior in terms of the emission lifetimes was in good agreement with the emission intensity changes, and no changes were observed in the UV-vis and emission spectra of the CuI complexes, even in the presence of BIH (Figures S5–S12). These quenching reactions can be attributed to a dynamic quenching process, in which the excited state reacts with BIH bimolecularly.
Then, in situ UV-Vis absorption spectral changes in the reaction solutions during the photocatalytic reactions were investigated and compared to those of the complexes recorded under an Ar atmosphere for up to 1 h of photoirradiation. In the cases of Cu(3fu), Cu(3th), and Cu(3Bzth) (Figures S15–17), which were good photosensitizers for photocatalytic CO2 reduction, almost no changes occurred in their spectra. This indicates that there was almost no decomposition of the Cu complexes in the photosensitizing cycles, donating electrons to the Fe catalyst, for the oxidation of BIH and CO2 reduction. Under an Ar atmosphere, the MLCT absorption bands of the original CuI complexes decreased just after the photoirradiation caused broad absorption up to 950 nm. These changes clearly indicate the original CuI complexes' photodecomposition. Because the one-electron reduced state produced by the reductive quenching of the excited state cannot donate to the CO2 reduction cycle of the Fe catalyst, the reduced species of the CuI complexes accumulate in the reaction solutions, decomposing due to their anionic radical character if CO2 is not present.
On the other hand, non-photosensitizing CuI complexes, such as Cu(NCph), Cu(2th), Cu(2Bzth), and Cu(2Bzfu) (Figures S13, S18–20, respectively) showed signs of drastic decomposition even in the presence of CO2. This means that the Cu complexes cannot donate their electrons generated through the reductive quenching by BIH in the excited state for the CO2 reduction. Thus, these complexes showed no photocatalytic CO2 reduction properties. However, the lack of the photosensitizing ability of Cu(NO2ph) should be due to its unstable excited state because no changes were observed, neither under a CO2 nor an Ar atmosphere, meaning that no any redox cycles occurred under photoirradiation.
The CuI complexes' electrochemical properties were then examined, and the results are shown in Figure 6. All of the CuI complexes, apart from Cu(H), showed a reversible redox couple in the CV as the first reduction wave, indicating that the corresponding one-electron reduced species was stabilized via the introduction of the aryl substituents on the dmp ligand, as observed for Cu(ph). This is quite reasonable considering that the first reduction occurs in the dmp ligand's low-lying π* orbital. However, for the CV of Cu(NO2ph), the redox couple current was much higher without any sharpening. Thus, the first reduction wave in the CV of Cu(NO2ph) arises as a result of two sequential local electron reductions in the two NO2ph parts of one Cu(NO2ph). The redox potentials (E1/2) of these first reduction waves are summarized in Table 3. Apparently, introducing electron-withdrawing groups, such as NCph and NO2ph, resulted in positive shifts in the E1/2 values up to −1.75 and −1.31 V vs. Ag/AgNO3 for Cu(NCph) and Cu(NO2ph), respectively, indicating that the electron-donating ability of the corresponding reduced species was lowered. Those of the Cu(Bph), Cu(3fu), Cu(3th), and Cu(3Bzth) complexes that have photosensitizing ability in this photocatalytic system maintained values more negative than −1.9 V, although these E1/2 values were positively shifted from those of Cu(H) and Cu(ph) (E1/2 = −2.03 and −1.96 V vs. Ag/AgNO3, respectively). For Cu(2th), Cu(2Bzth), and Cu(2Bzfu), in which the π-system largely extends to the aryl substituents, exhibited more positive first reduction potentials of −1.83, −1.74, and −1.70 V, respectively, due to the stabilization of the π* orbital over the substituted dmp ligand. Thus, the lowering energy of the π* orbital on the dmp ligand via the introduction of phenyl rings with π-electron-withdrawing groups or 5-membered aryl groups was clearly confirmed.
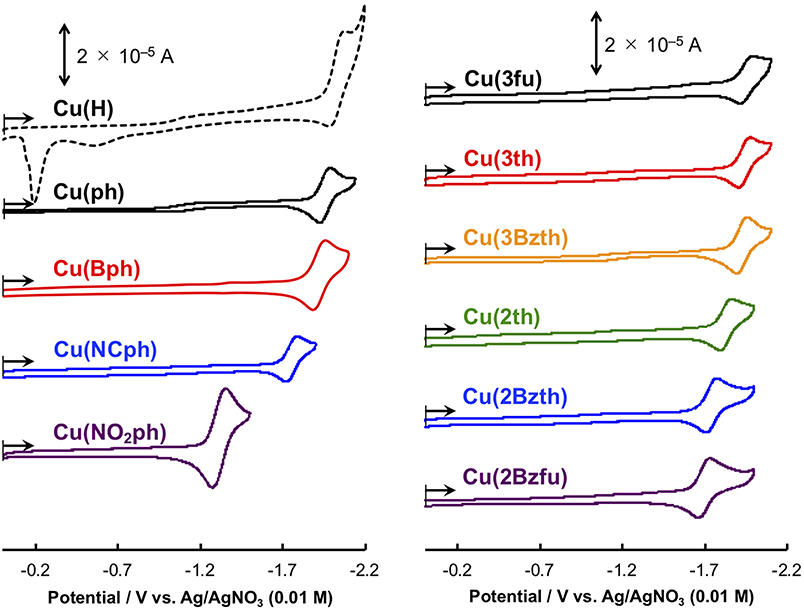
Figure 6. Cyclic voltammograms of the CuI complexes (0.5 mM) in CH3CN containing 0.1 M Et4NBF4 as a supporting electrolyte at a scan rate of 0.1 V s−1. WE: glassy carbon (Φ 3 mm); CE: Pt wire; RE: Ag/AgNO3 (0.01 M). The Cu(H) and Cu(ph) voltammograms are reproduced from ref. (Takeda et al., 2016) for comparison.
Compared to the first reduction wave potential of Fe(dmp)2(NCS)2 in CH3CN–TEOA (5:1 v/v) solution (−1.61 V vs. Ag/AgNO3) under a CO2 atmosphere (Takeda et al., 2016), the reducing power of these Cu complexes is enough for them to donate their first single electron on the corresponding reduced species to the Fe catalyst. However, the decomposition behavior during the photoirradiation process indicates that the electron transfer to the Fe catalyst is inefficient, resulting in an accumulation of the reduced species of the Cu complexes and decomposition over longer time scales than those of the CV measurements. We are now continuing the photocatalytic reaction using the other catalysts for the CO2 reduction that function at a more positive potential.
Conclusion
This study found the heteroleptic CuI complexes' visible-light absorption properties could be improved via expanding the dmp ligand's π-systems. Simple expansion using biphenyl substitution at the 4,7-popsitions of dmp enhanced ε without red shift of the 1MLCT band compared to that of the ph substituted one. Although π-electron accepting substituents such as 4-NCph or 4-NO2ph shifted the band, the λmax values were still around 400 nm. The X-ray single crystal analysis showed the presence of protons at the 2,6-positions of the ph substituents slows the π-conjugation between the ph groups and dmp due to the steric hindrance of the 5,6-protons in the dmp ligand. Thus, substitution with 5-membered heterorings, with heteroatoms and no protons at the 2-positions, was enough to make improvements to the properties of the complexes. Thus, the complexes containing 2-benzofuryl groups having an O atom which size is smaller than a S atom at each 2-position showed a planner structure over the substituted dmp ligand, resulting in a red shift in the 1MLCT absorption bands up to 500 nm. Because the 3-thienyl and 3-furyl substitutions showed almost no effect on the light absorbing property, this red shift in Cu(2Bzfu) was not from a difference of electronegativities of the S and O atoms. Photocatalytic CO2 reduction reactions were carried out with the complexes in the presence of a Fe catalyst, showing quantum yields of up to 4% for the maximum amount of CO generated. The knowledge obtained in this study should help not only for further expansion of visible-light absorbing but also for designing the efficient redox-photosensitizer using the CuI complex to construct efficient earth-abundant system for the photocatalytic CO2 reduction.
Experimental
General Measurements
1H NMR spectra were recorded on a JEOL ECA-400II NMR spectrometer. The chemical shifts and coupling values arising from the second order effects of the protons in the phenanthroline derivatives were assigned by analyzing the obtained 1H NMR spectra using the iNMR (ver. 6.1.8) and WINDNMR (ver. 7.1.14) software (Reich, 1995). UV-vis absorption spectra were recorded on a JASCO V-565 or V-670 spectrometers. To measure the molar extinction coefficiencies (ε [M−1cm−1]), a 10 mL solution containing quantitatively weighted each CuI complexes (~2 mg) was prepared by dissolving in CH2Cl2 or CH3CN as a stock solution. An 1 mL of the stock solution was further diluted to 5 mL by each solvent and UV-vis absorption spectra of these two solutions were recorded using a cuvette cell having an 1-cm path length. After confirmation of no concentration effect on the spectral features indicating no dimer formation by comparison these two spectra, the absorbance was divided by each concentration to obtain ε value. The spectrum of the stock solution was used for assessment of the 1MLCT band and the latter was used for π-π* absorption band because the detection range of absorbance (0.1−1.0) is suitable. Emission and excitation spectra were recorded on a JASCO FP8600 fluorescence spectrometer. Emission quantum yields of the CuI complexes in CH3CN were determined by a relative method, using an air-saturated CH3CN solution containing Ru(bpy)3(PF6) as a standard (Φ = 0.018) (Suzuki et al., 2009; Ishida and Beeby, 2016). Emission quantum yields in CH2Cl2 were collected using a Hamamatsu Photonics C9920–02G absolute photoluminescence quantum yield measurement system, consisting of a calibrated integrating sphere and a multi-channel spectrometer. Excited-state lifetimes were recorded on a HORIBA FluoroCube 1000U-S single-photon counting system. The sample solutions were degassed, via freeze–pump–thaw cycles, before the emission and lifetime measurements. For the quenching experiments, changes in the emission intensities and excited-state lifetimes of the CuI complexes in CH3CN, with or without various concentrations of BIH, were monitored under an Ar atmosphere. CVs were measured using Et4NBF4 (0.1 M) as a supporting electrolyte under an Ar atmosphere using a BAS CHI620EX or CHI760Es electrochemical analyzer with a glassy carbon working electrode (diameter, 3 mm), an Ag/AgNO3 (0.01 M) as a reference electrode, and a Pt counter electrode.
Photocatalytic Reactions
A 4-mL CH3CN–TEOA (5:1 v/v) solution containing Fe(dmp)2(NCS)2 (0.05 mM), and the Cu photosensitizer (0.5 mM), and BIH (10 mM) as a reductant in a quartz cubic cell (1 cm path length; 11.0 mL volume) was bubbled with CO2 for 20 min. For the quantum yield determinations, a SHIMADZU QYM-01 photoreaction, quantum yield, evaluation system was adopted. The sample solution was irradiated using an Asahi Spectra Co. MAX-303 300-W Xe lamp with a 436 nm band-pass filter (FWHM: 10 nm), with the irradiated light intensity set at 3 × 10−8 einstein s−1 and the temperature of the reaction solution was maintained at 25 ± 0.1 °C using an IWAKI CTS-134A cooling thermo pump during the irradiation. The gaseous products, i.e., CO and H2, were analyzed using GC-TCD (GL science GC323) with an active carbon column. The amount of formic acid in the reaction solution with diluted water was analyzed using a capillary electrophoresis system (Otsuka Electronics Co. 7100L) with a buffer solution (pH 5.9) consisting of quinolinic acid, hexadecyltrimethylammonium hydroxide, and 2-amino-2-hydroxymethyl-1,3-propanediol as the electrolyte. To observe in situ UV-Vis spectral changes of the photocatalytic-reaction solution, the solution was irradiated, using an Ushio Optical Module high-pressure Hg lamp (BA-H500), with a 436 nm band-pass filter (FWHM = 10 nm), purchased from Asahi Spectra Co., and a CuSO4 solution (250 g L−1, 5 cm path length) filter with a 436 nm band-pass filter (FWHM: 10 nm) purchased from Asahi Spectra Co. Neutral density glass filters reduced the light intensity. The spectral changes were recorded on a Photal MCPD-6800 photodiode array detector equipped with a MC-2530 light source.
Data Availability
All datasets generated for this study are included in the manuscript and/or the Supplementary Files.
Author Contributions
HT and OI planned the all experiments. HT and YM synthesized the samples and performed the photophysical, electrochemical, and photocatalytic measurements. HT, HS, and HU analyzed the single crystal structures. HT wrote the manuscript and all authors have read and approved it.
Funding
This work was supported by JST CREST (Grant Number JPMJCR13L1). HT has received funding from JSPS KAKENHI (Grant Number JP16K17876). HU has received funding from JSPS KAKENHI (Grant Number JP17K05745 and JP18H04504).
Conflict of Interest Statement
The authors declare that the research was conducted in the absence of any commercial or financial relationships that could be construed as a potential conflict of interest.
Supplementary Material
The Supplementary Material for this article can be found online at: https://www.frontiersin.org/articles/10.3389/fchem.2019.00418/full#supplementary-material
Data Sheet 1. The CuI complexes' photophysical properties in CH3CN, emission and excitation spectra of Cu(2Bzfu) in CH2Cl2 under Ar or degassed atmospheres, positive scans of the cyclic voltammograms of the CuI complexes, quenching experiments of the excited state of the CuI complexes by BIH, in situ UV-Vis spectral changes during the photoirradiation reactions, experimental details such as materials, synthesis, 1H NMR spectra, peak analysis of the 1H NMR spectra of the newly synthesized ligands, and details of the single-crystal X-ray crystallography of Cu(ph), Cu(NCph), Cu(2Bzth), and Cu(2Bzfu).
Data Sheet 2. Crystallographic data for Cu(ph), Cu(NCph), Cu(2Bzth), and Cu(2Bzfu) (CCDC numbers: 1895526-1895529) embedding RES and structure factors.
References
Bondi, A. (1964). van der Waals Volumes and Radii. J. Phys. Chem. 68, 441–451. doi: 10.1021/j100785a001
Breddels, P. A., Berdowski, P. A. M., Blasse, G., and McMillin, D. R. (1982). Luminescence of Some CuI Complexes. J. Chem. Soc. Faraday Trans. 278, 595–601. doi: 10.1039/f29827800595
Chen, N.-Y., Xia, L., Lennox, A. J. J., Sun, Y., Chen, H., Jin, H.-M., et al. (2017). Structure-Activated copper photosensitisers for photocatalytic water reduction. Chem. A Eur. J. 23, 3631–3636. doi: 10.1002/chem.201602598
Cook, M. J., Lewis, A. P., McAuliffe, G. S. G., Skarda, V., Thomson, A. J., Glasper, J. L., et al. (1984). Luminescent metal complexes. part 2. a model for the luminescence properties of the tris-chelates of substituted 2,2'-Bipyridyls with Ruthenium(II). J. Chem. Soc. Perkin Trans. 2, 1303–1307. doi: 10.1039/P29840001303
Cuttell, D. G., Kuang, S.-M., Fanwick, P. E., McMillin, D. R., and Walton, R. A. (2002). Simple Cu(I) complexes with unprecedented excited-state lifetimes. J. Am. Chem. Soc. 124, 6–7. doi: 10.1021/ja012247h
Damrauer, N. H., Boussie, T. R., Devenney, M., Mccusker, J. K., and April, R. V. (1997). Effects of intraligand electron delocalization, steric tuning, and excited-state vibronic coupling on the Photophysics of Aryl-Substituted Bipyridyl Complexes of Ru(II). J. Am. Chem. Soc. 119, 8253–8268. doi: 10.1021/ja971321m
Eggleston, M. K., McMillin, D. R., Koenig, K. S., and Pallenberg, A. J. (1997). Steric effects in the ground and excited states of systems. Inorg. Chem. 36, 172–176. doi: 10.1021/ic960698a
Giereth, R., Reim, I., Frey, W., Junge, H., Tschierlei, S., and Karnahl, M. (2019). Remarkably long-lived excited states of copper photosensitizers containing an extended π-system based on an anthracene moiety. Sustain. Energ. Fuels 3, 692–700. doi: 10.1039/C8SE00521D
Heberle, M., Tschierlei, S., Rockstroh, N., Ringenberg, M., Frey, W., Junge, H., et al. (2017). Heteroleptic copper photosensitizers: why an extended π-system does not automatically lead to enhanced hydrogen production. Chem. A Eur. J. 23, 312–319. doi: 10.1002/chem.201604005
Ishida, H., and Beeby, A. (2016). Guidelines for measurement of luminescence spectra and quantum yields of inorganic and organometallic compounds in solution and solid State (IUPAC Technical Report). Pure Appl. Chem. 88, 701–711. doi: 10.1515/pac-2014-0706
Kaeser, A., Mohankumar, M., Mohanraj, J., Monti, F., Holler, M., Cid, J.-J., et al. (2013). Heteroleptic Copper(I) Complexes prepared from phenanthroline and bis-phosphine ligands. Inorg. Chem. 52, 12140–12151. doi: 10.1021/ic4020042
Khnayzer, R. S., McCusker, C. E., Olaiya, B. S., and Castellano, F. N. (2013). Robust Cuprous Phenanthroline Sensitizer for Solar Hydrogen Photocatalysis. J. Am. Chem. Soc. 135, 14068–14070. doi: 10.1021/ja407816f
Lazorski, M. S., and Castellano, F. N. (2014). Advances in the Light Conversion Properties of Cu(I)-Based Photosensitizers. Polyhedron 82, 57–70. doi: 10.1016/j.poly.2014.04.060
Lennox, A. J. J., Fischer, S., Jurrat, M., Luo, S.-P. P., Rockstroh, N., Junge, H., et al. (2016). Copper-Based photosensitisers in water reduction: a more efficient in situ formed system and improved mechanistic understanding. Chem. A Eur. J. 22, 1233–1238. doi: 10.1002/chem.201503812
Luo, S. P., Esteban, M., Friedrich, A., Pazidis, A., Junge, H., Surkus, A. E., et al. (2013). Photocatalytic water reduction with copper-based photosensitizers: a noble-metal-free system. Angew. Chem. Int. Ed. 52, 419–423. doi: 10.1002/anie.201205915
Majewski, M. B., Smith, J. G., Wolf, M. O., and Patrick, B. O. (2016). Long-lived, emissive excited states in direct and amide-linked thienyl-Substituted RuII Complexes. Eur. J. Inorg. Chem. 1470–1479. doi: 10.1002/ejic.201501436
McCullough, B. J., Neyhouse, B. J., Schrage, B. R., Reed, D. T., Osinski, A. J., Ziegler, C. J., et al. (2018). Visible-light-driven photosystems using heteroleptic Cu(I) photosensitizers and Rh(III) catalysts to produce H2. Inorg. Chem. 57, 2865–2875. doi: 10.1021/acs.inorgchem.7b03273
McMillin, D. R., Kirchhoff, J. R., and Goodwin, K. V. (1985). Exciplex Quenching of photo-excited copper complexes. Coord. Chem. Rev. 64, 83–92. doi: 10.1016/0010-8545(85)80043-6
Mejía, E., Luo, S.-P., Karnahl, M., Friedrich, A., Tschierlei, S., Surkus, A., et al. (2013). A noble-metal-free system for photocatalytic hydrogen production from water. Chem. A Eur. J. 19, 15972–15978. doi: 10.1002/chem.201302091
Preiß, J., Jäger, M., Rau, S., Dietzek, B., Popp, J., Martínez, T., et al. (2015). how does peripheral functionalization of ruthenium(II)-Terpyridine complexes affect spatial charge redistribution after photoexcitation at the franck-condon point? ChemPhysChem 16, 1395–1404. doi: 10.1002/cphc.201500223
Rader, R. A., McMillin, D. R., Buckner, M. T., Matthews, T. G., Casadonte, D. J., Lengel, R. K., et al. (1981). Photostudies of 2,2'-bipyridine bis(triphenylphosphine)copper(1+), 1,10-phenanthroline bis(triphenylphosphine)copper(1+), and 2,9-dimethyl-1,10-phenanthroline bis(triphenylphosphine)copper(1+) in solution and in rigid, low-temperature glasses. simultaneous multiple emissions from intraligand and charge-transfer states. J. Am. Chem. Soc. 103, 5906–5912. doi: 10.1021/ja00409a048
Reich, H. J. (1995). WinDNMR: Dynamic NMR spectra for windows. J. Chem. Edu. 72:1086. doi: 10.1021/ed072p1086.1
Rockstroh, N., Lochbrunner, S., Tschierlei, S., Junge, H., Karnahl, M., Beller, M., et al. (2014). Substitution-controlled excited state processes in heteroleptic copper(I) photosensitizers used in hydrogen evolving systems. ChemPhysChem 15, 3709–3713. doi: 10.1002/cphc.201402585
Rosas-Hernández, A., Steinlechner, C., Junge, H., and Beller, M. (2017). Earth-abundant photocatalytic systems for the visible-light-driven reduction of CO2 to CO. Green Chem. 19, 2356–2360. doi: 10.1039/C6GC03527B
Ruthkosky, M., Kelly, C. A., Castellano, F. N., and Meyer, G. J. (1998). Electron and energy transfer from CuI MLCT excited states. Coord. Chem. Rev. 171, 309–322. doi: 10.1016/S0010-8545(98)90045-5
Suzuki, K., Kobayashi, A., Kaneko, S., Takehira, K., Yoshihara, T., Ishida, H., et al. (2009). Reevaluation of absolute luminescence quantum yields of standard solutions using a spectrometer with an integrating sphere and a back-thinned CCD Detector. Phys. Chem. Chem. Phys. 11, 9850–9860. doi: 10.1039/B912178A
Takeda, H., Cometto, C., Ishitani, O., and Robert, M. (2017). Electrons, photons, protons and earth-abundant metal complexes for molecular catalysis of CO2 reduction. ACS Catal. 7, 70–88. doi: 10.1021/acscatal.6b02181
Takeda, H., Kamiyama, H., Okamoto, K., Irimajiri, M., Mizutani, T., Koike, K., et al. (2018). Highly efficient and robust photocatalytic systems for CO2 reduction consisting of a Cu(I) photosensitizer and Mn(I) catalysts. J. Am. Chem. Soc. 140, 17241–17254. doi: 10.1021/jacs.8b10619
Takeda, H., Ohashi, K., Sekine, A., and Ishitani, O. (2016). Photocatalytic CO2 reduction using Cu(I) photosensitizers with a Fe(II) catalyst. J. Am. Chem. Soc. 138, 4354–4357. doi: 10.1021/jacs.6b01970
Tamaki, Y., Koike, K., Morimoto, T., and Ishitani, O. (2013). Substantial Improvement in the efficiency and durability of a photocatalyst for carbon dioxide reduction using a benzoimidazole derivative as an electron donor. J. Catal. 304, 22–28. doi: 10.1016/j.jcat.2013.04.002
Tsubomura, T., Kimura, K., Nishikawa, M., and Tsukuda, T. (2015). Structures and Photophysical Properties of Copper(I) Complexes Bearing Diphenylphenanthroline and Bis(Diphenylphosphino)Alkane: the effect of phenyl groups on the phenanthroline ligand. Dalton Trans. 44, 7554–7562. doi: 10.1039/C5DT00835B
Worl, L. A., Duesing, R., Chen, P., Ciana, L. D., and Meyer, T. J. (1991). Photophysical properties of polypyridyl carbonyl complexes of rhenium(I). J. Chem. Soc. Dalton Trans. 849–858. doi: 10.1039/DT9910000849
Xu, S., Wang, J., Zhao, F., Xia, H., and Wang, Y. (2015). Photophysical properties of copper(I) complexes containing pyrazine-fused phenanthroline ligands: a joint experimental and theoretical investigation. J. Mol. Model. 21:313. doi: 10.1007/s00894-015-2857-0
Yamazaki, Y., Takeda, H., and Ishitani, O. (2015). Photocatalytic reduction of CO2 using metal complexes. J. Photochem. Photobiol. C. 25, 106–137. doi: 10.1016/j.jphotochemrev.2015.09.001
Keywords: Cu(I) diimine complex, CO2 reduction photocatalyst, redox photosensitizer, visible-light absorption, emission
Citation: Takeda H, Monma Y, Sugiyama H, Uekusa H and Ishitani O (2019) Development of Visible-Light Driven Cu(I) Complex Photosensitizers for Photocatalytic CO2 Reduction. Front. Chem. 7:418. doi: 10.3389/fchem.2019.00418
Received: 08 March 2019; Accepted: 21 May 2019;
Published: 06 June 2019.
Edited by:
Hitoshi Ishida, Kitasato University, JapanReviewed by:
Helene Serier-Brault, UMR6502 Institut des Matériaux Jean Rouxel (IMN), FranceYang Yang, University of Central Florida, United States
Copyright © 2019 Takeda, Monma, Sugiyama, Uekusa and Ishitani. This is an open-access article distributed under the terms of the Creative Commons Attribution License (CC BY). The use, distribution or reproduction in other forums is permitted, provided the original author(s) and the copyright owner(s) are credited and that the original publication in this journal is cited, in accordance with accepted academic practice. No use, distribution or reproduction is permitted which does not comply with these terms.
*Correspondence: Hiroyuki Takeda, dGFrZWRhaEBjaGVtLnRpdGVjaC5hYy5qcA==; dGFrZWRhaEByZWNhcC5vc2FrYS1jdS5hYy5qcA==
†Present Address: Hiroyuki Takeda, Research Center for Artificial Photosynthesis, Osaka City University, Osaka, Japan
Haruki Sugiyama, Research and Education Center for Natural Sciences, Keio University, Yokohama, Japan