- 1Université de Nantes, CNRS, Unité Fonctionnalité et Ingénierie des Protéines (UFIP), UMR 6286, Nantes, France
- 2Université de Nantes, CNRS, Chimie Et Interdisciplinarité: Synthèse, Analyse, Modélisation (CEISAM), UMR 6230, Nantes, France
- 3Department of Pharmacology, Pharmacy and Pharmaceutical Technology, Center for Research in Molecular Medicine and Chronic Diseases (CIMUS), School of Pharamacy, Health Research Institute of Santiago de Compostela (IDIS), University of Santiago de Compostela, Santiago de Compostela, Spain
- 4Unité Biopolymères Interactions Assemblages Plate-Forme BIBS, INRA, Nantes, France
Glycoconjugate vaccines are formed by covalently link a carbohydrate antigen to a carrier protein whose role is to achieve a long lasting immune response directed against the carbohydrate antigen. The nature of the sugar antigen, its length, its ratio per carrier protein and the conjugation chemistry impact on both structure and the immune response of a glycoconjugate vaccine. In addition it has long been assumed that the sites at which the carbohydrate antigen is attached can also have an impact. These important issue can now be addressed owing to the development of novel chemoselective ligation reactions as well as techniques such as site-selective mutagenesis, glycoengineering, or extension of the genetic code. The preparation and characterization of homogeneous bivalent pneumococcal vaccines is reported. The preparation and characterization of homogeneous bivalent pneumococcal vaccines is reported. A synthetic tetrasaccharide representative of the serotype 14 capsular polysaccharide of Streptococcus pneumoniae has been linked using the thiol/maleimide coupling chemistry to four different Pneumococcal surface adhesin A (PsaA) mutants, each harboring a single cysteine mutation at a defined position. Humoral response of these 1 to 1 carbohydrate antigen/PsaA conjugates have been assessed in mice. Our results showed that the carbohydrate antigen-PsaA connectivity impacts the anti-carrier response and raise questions about the design of glycoconjugate vaccine whereby the protein plays the dual role of immunogen and carrier.
Introduction
Surface exposed polysaccharides of bacterial pathogens are perceived as non-self by the host immune system. Therefore, they are often the target of a protective humoral immune response. Immunization using polysaccharides from capsulated bacteria has been introduced by Gotschlich in the late 1960s (Gotschlich et al., 1972). Capsular polysaccharides are typical T cell-independent type 2 antigens (Mond et al., 1995). This group of antigens is able to deliver prolonged and persistent signaling to the B cell through B cell receptor cross-linking. However, these vaccines fail to be active in children, the population which is the major target of the infectious diseases caused by pathogenic bacteria. A major breakthrough in the field has been later achieved by the development of the glycoconjugate vaccines (Rappuoli, 2018). A purified capsular polysaccharide or a fragment thereof or a synthetic oligosaccharide mimicking the antigenic determinants expressed by capsular polysaccharide is conjugated to a protein scaffold referred to as carrier protein. In this case, the protein moiety of the conjugate is processed by carbohydrate antigen-specific B cells after engagement of their B cell receptor. This leads to the presentation of peptides—T helper epitopes—in association with major histocompatibility complex of type II (MHCII) molecules to carrier peptide-specific CD4+ T lymphocytes. These T helper cells, in turn, stimulate the production of both plasma cells and memory B cells (Pollard et al., 2009). This vaccine strategy proved highly efficient even in infants. Consequently several vaccines active against meningococcus, streptococcus, or Haemophilus influenza type b have been launched (Berti and Adamo, 2018). Alternatively it has recently been shown that a carbohydrate epitope presented in the form of a glycopeptide by the MHCII molecules could strongly stimulate CD4+ T cells (Avci et al., 2011; Berti and Adamo, 2013). While both mechanisms probably coexist, this discovery might considerably impact the design of future glycoconjugate vaccines. Indeed, it has long been established that both length and density of the carbohydrate antigens on the carrier protein influence the immunogenicity of the conjugates in an interconnected manner. At a fixed sugar/protein ratio, the anti-carbohydrate antigen titers vary according to a bell curve as a function of density (Pozsgay et al., 1999). On the other hand, the observed optimum depends on the length of the antigen, this value being usually lowered when one increases the chain length (Anderson et al., 1989). However, if second mechanism has to be considered, the selection of the glycosylation sites is equally important. Along this line, Peng et al. have taken advantage of the propensity of flagellin to self-assemble in a supercoiled structure to selectively modify the sole lysines exposed to the solvent and thus preserving the protein properties to activate immune response (Peng et al., 2018). Stefanetti et al. recently prepared a series of glycoconjugates made of CRM197 and Salmonella O-antigen as the carrier protein and the carbohydrate antigen, respectively (Stefanetti et al., 2015). An average of one up to four O-antigen chains per protein was introduced at controlled positions. The O-antigen chains were randomly linked to surface accessible glutamic/aspartic acid or lysine residues or at more defined sites upon exploiting the kinetically favored reactivity of lysines having the lowest pKa (Crotti et al., 2014; Matos et al., 2018), the rarity of surface exposed tyrosine selectively activated (Hu et al., 2013; Nilo et al., 2014), the transglutaminase catalyzed modification of a lysine (Nilo et al., 2015b) or upon designing stapled conjugate from a reduced disulfide bond. This study was useful to demonstrate that the conjugation site plays a role in determining the immunogenicity. However, the tested formulations still contained heterogeneous mixtures of conjugates since the derivatization processes remain largely empirical. Moreover, further discrepancies arose from structural differences among the linkers used in the study although the same strain promoted azide-alkyne cycloaddition reaction was applied for the preparation of every conjugate. Such biases do not allow an unequivocal interpretation of observed results. Yet recent progress made in unnatural amino acid incorporation (Quast et al., 2015), protein glycan coupling technology (PGCT) (Ma et al., 2018) or site-selective mutagenesis (Grayson et al., 2011) offer unique opportunities to access fully defined glycoconjugate vaccines and further elucidate the relationship between carbohydrate antigen/carrier protein connectivity and immunogenicity. We report herein the preparation and the characterization of homogeneous bivalent pneumococcal conjugates. A synthetic tetrasaccharide derived from Streptococcus pneumoniae serotype 14 capsular polysaccharide equipped of a maleimido-functionalized spacer arm at its reducing end has been site-specifically attached to four different cysteine mono-mutants of the Pneumococcal surface adhesin A (PsaA).
Results and Discussion
Conjugate Design, Synthesis, and Characterization
Pneumococcal infections are still a leading cause of mortality worldwide. Available prophylactic pneumococcal glycoconjugate vaccines induce capsule-specific memory B-cells and IgG capable to prevent colonization and disease (Jochems et al., 2017). Vaccine effectiveness is considerably improved by increasing the valency e.g., from 7 up to 13 serotypes (van der Linden et al., 2016). However, inclusion of serotype-independent immunogens able to control pneumococcal carriage to these vaccines has been identified as an appealing strategy (Jochems et al., 2017). PsaA is a nasopharyngeal colonization factor which is expressed by more than 99% of pneumococcal strains in a highly conserved form (Rajam et al., 2008a). These features have thus designed PsaA as a possible protein immunogen candidate (Wang et al., 2010; Gor et al., 2011; Olafsdottir et al., 2012; Lu et al., 2015). Concomitant administration of PsaA with PCV7 was accompanied with reduced colonization in a murine model (Whaley et al., 2010) and its protective effect in association with a panel of pneumococcal protein immunogens later assessed in phase I clinical trials (Schmid et al., 2011; Entwisle et al., 2017). Moreover, the successful use of PsaA both as an immunogen and a carrier protein PsaA by several laboratories including ours in mice models further encouraged us to select it as a model protein (Lin et al., 2010; Chen et al., 2016; Prasanna et al., 2019). Mature PsaA (mPsaA) i.e., PsaA deprived from its signal peptide, was therefore conjugated to the tetrasaccharide β−D−Galp−(1 → 4)−β−D−Glcp−(1 → 6)−[β−D−Galp−(1 → 4)]β-D-GlcpNAc 1 (referred to as Pn14TS) (Figure 1A). This easily synthesized tetrasaccharide is the minimal structure from the capsule of serotype 14, one of the prevalent pneumococcal serotype which has developed high antibiotic resistance (Yahiaoui et al., 2018), able to induce functional antibodies (Abs) (Safari et al., 2008). We observed that while high anti-mPsaA Ab titers were induced in mice immunized with mPsaA alone, this response was much lower when mice where immunized with a conjugate whereby Pn14TS was randomly coupled to surface-exposed lysine side-chains of mPsaA at a 5.4:1 average value for carbohydrate:protein ratio (Prasanna et al., 2019). This observation might result from mPsaA B epitope masking due to Pn14TS conjugation on the protein, immune-dominance of antigenic determinants expressed by Pn14TS over the mPsaA B epitopes or different processing of free vs. conjugated mPsaA. Developing an access to homogeneous Pn14TS-mPsaA conjugates will therefore provide tools to address these issues and document structure-immunogenicity relationships useful for future pneumococcal vaccine design.
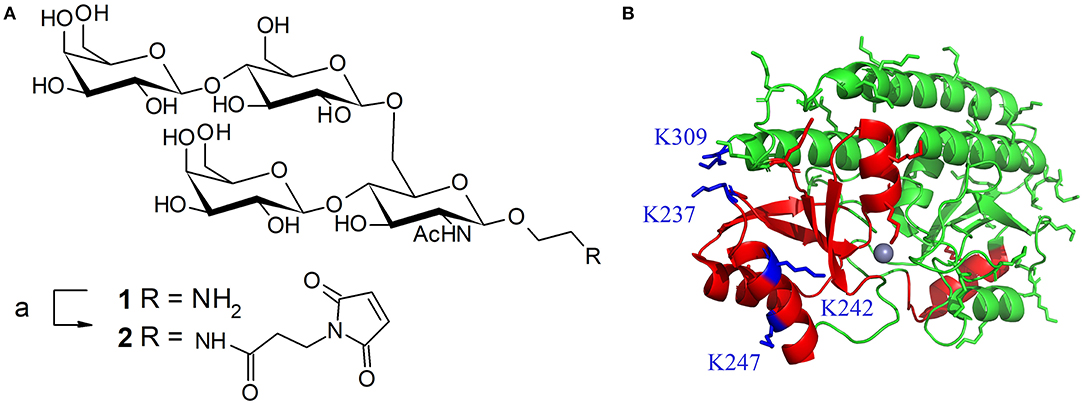
Figure 1. (A) Structure of tetrasaccharide antigen 1 from S. pneumoniae serotype 14 capsule and its activation with a maleimide linker; reagents and conditions: (a) 3-maleimidopropionic acid N-hydrosysuccimide ester (1.45 equiv), DIEA (2 equiv), DMF, RT, overnight, 58%. (B) Ribbon diagram of PsaA (green). Regions containing known Th and B-epitopes are colored in red. Lysine side-chain are represented in green except the four lysines targeted for mutagenesis (in blue). Representation based on the 1PSZ PDB file, with a resolution of 2.0 Å (Lawrence et al., 1998).
mPsaA is a 32.4 kDa-protein which corresponds to amino acids 21-309 of PsaA sequence. mPsaA essentially adopts alpha-helical secondary structures and contains 37 lysines, most of them accessible for conjugation (Figure 1B) (Couñago et al., 2014). Interestingly, mPsaA does not contain any cysteine residue which makes site-selective cysteine mutagenesis an attractive approach to envision homogenous mPsaA conjugate synthesis (Grayson et al., 2011). Previous studies based on secondary structure predictions, endopeptidase site analyses and in silico MCHII peptide-binding affinity screening helped identifying a panel of 24 putative PsaA T-helper epitopes. Three out of them proved to be able to provoke Th cell proliferation: PsaA67−82, PsaA199−221, and PsaA231−268. The last one was deduced from three potent overlapping 15-mer peptides among which sequence 243–257 was the most potent (Singh et al., 2014). Identification of PsaA B epitopes has also been carried out using a phage display peptide library and monoclonal Abs. Two sequences in the region 132–146 and 253–267 showed promises for their immunogenicity in mice noticeably for reducing carriage and colonization (Srivastava et al., 2000; Johnson et al., 2002). Further studies have demonstrated that the sequence PsaA251−278 was involved in PsaA-mediated adherence of S. pneumoniae to epithelial cells (Romero-Steiner et al., 2006; Rajam et al., 2008b). In view of these data, we elected to mutate C-terminal lysine 309 located in an apparently non-relevant region for immunity into a cysteine. The preparation of three additional cysteine mutants at K237, K242, and K247 i.e., within or close to the potent T-helper epitope was also envisaged (Figure 1B).
We have recently reported the production in Escherichia coli BL21(DE3) of the mPsaA N-terminated by a poly-6-histidine tag sequence to facilitate its purification by immobilized-Ni affinity chromatography plus a Tabacco Etch Protease (TEV) specific cleavage site to remove the tag after purification (Prasanna et al., 2019). mPsaA production level has been increased by 5–6 fold upon adopting time/temperature/induction conditions reported by Laurentis et al. and replacing the LB by the TB growth medium (Supplementary Figure 1 and Supplementary Table 1) (Larentis et al., 2011). The four mutants have been produced accordingly after having introduced every desired mutation in the original sequence using the QuickChange method. Culture have been carried out at a 250 ml scale and yielded about 19 mg of each mutant after purification and histidine tag removal (Figure 2).
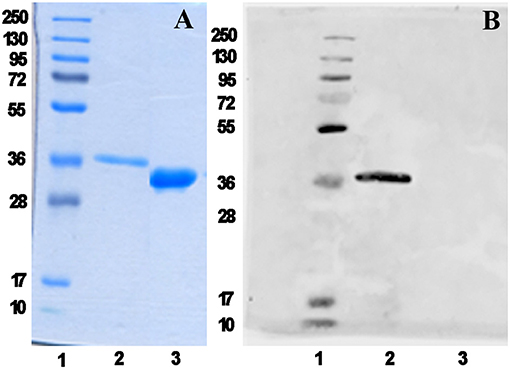
Figure 2. mPsaA K309C characterization as a representative example. Analysis of histidine tag removal from mutant mPsaA K309C by SDS-PAGE (A) and by Western blot (B). Lane 1: unstained protein marker; Lane 2: tagged mPsaA K309C; Lane 3: purified mPsaA K309C after removal of poly-histidine tag.
Having the four mutants in hand we next examined their respective conjugation with the carbohydrate antigen. To this aim, known tetrasaccharide 1 (Prasanna et al., 2019) was derivatized with a maleimide linker to provide 2 in 58% yield following RP-HPLC purification (Figure 1A). The four mutants were treated separately with DTT to reduce any inter-protein disulfide bond which might have formed during their preparation and further reacted with no more than 5 equivalent of 2 in degassed PBS, pH 7.0 at room temperature overnight. Each conjugate was then subjected to gel filtration purification to remove excess tetrasaccharide (Figure 3) and freeze-dried for storage. Gel filtration chromatography profiles of conjugates associated to mPsaA K237C, K242C, and K247C were very similar and essentially composed of a single peak. A second unidentified peak which is eluted earlier is apparent in the Pn14TS-mPsaA K309C profile (Figure 3). We first thought that it corresponds to a dimer of the mPsaA K309C whose formation had been favored due to a greater exposure of the cysteine at the C-terminus. However, mass spectrometry experiments ruled out this hypothesis. Effectiveness of the conjugation was checked by gel electrophoresis which uniformly showed that spots are shifted toward higher molecular weight, compatible with the attachment of a single tetrasaccharide (Figure 4A). These results were confirmed by MALDI mass spectrometry experiments which indicate that parent mutant mPsaA proteins were incremented by 896–906 mass unit (calculated +901 Da) within the error range mass measurements (Figure 4B). In the end homogenous 1:1 carbohydrate antigen/carrier protein K237C, K242C, K247C, K309C conjugates have been obtained in 55, 36, 44, and 30% yield, respectively, with a purity superior to 90%.
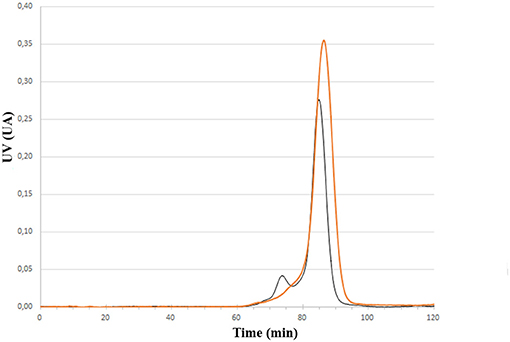
Figure 3. Comparison of size exclusion chromatography profile of Pn14TS-mPsaA K247C (red trace) and Pn14TS-mPsaA K309C (Black trace) conjugates. Column HiLoad 15/600 Superdex™ 75 pg (GE Healthcare) column and PBS 0.1 M, pH 7.3 as eluent.
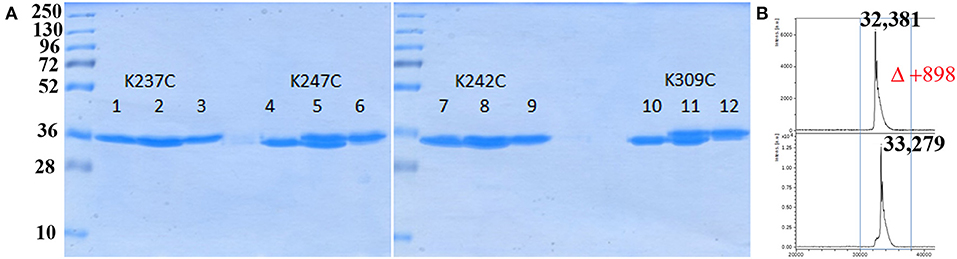
Figure 4. Analysis of bioconjugation efficiency by SDS PAGE and mass spectrometry. (A) Comparison of each mPsaA mutant before, mix of before and after, and after conjugation. Lanes 1–3: mPsaA K237C; Lanes 4–6: mPsaA K247C; Lanes 7–9: mPsaA K242C; Lanes 10–12: mPsaA K309C. Two micrograms protein sample/lane, 12% SDS-PAGE, 100 V, 2 h; (B) MALDI MS spectrum of mPsaA K309C (top spectrum) and Pn14TS-mPsaA K309C (bottom spectrum).
Humoral Response Evaluation
Groups of five C57/BL6 mice were immunized thrice at 2 weeks interval with each of the four conjugates and PBS as a negative control formulated in chitosan and Ribi as adjuvant. The antibody response against both mPsaA and capsular polysaccharide of S. pneumoniae serotype 14 (CP14), was then determined by ELISA assays 1 week after the second and third immunization (i.e., on Days 21 and 35). The IgG response raised against CP14 by the conjugates was very low and not significant compared to the negative control group (data not shown). This response was equally low when we used Pn14TS as the coating antigen indicating that the observed results were not due to a lack of recognition of CP14 by anti-Pn14TS Abs. Anti-CP14 IgM response was also determined after the second and the third immunizations. Low titers of anti-CP14 IgM Abs could be measured in the sera of mice for any of the tested formulations after the second immunization (Supplementary Figure 2A). Highest titers of anti-CP14 Abs seem to be induced by Pn14TS-mPsaA K247C conjugate in comparison with other conjugates and PBS. Pn14TS-mPsaA K309C gave rise to the weakest response comparable to that induced by PBS. However, these tendencies were not statistically significant. Anti-IgM responses further diminished after the boost although the expected IgM to IgG switch was not observed (Supplementary Figure 2B). In fact, we and other experienced in the past that Pn14Ts was able to efficiently mimic native CP14 (Safari et al., 2008; Kurbatova et al., 2017; Prasanna et al., 2019). However, as mentioned in the introduction, it is assumed that the level of the anti-carbohydrate antigen response depends on intricate related parameters such as carbohydrate antigen length, carbohydrate antigen:carrier (S/P) ratio and administered sugar dose (Pozsgay et al., 1999). For examples, significant humoral response has been previously observed against Pn14TS: this hapten being used at a S/P ratio of 4, at a 2.5 μg dose using adipic acid coupling chemistry and CRM197 as the carrier (Mawas et al., 2002); at a S/P ratio of 4.8 or 6, at a 2.5 μg dose, using squarate coupling chemistry and CRM197 as carrier protein (Mawas et al., 2002; Safari et al., 2008); at a S/P ratio of 11, at 1.25–10 μg dose, using squarate chemistry and BSA as carrier; at a S/P ratio of 5.4, at 3 μg dose using thio/maleimide coupling chemistry and PsaA as carrier protein. Testing of Pn14TS at a S/P of 1 is unprecedented. Absence of anti-CP14 IgG response might be circumvented upon increasing the length of the antigen. Effective response was nicely observed when a dodecasaccharide (corresponding to 3 × Pn14TS units), conjugated to CRM197 was used in a 1:1 carbohydrate antigen/carrier protein ratio (Safari et al., 2008). Increasing the administered dose could also been envisaged. A very low amount of carbohydrate antigens (0.5 μg/dose/mouse) was indeed used for immunizations. This value was chosen to keep the amount of injected mPsaA equal to 25 μg/dose/mouse and remaining coherent with our previous experiments (Prasanna et al., 2019). The adopted strategy was nevertheless applicable to the investigation of the anti-mPsaA response. IgM Ab response was absent in all tested sera in agreement with our previous findings (data not shown) (Prasanna et al., 2019). Contrasting with these results a significant response was observed in the secondary sera of mice immunized with the conjugates in comparison with the control group (P < 0.05) (Figure 5A). Level of anti-mPsaA IgG titers was further raised up to 1/8,000–1/64,000 for all sera after the third immunization. The highest titers were observed in sera of mice immunized with Pn14TS-mPsaA K242C and K309C. Noticeably, anti-mPsaA IgG Abs induced by the later conjugate was significantly higher than those induced by Pn14TS-mPsaA K237C or Pn14TS-mPsaA K247C (P < 0.05) but not Pn14TS-mPsaA K242C (Figure 5B). We have recently shown that mPsaA was highly immunogenic in mice while introduction of only five tetrasaccharide haptens by classic lysine random conjugation severely impaired its immunogenicity (Prasanna et al., 2019). Maintaining the protective properties of the mPsaA epitopes is of paramount importance if a dual role of both vaccine immunogen and carrier for carbohydrate antigens is envisaged. Dual anti-Group B Streptococcus glycoconjugate vaccines have been prepared using transglutaminase or tyrosine-directed conjugation technology. This coupling strategy was shown to preserve the antigenicity of the Streptococcus proteins used as the carriers (Nilo et al., 2014). Immunogenicity of the conjugates with defined connectivity was essentially comparable to that observed for conjugates obtained upon conjugation of surface-exposed lysine side-chains (Nilo et al., 2015a,b). In a different study, PGCT was applied to the controlled transfer of E. coli O157:H7 O-polysaccharide to the asparagine residue amino acid sequence (DQNAT)4 introduced at the C-terminus of the maltose-binding protein used as carrier protein model (Ma et al., 2018). The glycosylation had slight interference on the global anti-MBP response. However, for the first time it has been shown that the carbohydrate antigen decreased the response against the peptides containing or adjacent to the polysaccharide but not against peptide at distal site. Our results are in agreement with these data. Immunogenicity of mPsaA is globally conserved but finely tuned by the grafting of a single carbohydrate antigen. The highest anti-mPsaA response is observed when Pn14TS is introduced at position 309 of mPsaA i.e., in a peptide segment distant from the identified T-helper epitope and not when it is introduced at position 237, 242, or 247. This result makes sense if mPsaA B-cell epitopes and Pn14TS antigen compete for the assistance of the same T-helper epitopes. CRM197 remains the best carrier protein develop so far for use in humans. Structural alteration of the epitopes expressed by CRM197 during conjugation and detoxification processes have been proposed to explain its superiority as a carrier protein over diphtheria toxoid or tetanus toxoid (Pecetta et al., 2015). Beyond, if one cannot improve the response against one antigen at the detriment of the response against another equally important one then the strategy consisting in using a protein with dual role of antigen and carrier is questionable. Consistent with this assumption, the weakest anti-Pn14TS response was measured in sera of mice immunized with the Pn14TS-mPsaA K309C conjugate. However, further studies are required to confirm this tendency since the anti-CP14 titers were weak and limited to IgM Abs. In a study based on the use of group B streptococcal type III polysaccharide, it has been calculated that approximately eight repeat units of a polysaccharide branched to a T-helper peptide could be presented in complex with a MCHII molecule to a T-cell receptor (TCR) (Avci et al., 2011). We herein elected to work with a short tetrasaccharide antigen. It is conceivable that a TCR can recognize both our small carbohydrate antigen and part of the T-helper peptide sequence even though this is not yet demonstrated. Access to longer synthetic oligosaccharides is more demanding from the synthetic aspect and not necessarily associated with improved antigenicity (Safari et al., 2008). Also the use of purified CP will be accompanied by a loss of conjugate homogeneity while complexing its immune investigation. However, administration of a higher dose of sugar might improve the response against the Pn14TS and allow assessment of the variation of both anti-sugar and anti-protein responses. Alternatively, such study could be envisaged upon coupling not one but several carbohydrate antigen at controlled positions. Further work is also needed to assess the protective properties of the Abs and to investigate in detail the B-cell response, in particular which part of the protein is targeted by the humoral response.
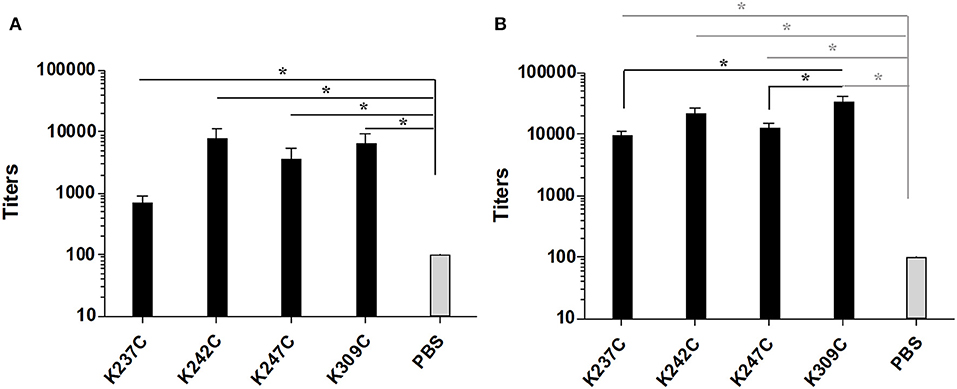
Figure 5. Titers of anti-mPsaA (coated on microtiter plates) IgG Abs of mice immunized with Pn14TS-mPsaA K237C, K242C, K247C, K309C, PBS 1 week after the 2nd (J21) (A) and the 3rd (J35) immunization (B). The serum samples data presented as geometric mean titer ± standard deviation of five mice per group. Statistical analysis was performed using one-way ANOVA with Tukey analysis for multiple comparisons. Statistical difference between the groups is *P < 0.05.
Conclusion
The development of technologies based on site-directed or site-specific conjugation for example relying on cysteine mutagenesis as exemplified in this study can lead to fully characterized glycoconjugate vaccines while ensuring higher batch-to-batch reproducibility. Moreover, it offers unique opportunity to study structure-immunogenicity relationship while unraveling molecular aspects of the immune response and giving rise to an optimized generation of glycoconjugate vaccines.
Experimental Section
Mutagenesis
Mutations K237C, K242C, K247C, and K309C have been performed by PCR amplification Phusion polymerase (Thermo Scienyific), followed by DpnI digestion using the following primers: K237C forward 5′-GCACCCCGGAGCAAATCTGCACCCTGGTGGAAAAGC, reverse 5′-GCTTTTCCACCAGGGTGCAGATTTGCTCCGGGGTGC; K242C forward 5′-CAAATCAAAACCCTGGTGGAATGCCTGCGTCAGACCAAAGTTCCG, reverse 5′-CGGAACTTTGGTCTGACGCAGGCATTCCACCAGGGTTTTGATTTG; K247C forward 5′- GAAAAGCTGCGTCAGACCTGCGTTCCGAGCCTGTTCGTG, reverse 5′-CACGAACAGGCTCGGAACGCAGGTCTGACGCAGCTTTTC; K309C forward 5′-GGAGGGTCTGGCGTGCTAAGGATCCGGC, reverse 5′-GCCGGATCCTTAGCACGCCAGACC CTCC. The plasmids obtained are then transformed into competent XL1 blue bacteria. For each mutation, extraction of the plasmid (Quiaprep®Miniprep from Quiagen) on three different clones is carried out according to manufacturer protocol, and insertion of the mutations checked by sequencing.
Mutant mPsaA Expression
The validated plasmids have been used to transform E. coli BL21 (DE3) strains for expression of the different mutants. The expression of mPsaA mutant proteins was performed according to the following protocol. Briefly, 250 mL of fresh TB medium with 100 μg/ml ampicillin were inoculated with 0.5 mL of overnight pre-culture and left to grow at 37°C, 180 rpm until they reach the exponential phase (approximately OD of 0.6/0.7 is reached) and at this point the HtTEV-mPsaA expression in the cultures were induced with IPTG (0.1 mM final concentration). The cultures were left to grow for another 16 h at 25°C and 180 rpm. The cells were harvested at 10,000 rpm. The pellet were re-suspended in 25 ml cold lysis buffer (50 mM NaH2PO4, 150 mM NaCl, pH 8.0 + 5 mM Imidazole + 1 mM PMSF) + 1 μg/mL DNAse, and 1 mg/mL of lysozyme incubated at 4°C under stirring during 30 min and were subjected to sonication (7 min, 50% amplitude, pulse of 5 s ON/OFF) while they were kept in ice, and the cell debris were removed by centrifugation (30 min, 13,000 rpm). The obtained supernatant was filtered (0.45 μm) and incubated with 0.5 ml of preconditioned Ni NTA beads for 1 h on rotating disk at 4°C, 12 rpm. Unbound fraction was collected, and the beads were washed with a lysis buffer containing 2–10 mM imidazole. The elution was performed with a lysis buffer containing 300 mM imidazole. The eluted fractions were subjected to SDS-PAGE to identify the fractions containing target protein. Later, the eluted fractions, containing the target protein (100 mg/L of culture), were pooled and dialyzed against water at 4°C.
Removal of Poly-6-Histidine Tag and Western Blot Analysis
The enzymatic cleavage of the histidine tag from mutants was performed using a 5:1 HtTEV-mPsaA/AcTEVTM protease ratio in the reaction buffer (50 mM Tris-HCl (pH 7.6), 1 mM EDTA, 1 mM DTT), for 16 h at 20°C, without stirring. The crude reaction mixtures were then dialyzed against water and incubated with the Ni NTA beads for the separation of the cleaved mPsaA mutants from the histidine tag, parent mPsaA mutants if not entirely digested and the tagged TEV protease. The eluate was collected and was subjected to SDS-PAGE and western blot analyses to confirm the removal of the histidine tag and assess their purity.
For the western blot, the vertical SDS-PAGE was carried out using 12% acrylamide gels with the loadings of the reaction mixture and tagged mPsaA mutants as controls using the Bio-rad system. The proteins were transferred to a nitrocellulose membrane at 150 mA for 90 min. For the antibody probing, the nitrocellulose membrane was initially blocked with TBS containing 5% skim milk and 0.1% tween 20 for 1 h at RT. A primary antibody to mouse anti-poly histidine (Sigma H1029, diluted 1:1,000), was applied to the membrane and incubated for 16 h at RT with agitation. The membranes were washed thrice with PBS containing 0.1% tween 20 and incubated with secondary antibody (Goat anti-Mouse IgG (H+L) Secondary Antibody, Alexa Fluor 680) for 1 h at RT on a shaker. Membranes were subjected to a final wash with PBS, and the detection was performed using an Odyssey CLx scanner (LI-COR) at 700 nm.
2-(N-3-maleimidopropanoyl)ethyl(β−d−galactopyranosyl)−(1 → 4)−(β−d−galactopyranosyl)−(1 → 6)−[(β−d−galactopyranosyl)−(1 → 4)]−2−deoxy−2−acetamido−β-d-glucopyranoside 2
To a solution of 2-amino-ethyl (β−D−galactopyranosyl)−(1 → 4)−(β−D−galactopyranosyl)−(1 → 6)−[(β−D−galactopyranosyl)−(1 → 4)]−2−deoxy−2−acetamido−β-D-glucopyranoside 1 (17.5 mg, 0.023 mmol, 1 equiv) in DMF were successively added 3-maleimido-propionic acid succinimidyl ester (9 mg, 1.45 equiv) and DIEA (8.30 μL, 2 equiv) at RT. The reaction mixture was stirred at RT overnight, diluted in water and freeze-dried. The crude residue was purified by RP-HPLC to provide 2 (12.2 mg, 58% yield); Rf 0.39 (nBuOH/EtOH/H2O 5:5:3); 1H NMR (400 MHz, D2O): δ 6.85 (s, 2H), 4.52 (d, J = 8.2 Hz, 1H), 4.51 (d, J = 7.8 Hz, 2H), 4.43 (d, J = 7.8 Hz, 1H), 4.26 (dd, J = 1.9, 11.7 Hz, 1H), 3.99–3.88 (m, 4H), 3.84–3.62 (m, 20H), 3.60–3.55 (m, 1H), 3.54–3.48 (m, 2H), 3.36 (ddd, J = 2.5, 7.8, 9.8 Hz, 1H), 3.28 (t, J = 4.1 Hz, 2H), 2.48 (t, J = 7.0 Hz 2H), 2.00 (s, 3H); 13C NMR (100 MHz, D2O): δ 174.7, 173.5, 172.6, 134.5, 103.0, 102.8, 102.5, 101.2, 78.5, 77.9, 75.4, 75.3, 74.7, 74.3, 73.5, 72.7, 72.6, 72.6, 72.4, 71.0, 71.0, 68.9, 68.6, 68.2, 67.4, 61.1, 61.0, 60.2, 55.1, 39.3, 34.7, 34.5, 22.3; HR-ESI-MS: m/z Calcd for C35H55N3O24[M+Na]+ 924.3073, found 924.3088.
Glycoconjugate Syntheses
mPsaA mutants proteins are rehydrated with ultrapure water to reconstitute 50 mM phosphate buffer. The pH are tested and adjusted to pH 8 with NaOH 0.1 M if necessary. mPsaA solutions are then treated with DTT (1.6 mg/mg of protein). Solutions are mixed first by flush and next let in rotary shaker at room temperature for 15 min. DTT is eliminated with desalting column (Zeba Spin, 7 MWCO) prior equilibrated with degassed pH 7 PBS buffer using swing centrifuge. Protein contents are quantified using Nanodrop spectrophotometer at 280 nm prior their conjugation. To a solution of mPsaA mutants (4.8–7.2 mg) in degassed 40 mM PBS, pH 7.0 (1.8–2.4 mg/mL), was added 2 (5 equiv) dissolved in water (1 mg/mL), and the resulting mixture stirred overnight and then purified by gel filtration using a HiLoad 15/600 Superdex™ 75 pg (GE Healthcare) column and 0.1 M PBS, pH 7.3 as eluent at 0.8 ml/min. Collected fractions were concentrated by centrifugal concentrators (cut-off 3 MWCO) (Vivaspin; 1 h 7,000 g 4°C) and then freeze-dried to give the corresponding conjugates which were analyzed for identity and purity by gel electrophoresis and mass spectrometry.
Immunizations
The conjugates (40 μl of a 5 mg/ml solution in PBS), were first formulated with a mixture of chitosan/poloxamer/TPP (1:1:1) (6 mL) under stirring (600 rpm) for 30 min. The particles were concentrated by centrifugation at 12000 RCF for 12 min at 15°C, using 10 μL of glycerol bed. After the centrifugation, the pellet in the bottom is carefully collected and re-suspended in PBS (50 μL). The Groups of 5 male C57/BL6JRj (5 week old) mice were injected sub-cutaneously (s.c.) with PBS, Pn14TS-mPsaA K237C, Pn14TS-mPsaA K242C, Pn14TS-mPsaA K247C, or Pn14TS-mPsaA K309C (25 μg protein/dose-−0.5 μg tetrasaccharide/dose) diluted with 50 μL of RIBI in PBS. The mice were immunized at day 0, 14, and 28. The sera were collected on days 21 and 35. Sera were stored at −80°C.
Measurement of Humoral Response
The Ab responses induced upon immunizations were assessed 1 week after the second and the third injections by ELISA. mPsaA and capsular polysaccharide serotype 14 (CP14) (Alliance Bio Expertise), were used as coated antigens to define the anti-mPsaA or anti-CP14 Ab titers. mPsaA (0.1 μg/well) in 10 mM PBS, pH 7.3 (100 μL/well), was coated on 96 wells microtiter plates Nunc Maxisorp (ThermoFisher Scientific) plates overnight at 4°C. CP14 (1 μg/well) was coated for 48 h at 4°C in 10 mM PBS, pH 7.3 (100 μL/well). Plates were then washed with PBS 0.05% Tween 20 (3 × 200 μL), saturated using PBS containing 10% skimmed milk at 37°C for 2 h, then washed using PBS Tween 20 (PBST, 50 mM Tris, 150 mM NaCl, 0,1% Tween 20) (3 × 200 μL). Series of dilution of sera in PBS containing 10% skimmed milk (100 μL/well), were incubated at 37°C for 2 h. Plates were then washed with PBST (3 × 200 μL) and then incubated with goat anti-mouse IgG(H+L)-horse radish peroxidase-labeled conjugate (CliniSciences) used as secondary Ab at a dilution of 1/6,000, for 1 h at 37°C and further washed with PBST (5 × 200 μL). The enzyme substrate, o-phenylenediamine dihydrochloride (100 μL at 0.4 mg mL−1) in 0.1 M sodium citrate (pH 5.2), containing 0.02% hydrogen peroxide, was added to each well and the plate incubated for 20 min at RT in the dark. The reaction was terminated by adding 3 M HCl (1,000 μL per well), and the A492 was read in an Infinite M1000 spectrophotometer (TECAN). The Ab titer was defined as the dilution of immune serum that gave an OD (405 nm) at least twice that observed with pre-immune serum.
Data Availability Statement
All datasets generated for this study are included in the article/Supplementary Material.
Ethics Statement
The animal study was reviewed and approved by ethical permit number from Comité d'Ethique en Expérimentation Animale (CEEA): 7897.
Author Contributions
CG designed the research and wrote the manuscript with AP, AD, and AL. MPr developed the route for the production of PsaA under EC, NC, and CG supervision. AP and AD produced the PsaA mutants under MPi, TV, and EC guidance. AL and CG carried out the conjugation step and the purification of the conjugates. AP and AF performed the ELISA assays. MF assisted in mass measurements.
Funding
AP, MPr, and TV acknowledge their respective doctoral fellowship from the Région Pays de la Loire/Université de Nantes, under the GlycoOuest Program, from the European Commission, Education, Audiovisual and Culture Executive Agency (EACEA), under the Erasmus Mundus program, NanoFar and from the Région Pays de la Loire, under the Pari Scientifique BioSynProt program.
Conflict of Interest
The authors declare that the research was conducted in the absence of any commercial or financial relationships that could be construed as a potential conflict of interest.
Acknowledgments
The authors gratefully acknowledge Dorian Caudal and Aude Lafoux from platform Therassey (Nantes) for animal experiments.
Supplementary Material
The Supplementary Material for this article can be found online at: https://www.frontiersin.org/articles/10.3389/fchem.2019.00726/full#supplementary-material
References
Anderson, P. W., Pichichero, M. E., Stein, E. C., Porcelli, S., Betts, R. F., Connuck, D. M., et al. (1989). Effect of oligosaccharide chain length, exposed terminal group, and hapten loading on the antibody response of human adults and infants to vaccines consisting of haemophilus influenzae type b capsular antigen unterminally coupled to the diphtheria protein CRM197. J. Immunol. 142, 2464–2468.
Avci, F. Y., Xiangming, L., Moriya, T., and Kasper, D. L. (2011). A mechanism for glycoconjugate vaccine activation of the adaptive immune system and its implications for vaccine design. Nat. Med. 17, 1602–1609. doi: 10.1038/nm.2535
Berti, F., and Adamo, R. (2013). Recent mechanistic insights on glycoconjugate vaccines and future perspectives. ACS Chem. Biol. 8, 1653–1663. doi: 10.1021/cb400423g
Berti, F., and Adamo, R. (2018). Antimicrobial glycoconjugate vaccines: an overview of classic and modern approaches for protein modification. Chem. Soc. Rev. 47, 9015–9025. doi: 10.1039/C8CS00495A
Chen, Z., Guo, R., Xu, J., and Qiu, C. (2016). Immunogenicity and protective immunity against otitis media caused by pneumococcus in mice of Hib conjugate vaccine with PsaA protein carrier. Front. Med. 10, 490–498. doi: 10.1007/s11684-016-0470-y
Couñago, R. M., Ween, M. P., Begg, S. L., Bajaj, M., Zuegg, J., O'Mara, M. L., et al. (2014). Imperfect coordination chemistry facilitates metal ion release in the Psa permease. Nat. Chem. Biol. 10, 35–41. doi: 10.1038/nchembio.1382
Crotti, S., Zhai, H., Zhou, J., Allan, M., Proietti, D., Pansegrau, W., et al. (2014). Defined conjugation of glycans to the lysines of CRM197 guided by their reactivity mapping. Chembiochem 15, 836–843. doi: 10.1002/cbic.201300785
Entwisle, C., Hill, S., Pang, Y., Joachim, M., McIlgorm, A., Colaco, C., et al. (2017). Safety and immunogenicity of a novel multiple antigen pneumococcalvaccine in adults: a Phase 1 randomised clinical trial. Vaccine 35, 7181–7186. doi: 10.1016/j.vaccine.2017.10.076
Gor, D. O., Ding, X., Li, Q., Sultana, D., Mambula, S. S., Bram, R. J., et al. (2011). Enhanced immunogenicity of pneumococcal surface adhesin A (PsaA) in mice via fusion to recombinant human B lymphocyte stimulator (BLyS). Biol. Direct 6:9. doi: 10.1186/1745-6150-6-9
Gotschlich, E. C., Rey, M., Triau, R., and Sparks, K. J. (1972). Quantitative determination of the human immune response to immunization with meningococcal vaccines. J. Clin. Invest. 51, 89–96. doi: 10.1172/JCI106801
Grayson, E. J., Bernardes, G. J. L., Chalker, J. M., Boutureira, O., Koeppe, J. R., and Davis, B. J. (2011). A coordinated synthesis and conjugation strategy for the preparation of homogeneous glycoconjugate vaccine candidates. Angew. Chem. Int. Ed. 50, 4127–4132. doi: 10.1002/anie.201006327
Hu, Q.-Y., Allan, M., Adamo, R., Quinn, D., Zhai, H., Wu, G., et al. (2013). Synthesis of a well-defined glycoconjugate vaccine by a tyrosine-selective conjugation strategy. Chem. Sci. 4, 3827–3832. doi: 10.1039/c3sc51694f
Jochems, S. P., Weiser, J. N., Malley, R., and Ferreira, D. M. (2017). The immunological mechanisms that control pneumococcal carriage. PLoS Pathog. 13:e1006665. doi: 10.1371/journal.ppat.1006665
Johnson, S. E., Dykes, J. K., Jue, D. L., Sampson, J. S., Carlone, G. M., and Ades, E. W. (2002). Inhibition of pneumococcal carriage in mice by subcutaneous immunization with peptides from the common surface protein pneumococcal surface adhesin A. J. Infect.Dis. 185, 489–496. doi: 10.1086/338928
Kurbatova, E. A., Akhmatova, N. K., Akhmatova, E. A., Egorova, N. B., Yastrebova, N. E., Sukhova, E. V., et al. (2017). Neoglycoconjugate of tetrasaccharide representing one repeating unit of the Streptococcus pneumoniae type 14 capsular polysaccharide induces the production of opsonizing IgG1 antibodies and possesses the highest protective activity as compared to hexa- and octasaccharide conjugates. Front. Immunol. 8:659. doi: 10.3389/fimmu.2017.00659
Larentis, A. L., Corrêa Argondizzo, A. P., dos Santos Esteves, G., Jessouron, E., Galler, R., and Medeiros, M. A. (2011). Cloning and optimization of induction conditions for mature PsaA (Pneumococcal Surface Adhesin A) expression in Escherichia coli and recombinant protein stability during long-term storage. Protein Exp. Purif. 78, 38–47. doi: 10.1016/j.pep.2011.02.013
Lawrence, M. C., Pilling, P. A., Epa, V. C., Berry, A. M., Ogunniyi, A. D., and Paton, J. C. (1998). The crystal structure of pneumococcal surface antigen PsaA reveals a metal-binding site and a novel structure for a putative ABC-type binding protein. Structure 6, 1553–1561. doi: 10.1016/S0969-2126(98)00153-1
Lin, H., Lin, Z., Meng, C., Huang, J., and Guo, Y. (2010). Preparation and immunogenicity of capsular polysaccharide-surface adhesin A (PsaA) conjugate of Streptococcus pneumoniae. Immunobiology 215, 545–550. doi: 10.1016/j.imbio.2009.08.008
Lu, J., Sun, T., Wang, D., Dong, Y., Xu, M., Hou, H., et al. (2015). Protective immune responses elicited by fusion protein containing PsaA and PspA fragments. Immunol. Invest. 44, 482–496. doi: 10.3109/08820139.2015.1037956
Ma, Z., Zhang, H., Wang, P. G., Liu, X.-W., and Chen, M. (2018). Peptide adjacent to glycosylation sites impacts immunogenicity of glycoconjugate vaccine. Oncotarget 9, 75–82. doi: 10.18632/oncotarget.19944
Matos, M. J., Oliveira, B. L., Martínez-Sáez, N., Guerreiro, A., Cal, P. M. S. D., Bertoldo, J., et al. (2018). Chemo- and regioselective lysine modification on native proteins. J. Am. Chem. Soc. 140, 4004–4017. doi: 10.1021/jacs.7b12874
Mawas, F., Niggemann, J., Jones, C., Corbel, M. J., Kamerling, J. P., and Vliegenthart, J. F. (2002). Immunogenicity in a mouse model of a conjugate vaccine made with a synthetic single repeating unit of type 14 pneumococcal polysaccharide coupled to CRM197. Infect. Immun. 70, 5107–5114. doi: 10.1128/IAI.70.9.5107-5114.2002
Mond, J. J., Lees, A., and Snapper, C. M. (1995). T cell-independent antigens type 2. Ann. Rev. Immunol. 13, 655–692. doi: 10.1146/annurev.iy.13.040195.003255
Nilo, A., Allan, M., Brogioni, B., Proietti, D., Cattaneo, V., Crotti, S., et al. (2014). Tyrosine-directed conjugation of large glycans to proteins via copper-free click chemistry. Bioconj. Chem. 25, 2105–2111. doi: 10.1021/bc500438h
Nilo, A., Morelli, L., Passalacqua, I., Brogioni, B., Allan, M., Carboni, F., et al. (2015a). Anti-group B streptococcus glycan-conjugate vaccines using pilus protein GBS80 as carrier and antigen: comparing lysine and tyrosine-directed conjugation. ACS Chem. Biol. 10, 1737–1746. doi: 10.1021/acschembio.5b00247
Nilo, A., Passalacqua, I., Fabbrini, M., Allan, M., Usera, A., Carboni, F., et al. (2015b). Exploring the effect of conjugation site and chemistry on the immunogenicity of an anti-group B streptococcus glycoconjugate vaccine based on GBS67 pilus protein and type V polysaccharide. Bioconj. Chem. 26, 1839–1849. doi: 10.1021/acs.bioconjchem.5b00480
Olafsdottir, T. A., Lingnau, K., Nagy, E., and Jonsdottir, I. (2012). Novel protein-based pneumococcal vaccines administered with the Th1-promoting adjuvant IC31 induce protective immunity against pneumococcal disease in neonatal mice. Infect. Immun. 80, 461–468. doi: 10.1128/IAI.05801-11
Pecetta, S., Lo Surdo, P., Tontini, M., Proietti, D., Zambonelli, C., Bottomley, M. J., et al. (2015). Carrier priming with CRM 197 or diphtheria toxoid has a different impact on the immunogenicity of the respective glycoconjugates: biophysical and immunochemical interpretation. Vaccine 33, 314–320. doi: 10.1016/j.vaccine.2014.11.026
Peng, C.-J., Chen, H.-L., Chiu, C.-H., and Fang, J.-M. (2018). Site-selective functionalization of flagellin by steric self-protection: a strategy to facilitate flagellin as a self-adjuvanting carrier in conjugate vaccine. Chembiochem 19, 805–814. doi: 10.1002/cbic.201700634
Pollard, A. J., Perrett, K. P., and Beverley, P. C. (2009). Maintaining protection against invasive bacteria with protein-polysaccharide conjugate vaccines. Nat. Rev. Immunol. 9, 213–220. doi: 10.1038/nri2494
Pozsgay, V., Chu, C., Pannell, L., Wolfe, J., Robbins, J. B., and Schneerson, R. (1999). Protein conjugates of synthetic saccharides elicit higher levels of serum IgG lipopolysaccharide antibodies in mice than do those of the O-specific polysaccharide from Shigella Dysenteriae type 1. Proc. Natl Acad. Sci. U.S.A. 96, 5194–5197. doi: 10.1073/pnas.96.9.5194
Prasanna, M., Soulard, D., Camberlein, E., Ruffier, N., Lambert, A., Trottein, F., et al. (2019). Semisynthetic glycoconjugate based on dual role protein/PsaA as a pneumococcal vaccine. Eur. J. Pharm. Sci. 129, 31–41. doi: 10.1016/j.ejps.2018.12.013
Quast, R. B., Mrusek, D., Hoffmeister, C., Sonnabend, A., and Kubick, S. (2015). Cotranslational incorporation of non-standard amino acids using cell-free protein synthesis. FEBS Lett. 589, 1703–1712. doi: 10.1016/j.febslet.2015.04.041
Rajam, G., Anderton, J. M., Carlone, G. M., Sampson, J. S., and Ades, E. W. (2008a). Pneumococcal surface adhesin A (PsaA): a review. Crit. Rev. Microbiol. 34, 131–142. doi: 10.1080/10408410802275352
Rajam, G., Phillips, D. J., White, E., Anderton, J., Hooper, C. W., Sampson, J. S., et al. (2008b). A functional epitope of the pneumococcal surface adhesin A activates nasopharyngeal cells and increases bacterial internalization. Microb. Pathog. 44, 186–196. doi: 10.1016/j.micpath.2007.09.003
Rappuoli, R. (2018). Glycoconjugate vaccines: principles and mechanisms. Sci. Transl. Med. 10:eaat4615. doi: 10.1126/scitranslmed.aat4615
Romero-Steiner, S., Caba, J., Rajam, G., Langley, T., Floyd, A., Johnson, S. E., et al. (2006). Adherence of recombinant pneumococcal surface adhesin A (RPsaA)-coated particles to human nasopharyngeal epithelial cells for the evaluation of anti-PsaA functional antibodies. Vaccine 24, 3224–3231. doi: 10.1016/j.vaccine.2006.01.042
Safari, D., Dekker, H. A. T., Joosten, J. A. F., Michalik, D., Carvalho de Souza, A., Adamo, R., et al. (2008). Identification of the smallest structure capable of evoking opsonophagocytic antibodies against Streptococcus pneumoniae type 14. Infect. Immun. 76, 4615–4623. doi: 10.1128/IAI.00472-08
Schmid, P., Selak, S., Keller, M., Luhan, B., Magyarics, Z., Seidel, S., et al. (2011). Th17/Th1 biased immunity to the pneumococcal proteins PcsB, StkP and PsaA in adults of different age. Vaccine 29, 3982–3989. doi: 10.1016/j.vaccine.2011.03.081
Singh, R., Gupta, P., Sharma, P. K., Ades, E. W., Hollingshead, S. K., Singh, S., et al. (2014). Prediction and characterization of helper T-cell epitopes from pneumococcal surface adhesin A. Immunology 141, 514–530. doi: 10.1111/imm.12194
Srivastava, N., Zeiler, J. L., Smithson, S. L., Carlone, G. M., Ades, E. W., Sampson, J. S., et al. (2000). Selection of an immunogenic and protective epitope of the PsaA protein of Streptococcus pneumoniae using a phage display library. Hybridoma 19, 23–31. doi: 10.1089/027245700315761
Stefanetti, G., Hu, Q.-Y., Usera, A., Robinson, Z., Allan, M., Singh, A., et al. (2015). Sugar-protein connectivity impacts on the immunogenicity of site-selective salmonella O-Antigen glycoconjugate vaccines. Angew. Chem. Int. Ed. 54, 13198–13203. doi: 10.1002/anie.201506112
van der Linden, M., Falkenhorst, G., Perniciaro, S., Fitzner, C., and Imöhl, M. (2016). Effectiveness of pneumococcal conjugate vaccines (PCV7 and PCV13) against invasive pneumococcal disease among children under two years of age in Germany. PLoS ONE 11:e0161257. doi: 10.1371/journal.pone.0161257
Wang, S., Li, Y., Shi, H., Scarpellini, G., Torres-Escobar, A., Roland, K. L., et al. (2010). Immune responses to recombinant pneumococcal PsaA antigen delivered by a live attenuated Salmonella vaccine. Infect. Immun. 78, 3258–3271. doi: 10.1128/IAI.00176-10
Whaley, M. J., Sampson, J. S., Johnson, S. E., Rajam, G., Stinson-Parks, A., Holder, P., et al. (2010). Concomitant administration of recombinant PsaA and PCV7 reduces Streptococcus pneumoniae serotype 19A colonization in a murine model. Vaccine 28, 3071–3075. doi: 10.1016/j.vaccine.2010.02.086
Yahiaoui, R. Y., Bootsma, H. J., den Heijer, C. D. J., Pluister, G. N., Paget, W. J., Spreeuwenberg, P., et al. (2018). Distribution of serotypes and patterns of antimicrobial resistance among commensal Streptococcus pneumoniae in nine European countries. BMC Infect. Dis. 18:440. doi: 10.1186/s12879-018-3341-0
Keywords: glycoconjugate vaccine, cysteine mutagenesis, chemoselective ligation, protein conjugation, pneumococcal vaccine, thio/maleimide ligation
Citation: Pillot A, Defontaine A, Fateh A, Lambert A, Prasanna M, Fanuel M, Pipelier M, Csaba N, Violo T, Camberlein E and Grandjean C (2019) Site-Specific Conjugation for Fully Controlled Glycoconjugate Vaccine Preparation. Front. Chem. 7:726. doi: 10.3389/fchem.2019.00726
Received: 28 July 2019; Accepted: 10 October 2019;
Published: 01 November 2019.
Edited by:
Christoph Rademacher, Max Planck Institute of Colloids and Interfaces, GermanyReviewed by:
Rino Rappuoli, GlaxoSmithKline, ItalyNeil Ravenscroft, University of Cape Town, South Africa
Copyright © 2019 Pillot, Defontaine, Fateh, Lambert, Prasanna, Fanuel, Pipelier, Csaba, Violo, Camberlein and Grandjean. This is an open-access article distributed under the terms of the Creative Commons Attribution License (CC BY). The use, distribution or reproduction in other forums is permitted, provided the original author(s) and the copyright owner(s) are credited and that the original publication in this journal is cited, in accordance with accepted academic practice. No use, distribution or reproduction is permitted which does not comply with these terms.
*Correspondence: Cyrille Grandjean, Y3lyaWxsZS5ncmFuZGplYW5AdW5pdi1uYW50ZXMuZnI=