- 1EntreChem SL, Vivero Ciencias de la Salud, Santo Domingo de Guzmán, Oviedo, Spain
- 2Laboratorio de Compuestos Organometálicos y Catálisis (Unidad Asociada al CSIC), Departamento de Química Orgánica e Inorgánica (IUQOEM), Centro de Innovación en Química Avanzada (ORFEO-CINQA), Universidad de Oviedo, Oviedo, Spain
- 3Institute of Chemistry, Organic & Bioorganic Chemistry, NAWI Graz, BioTechMed Graz, University of Graz, Graz, Austria
- 4Heterogeneous Biocatalysis Laboratory, CIC biomaGUNE, Donostia-San Sebastian, Spain
The self-assembly of styrene-type olefins into the corresponding stilbenes was conveniently performed in the Deep Eutectic Solvent (DES) mixture 1ChCl/2Gly under air and in the absence of hazardous organic co-solvents using a one-pot chemo-biocatalytic route. Here, an enzymatic decarboxylation of p-hydroxycinnamic acids sequentially followed by a ruthenium-catalyzed metathesis of olefins has been investigated in DES. Moreover, and to extend the design of chemoenzymatic processes in DESs, we also coupled the aforementioned enzymatic decarboxylation reaction to now concomitant Pd-catalyzed Heck-type C-C coupling to produce biaryl derivatives under environmentally friendly reaction conditions.
Introduction
Following the basis of the Sustainable Development (United Nations, 2015), and trying to confront both the current diminution of crude oil resources and the dramatic environmental difficulties connected with its use, the Chemical Community is making great efforts to employ bio-based feedstock as starting materials instead of non-renewable fossil supplies, therefore attempting to accomplish some of the essential Principles of Green Chemistry (Anastas and Warner, 1998; Matlack, 2001; Lancaster, 2002; Poliakoff et al., 2002; Sheldon et al., 2007). Nowadays, one of the major challenges of Sustainable Chemistry is replacing the use of organic solvents in chemical reactions since their production mainly relies on petrochemical manufacturing (Constable et al., 2007). In this sense, it is estimated that around 90% of the mass balance of chemical processes is associated with the solvents employed not only in the synthetic methodologies but also in the isolation and purification steps of the target products (Clark and Tavener, 2007; Jessop, 2011). Traditionally, organic chemists have employed volatile, hazardous and non-renewable petroleum-based Volatile Organic Compounds (VOCs) as reaction media taking advantage of the so-called “positive solvent effect” (Reichardt and Welton, 2010). In the search of maximizing this “positive solvent effect” while deviating from the previously commented traditional hazardous VOC solvents, chemists have endeavored to research new greener and non-conventional reaction media (Anastas, 2010), which should be easily available, biodegradable, non-toxic, biorenewable, and safe for both humans and the environment (Moity et al., 2012). In this context and during the last decade, a new family of sustainable reaction media, the so-called Deep Eutectic Solvents (DESs) (Abbott et al., 2003, 2004) has attracted the attention of many research groups worldwide. As consequence, these neoteric solvents have been broadly applied in chemistry as sustainable reaction media for: (i) polar organometallic chemistry (Mallardo et al., 2014; Vidal et al., 2014, 2016; Sassone et al., 2015; García-Álvarez et al., 2018; Rodríguez-Álvarez et al., 2018; Ghinato et al., 2019); (ii) metal catalyzed organic reactions (García-Álvarez, 2015; Vidal and García-Álvarez, 2019); (iii) biocatalysis (Gotor-Fernández and Paul, 2018); (iv) traditional organic synthesis (Liu et al., 2015; Alonso et al., 2016); (v) metal extraction and electrochemistry (Smith et al., 2014; Millia et al., 2018); and (v) polymer science (Carriazo et al., 2012; del Monte et al., 2014; Mota-Morales et al., 2018; Quirós-Montes et al., 2019; Roda et al., 2019; Sánchez-Condado et al., 2019). These sustainable eutectic solvents can be obtained just by mixing (without any further steps of purification or isolation) two molecules capable of forming a complex intermolecular network based on hydrogen-bond interactions. One molecule must be a HBA (hydrogen-bond-acceptor), whereas the other must act as hydrogen-bond-donor (HBD) (García-Álvarez, 2019). In this field, one of the most commonly used HBA for the synthesis of DESs is choline chloride (ChCl, [(CH3)3NCH2CH2OH]Cl), which is a safe and non-toxic (vitamin B4, RDA 550 mg) quaternary ammonium salt produced at multi-ton scale and employed as additive for feeding chicken (cost: 2€/Kg) (Blusztajn, 1998). In combination with different sustainable HBD [like glycerol, saccharides, urea or bio-based organic acid (i.e., lactic acid)], ChCl is able to form liquid eutectic mixtures with tunable physicochemical properties.
On the other hand, during the last decade organic chemists have tried to design cleaner and more efficient one-pot multistep processes as alternative routes to classical step-by-step processes with the concomitant: (i) minimization of the time consumption and chemical waste; (ii) simplification of the practical aspects; and (iii) possibility to cope with sensitive reaction intermediates (no isolation of transiently-formed unstable products is needed; Hayashi, 2016). However, these one-pot multistep processes typically rely on the employment of the same synthetic organic tool (metal-, bio-, or organo-catalyzed reactions) throughout the transformation, while the corresponding hybrid counterparts combining different catalytic disciplines are still very scarce. In this sense, transition-metal-catalyzed reactions (van Leeuwen, 2004; Hartwig, 2010) represent an excellent platform to produce prochiral intermediates that enzymes can subsequently convert into high-added-value enantiopure compounds (Sheldon and Pererira, 2017). This chemoenzymatic approach has been fruitfully employed in traditional and hazardous organic solvents1 due to the intrinsic shortcomings (hydrolysis, oxidations) that transition-metal complexes usually suffered in water. However, the recent advances on the field of organometallic catalysis in water (Dixneuf and Cadierno, 2013) opened the door to its possible coupling with biotransformations in aqueous systems after overcoming other concomitant drawbacks related with: (i) reciprocal poisoning of catalysts; (ii) degradation because of additives, co-factors or co-solvents; (iii) incompatibility of reaction conditions; and (iv) undesired side-reactions (Gröger and Hummel, 2014; Bornscheuer, 2015; Schmidt et al., 2018). Despite these issues, current studies are boosting the aqueous combination of well-established metal-catalyzed organic reactions (Pd, Cu, or Ru-catalyzed processes) with enzyme-mediated aminations, reductions, halogenations, or decarboxylation processes (Gröger and Hummel, 2014; Bornscheuer, 2015; Ríos-Lombardía et al., 2018; Schmidt et al., 2018). Interestingly, the combination of transition metals and enzymes in the above-mentioned DESs has been barely noticed, despite the beneficial effect of these neoteric solvents in both metal-catalyzed transformations (García-Álvarez, 2015; Vidal and García-Álvarez, 2019) and enzymatic processes (Gotor-Fernández and Paul, 2018) is now well-established. In this sense, some of us have reported the pioneering combinations in ChCl-based eutectic solvents of the ruthenium(IV)-catalyzed isomerization of allylic alcohols with bioreductions (Cicco et al., 2018); or the assemble of Pd-catalyzed Suzuki C-C coupling with bioreductions (Ketoreductases, KRED; Paris et al., 2018) and bioaminations (transaminases, ATA) (Paris et al., 2019; see Scheme 1).
Based on the excellent catalytic activity of Phenolic Acid Decarboxylase from Bacillus subtilis (BsPAD) in the decarboxylation of p-hydroxycinnamic acids in DESs (see Scheme 2; Schweiger et al., 2019), we herein present the unprecedented one-pot combination of the selective production of p-hydroxystyrenes catalyzed by a BsPAD in the eutectic mixture choline chloride/glycerol (1ChCl/2Gly) with two well-established metal-catalyzed organic processes that employ styrene-type precursors, like: (i) the Grubbs-II catalyzed metathesis of olefins (an organic reactions which remained unreported in eutectic mixtures); and (ii) the Pd-catalyzed Heck-type C-C coupling reaction2,3.
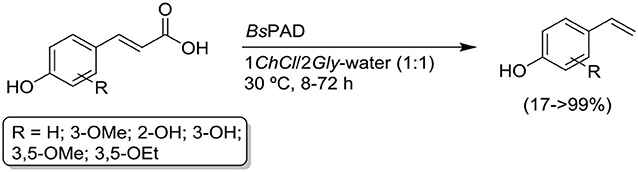
Scheme 2. BsPAD-catalyzed decarboxylation of p-hydroxycinnamic acids in the 1ChCl/2Gly-water medium.
Results and Discussion
Ruthenium Catalyzed (Grubbs-II) Metathesis of Styrene-Type Olefins in Deep Eutectic Solvents (DESs)
Ru-catalyzed olefin metathesis is considered as one of the most efficient and reliable synthetic utensils within the organic synthetic toolbox for the selective formation of new C-C bonds, thus allowing the direct access to organic compounds that would require a larger number of conventional steps for their synthesis (Grubbs, 2003; Hoveyda and Zhugralin, 2007; Grela, 2014). In this sense, and while olefin metathesis in organic and hazardous VOC solvents is nowadays ubiquitous [and even in aqueous media (Lipshutz and Ghorai, 2008; Burtscher and Grela, 2009; Tomasek and Schatz, 2013)], the possibility to carry out ruthenium-catalyzed metathesis of olefins in biorenewable eutectic mixtures has got not precedents, as far as we are aware. Accordingly, and trying to finish with this discontinuity in the employment of DESs in transition-metal-catalyzed organic transformations, our experimental work started with the study of the archetypical self-assembly metathesis of styrene (1a) as a model reaction (see Table 1) by employing the Grubbs-II complex (4 mol%) in the eutectic mixture 1ChCl/2Gly, at 50°C and in the presence of air (entry 1, Table 1). After 20 h of reaction under the aforesaid mild reaction conditions, the desired stilbene (2a) was selectively obtained in almost quantitative yield (95%). For comparison, water (the prototypical example of green solvent) was studied under the same reaction conditions (4 mol% of Grubbs-II at 50°C), giving rise to lower yields of 2a (87%, entry 2, Table 1) after longer reaction times (24 h), thus disclosing a new example of an accelerated organic reaction in DESs (García-Álvarez, 2015; Vidal and García-Álvarez, 2019). A similar reduction of the yield of the target 2a was also observed when the catalyst loading was reduced by half (i.e., 2 mol%, entry 3, Table 1). After this first parametric study, we extended our investigations to other ChCl-based DES containing different hydrogen-bond-donors [HBD, urea (entry 4) or lactic acid (Lac, entry 5)], finding in both cases a similar decrease of the catalytic activity of the Grubbs-II catalyst (60–61%). Taking into consideration the experimental fact that uncovers 1ChCl/2Gly as the best reaction media, we investigated if the presence of the quaternary ammonium salt ChCl was necessary to achieve high conversions on the final stilbene (2a). Thus, the self-assembly metathesis of 1a upon the optimized reaction conditions (50°C, 4 mol% of Grubbs-II and under air) was studied but employing pure glycerol as solvent (entry 6, Table 1). As expected from our previous experience on metal-catalyzed processes in ChCl-based DESs (García-Álvarez, 2015; Vidal and García-Álvarez, 2019), the reaction in the absence of ChCl (pure glycerol) also produced 2a but in lower yield (71%) after longer reaction time (24 h), thus revealing the positive effect of non-molecular ChCl-based DESs when compared with their separated components.
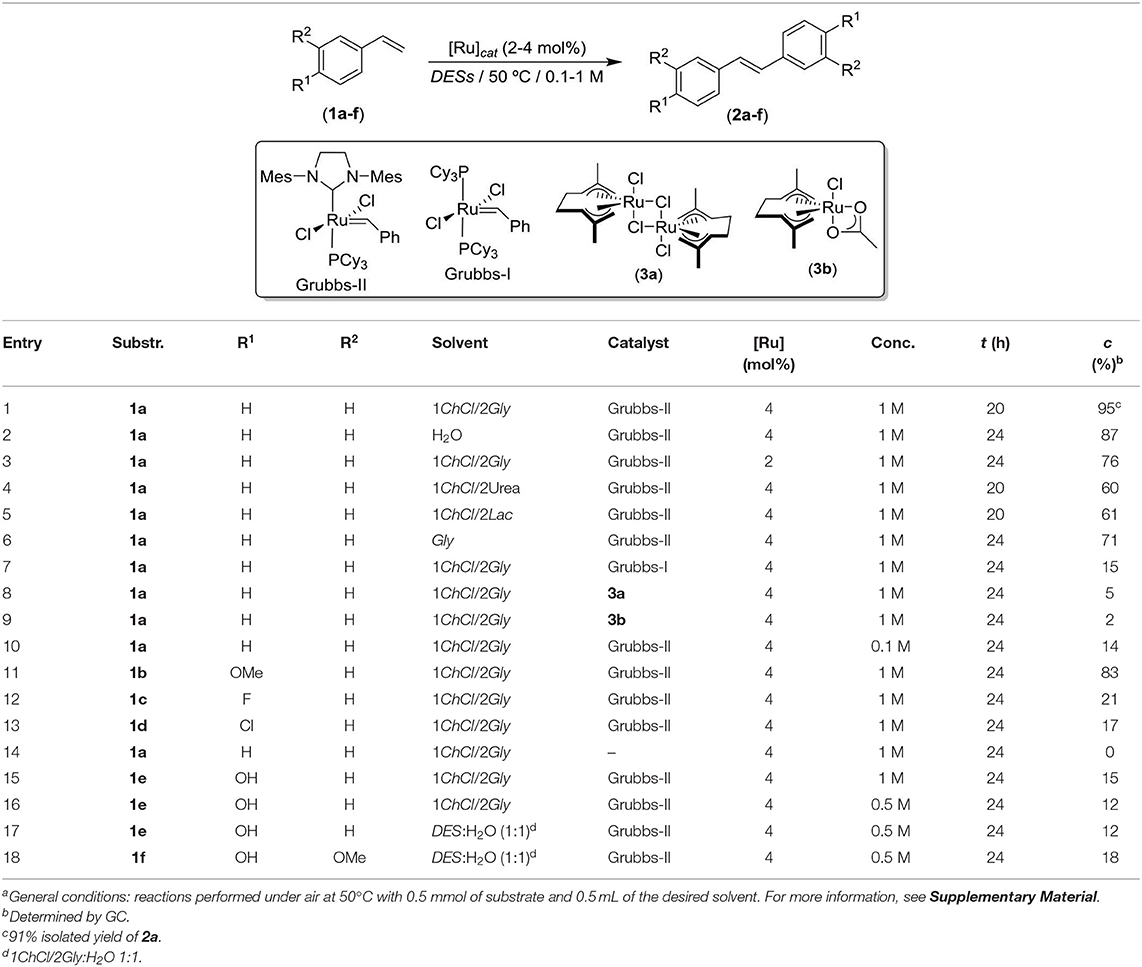
Table 1. Parameterization studies on the Ru-catalyzed self-assembly metathesis of styrenes (1a–f) in different Deep Eutectic Solvents (DESs)a.
Next, we focus our attention on the activity of other ruthenium catalysts for the self-assembly metathesis of 1a in 1ChCl/2Gly (entries 7–9). The Grubbs-I complex was tested as catalyst, discovering a dramatic decrease in the catalytic activity under the optimized reaction conditions as only 15% of 2a was obtained after 24 h (entry 7, Table 1). Taking into account the high activity exhibited for the bis(allyl)-Ru(IV) complexes 3a,b in the isomerization of allylic alcohols in DESs, these complexes were also tested in the metathesis of 1a in the eutectic mixture 1ChCl/2Gly. Unfortunately, both complexes were almost inactive in such transformation (entries 8–9, Table 1). For completeness, the parameterization study included the effect of the substrate concentration in the course of the catalytic reaction. As a result, a decrease from 1 M of 1a (entry 1) to 0.1 M (entry 10) produced a remarkable decrease in the final conversion (14%).
With the optimized reaction conditions in hand, the scope of the reaction was extended to other styrene-like olefins finding that electron donating groups (MeO, entry 11) are tolerated in the process (83%) while the presence of electron withdrawing groups (F, entry 12; Cl, entry 13) results in concomitant decrease of the activity of the Grubbs-II catalyst. Likewise, the presence of the Ru catalyst was verified as mandatory for the reaction to take place (entry 14, Table 1). Bearing in mind the planned cascade and the fact that BsPAD decarboxylates exclusively p-hydroxycinnamic acids4, p-hydroxystyrene and 4-hydroxy-3-methoxystyrene (1e–f, the tentative products resulting from the biotransformation), were subjected to the metathesis reaction. Unfortunately, the p-hydroxyl group within 1e exerted a strong negative effect and the measured conversion was exclusively of 15% (entry 15). Further attempts at 0.5 M and using a medium consisting of DES:H2O 1:1 led to similar outcome (entries 16–17). Analogously, the disubstituted 1f styrene led to the stilbene-type derivative 2f in 18%. At this point, and bearing in mind the results obtained in entries 11–18 (Table 1), it is important to highlight that we observed experimentally a direct relationship between the solubility of the styrenes 1a–f in the eutectic mixture 1ChCl/2Gly and the reaction yield. In this sense, and for the case of styrene (1a) and p-methoxy-styrene (1b), which are totally insoluble in the eutectic mixture 1ChCl/2Gly, the catalytic reaction takes place under heterogeneous conditions and best reaction yields were observed (95 and 83%, respectively). However, we observed a dramatic decrease in the yield of the reaction (12–21%) when partially soluble (1c–d) or soluble (1e–f) styrenes were employed5, 6. Similarly, some of us have previously reported an analogous scenario when using organolithium reagents (RLi) in the heterogeneous anionic polymerization of the aforementioned styrenes 1a-d when employing polar eutectic mixtures as reaction media (Sánchez-Condado et al., 2019).
As stated above, the inhibition of the catalytic activity of transition-metal complexes in the presence of enzymes, co-factors or buffers is a well-known phenomenon in one-pot chemoenzymatic processes (Gröger and Hummel, 2014; Bornscheuer, 2015; Schmidt et al., 2018). Accordingly, we designed two experiments aimed at verifying the compatibility of the co-factor free BsPAD-catalyzed decarboxylation process with the Grubbs-II catalyzed self-assembly metathesis of styrenes in DESs7. Initially, the catalytic activity of the Grubbs-II catalyst in the metathesis of 1a was evaluated in the medium employed in the enzymatic decarboxylation, namely a mixture 1ChCl/2Gly:H2O 1:1 (see Scheme 2). Similarly, in a second experiment the enzyme was also added to the reaction media 1ChCl/2Gly:H2O containing the Grubbs-II catalyst. Pleasantly, both tests showed that the metallic complex suffers virtually no erosion of its catalytic activity (96% of 2a) in the reaction conditions needed for the starting enzymatic step of the planned cascade process.
Chemoenzymatic Combination of BsPAD-Catalyzed Decarboxylation of p-Hydroxycinnamic Acids With the Ru-Catalyzed (Grubbs-II) Metathesis of Styrene-Type Olefins in DESs
Encouraged by the previous parametrization that suggest the possibility to trigger the self-assembly metathesis of p-hydroxystyrenes employing directly the reaction media coming from the enzymatic decarboxylation of p-hydroxycinnamic acids, we designed the chemoenzymatic coupled process in a 1ChCl/2Gly-water medium (see Scheme 3). According to conditions optimized previously (Schweiger et al., 2019), the BsPAD-catalyzed decarboxylation of 3f was performed at 300 mM substrate concentration and 30°C in 1ChCl/2Gly-water. Once the biotransformation was completed, the Grubbs-II catalyst was then added and the reaction mixture stirred for 24 h at 50°C. As expected, the conversion in the metathesis step was very low and the isolated overall yield of 2f for the two-steps process resulted in 15%. Hence, our studies revealed that the stepwise enzymatic decarboxylation/metal-catalyzed metathesis was hampered by the intrinsic limitations of the metallic step, not by the incompatibility between single reactions. At least, it can be taken as a proof of concept of the viability of this chemoenzymatic process in DES-water media at the expense of developing more efficient catalysts for the metathesis of p-hydroxystyrenes.

Scheme 3. One-pot sequential enzymatic decarboxylation (BsPAD)/metal-catalyzed metathesis (Grubbs-II) of p-hydroxycinnamic acid (3f) in the mixture 1ChCl/2Gly-water.
Chemoenzymatic Combination of the BsPAD-Catalyzed Decarboxylation of p-Hydroxycinnamic Acids With the Pd-Catalyzed Heck Coupling in DESs
Seeing as the above unsatisfactory results, we turned our attention on other metal-catalyzed transformations reported in eutectic mixtures, like the case of the Pd-catalyzed Heck-type C-C coupling2, 3. In fact, such chemoenzymatic process, namely the tandem enzymatic decarboxylation/Heck coupling has just been reported in continuous flow in DES-water to tackle incompatibility of catalysts and solubility issues (Grabner et al., 2020). Accordingly, we planned to get more insight about this system by setting up the counterpart process in batch conditions (Scheme 4). Following the reported conditions in the flow system, we essayed the Heck coupling between p-hydroxystyrene (1e) and iodobenzene (4) in 1ChCl/2Gly:H2O 1:1 but employing the commercially available catalyst Pd(PPh3)4. The use of EtOH as co-solvent (25% v/v) proved to be critical for the outcome of the reaction. Working at 100°C and 60 mM of 4, the conversion reached a value >90% with an isolated yield of 65% on (E)-4-hydroxystilbene (5e) after 8 h. In fact, due to the strong reaction conditions, the yield was limited by the formation of the isomeric byproduct p-hydroxy-1,1-diphenylethylene at a percentage close to 10%. On the other hand, the inhibition studies unveiled that by adding exogenous BsPAD the Pd catalyst is deactivated (c = 0%) which precludes the chemoenzymatic process without additional settings.

Scheme 4. One-pot sequential enzymatic decarboxylation (BsPAD)/Pd(PPh3)4-catalyzed Heck coupling of p-hydroxycinnamic acid (3e) with PhI (4) in DES-water mixtures.
In a first attempt to remove the biocatalyst for the tentative second step of the cascade, a BsPAD-catalyzed decarboxylation of 3e was conducted at 120 mM and 30°C in 1ChCl/2Gly:H2O 1:1 during 2 h. Once the biotransformation was accomplished (99%), the reaction mixture was subjected to centrifugation at 13,000 rpm during 5 min. The insolubles were discarded and the supernatant diluted (up to 60 mM of PhI, the limiting reactant) and treated with the reagents for the Heck coupling. As a result, the overall yield for the two-steps process was exclusively of 15%, which suggests that BsPAD partially remained in the supernatant. Hence, two strategies were planned to avoid the contact between catalysts: (i) immobilization of BsPAD on a solid support; or (ii) employment of aqueous micellar solutions which enable to isolate metal catalyst and enzyme from each other. For more information, see Supplementary Material.
With regards to the first approach, we conceived a solid support for BsPAD which fitted the requirements for the chemoenzymatic cascade; preserving the catalytic activity of the enzyme and avoiding the enzyme lixiviation to the reaction medium based on DES-water mixtures. With these premises, we selected the commercially available carrier EP-403S (pore size: 40–60 nm and particle size: 100–300 μm). We further activated such carrier with tertiary amine groups (EP-TEA) to promote the enzyme immobilization through reversible but strong ionic interactions as reported for other industrially relevant enzymes as ketoreductases (Benitez-Mateos et al., 2017). 100% of the offered BsPAD was immobilized on the carrier although the specific activity of the enzyme was reduced to 51% upon the immobilization (Table 2). Noteworthy, the immobilized BsPAD was significantly more active than other decarboxylases immobilized on pre-existing carriers through both reversible and irreversible chemistries (Aßmann et al., 2017). When we performed fluorescence studies, we observed that the intrinsic Trp-fluorescence intensity of BsPAD decreased and its maximum emission wavelength was red-shifted when the enzyme was immobilized (Figure 1A). That fluorescence decay suggests that the immobilization induced some conformational distortions on BsPAD structure that would explain its lower recovered enzyme. Inspecting the X-ray structure of BsPAD, we found a region clearly enriched in Asp and Glu, which makes us to postulate that region as the one through the immobilization takes place. Moreover, in that acidic region, we find two main tyrosines that may be quenched to some extent upon the immobilization (Figures 1A,B). Hence, we suggest that the lower catalytic efficiency of this heterogeneous biocatalyst may rely on some structural distortion at the interface between that acidic region of the enzyme and the surface of acrylic porous beads.
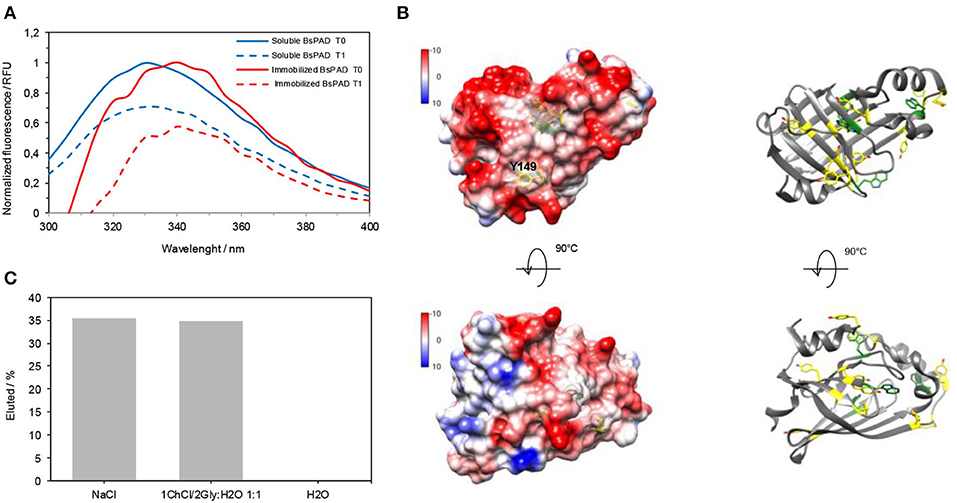
Figure 1. (A) Total intrinsic fluorescence of soluble and immobilized BsPAD using an excitation wavelength of 280 nm. T0 (solid lines) correspond to spectra of the non-incubated enzymes, while T1 (dashed lines) do to enzyme incubated for 1 h at 45°C. Data were normalized assigning a value of 1 to the highest fluorescence intensity value for each sample non-thermally incubated (sample T0s). (B) Electrostatic surface representation of BsPAD (PDB ID: 2P8G). Tryptophan and tyrosine residues are colored in green and yellow, respectively. Images were made with Chimera software. (C) Percentage of protein eluted from the carrier after an incubation of 1 h with 1 M NaCl, a solution of (1ChCl/2Gly:H2O 1:1) and H2O.
We named the resulting heterogeneous biocatalysts as PAD@EC-TEA, which despite presenting lower specific activity than the soluble enzyme, exhibited an excellent decarboxylation efficiency toward 3e both in water and 1ChCl/2Gly:H2O 1:1. In particular, working at 200 mM of 3e and a comparable loading of biocatalyst, the reaction was complete in 2 h (c > 99%). Remarkably, PAD@EC-TEA could be efficiently recycled up to four times in H2O meanwhile the heterogeneous biocatalyst completely lost its activity after just one cycle in the DES mixture. This fact was due to the high concentration of choline needed to form the DES (3 M) that partially released BsPAD from the aminated carrier (Figure 1C). In fact, the DES was as efficient as 1 M NaCl breaking the electrostatic interactions between the proteins and the positively charged carrier, and therefore eluting BsPAD to the reaction crude. Then, two decarboxylation reactions of 3e were run at 120 mM in 1ChCl/2Gly:H2O 1:1 and water. After verifying complete conversion of the cinnamate by HPLC, the reaction mixtures were filtered off to remove PAD@EC-TEA and subjected to further Heck coupling (60 mM). Using the selected DES mixture, no product was detected as expected since choline partially eluted the BsPAD to the reaction crude (Figure 1C), thus that soluble enzyme inhibited the Pd catalyst, precluding the formation of the C-C bond (Table 3, entry 2). On the contrary, running the decarboxylation in water, the enzyme remained bound to the carrier and consequently the metal-catalyzed reaction rendered 5e in 60% overall yield (Table 3, entry 3). Therefore, this two-step one-pot sequential chemoenzymatic process successfully worked up when firstly performing the BsPAD-driven decarboxylation in water, separation the heterogeneous biocatalysts by simple vacuum filtration and finally diluting the reaction by 2-fold with the DES and adding the Pd-catalyst. Using this synthetic sequence, we are able to achieve high yields of the desired product and recycle the immobilized BsPAD.
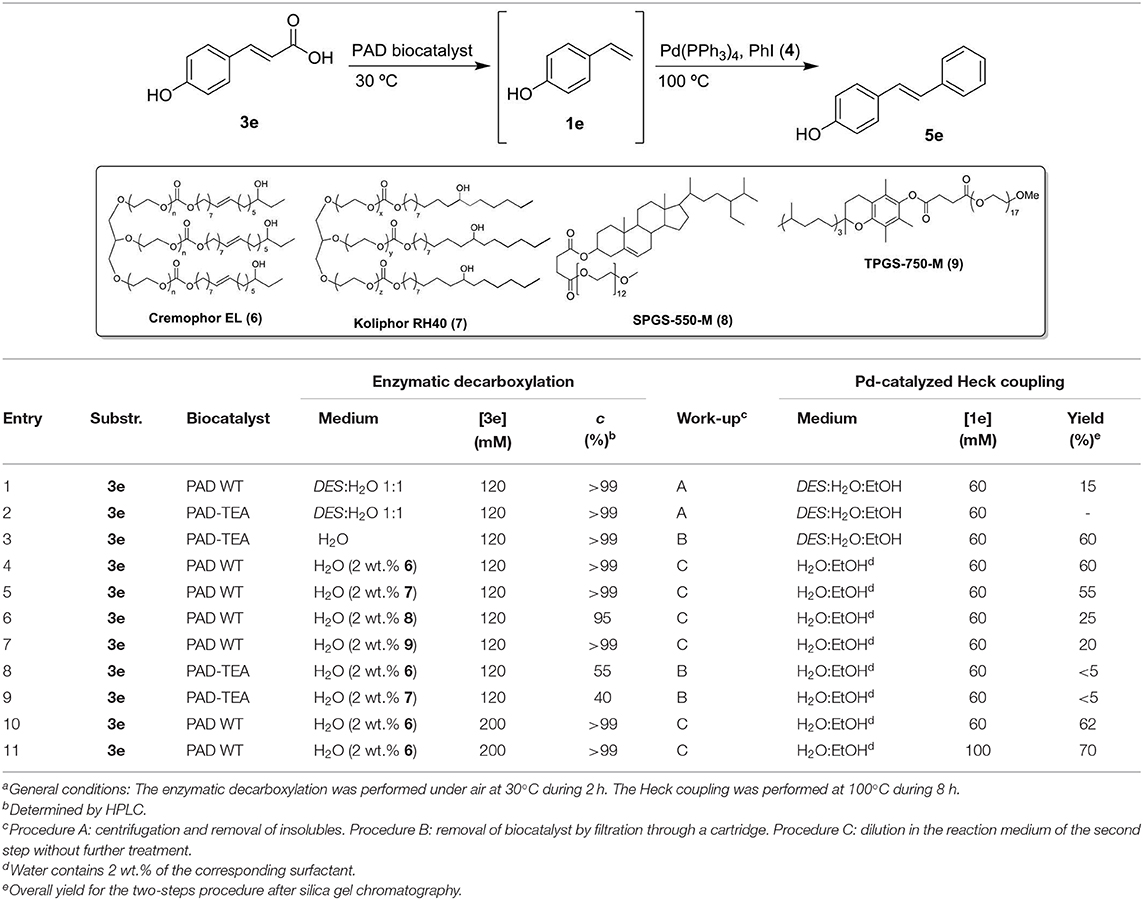
Table 3. Strategies for the one-pot sequential enzymatic decarboxylation/Pd-catalyzed Heck coupling of p-hydroxycinnamic acid (3e) in non-conventional mediaa.
Very recently, designer surfactants based on aqueous micellar solutions have been demonstrated as valuable reaction media for chemoenzymatic cascades (Cortes-Clerget et al., 2019). In particular, Lipshutz et al. developed several one-pot two-step combinations of metal-catalyzed reactions with a subsequent bioreduction. In the so-called micellar catalysis, the biocatalyst remains in the aqueous solution, while the organic solvents host the metal-catalyst and acts as reservoir for substrate and product. On the other hand, the solubilizing properties of the surfactant Cremophor® enabled to enhance the substrate concentration of poorly-water soluble substrates in a two-enzyme cascade reaction (Correia Cordeiro et al., 2019). With these precedents, the BsPAD-catalyzed decarboxylation of 3e was essayed at 200 mM in a medium consisting of water and 2 wt.% of different surfactants (above their reported critical micelle concentration)8. As deduced from Table 3, the reaction proceeded smoothly in such a low percentage of the four solubilizers tested (c > 90% entries 4–7). Then, the corresponding reaction mixtures were supplemented with an aqueous solution (2 wt.% solubilizer) containing the Pd catalyst, EtOH, PhI, and K2CO3 and heated at 100°C during 8 h. The media containing Cremophor EL (6) and Kolliphor RH40 (7) led to good yields (55–60%, entries 4–5), in contrast to those containing both SPGS-550-M (8) and TPGS-750-M (9) (20–25%, entries 6–7)9. Likewise, the immobilized biocatalyst (BsPAD-TEA) was also tested in the best micellar solutions (those containing 6 and 7). Unfortunately, such media resulted in incomplete decarboxylation of 3e (c < 55%, entries 8–9) which led to very low overall yields after the metal-catalyzed step (c < 5%). Finally, the effect of substrate concentration on the two-steps protocol was studied. The enzymatic decarboxylation of 3e took place warmly at 200 mM in the micellar solution containing 6 (entry 10). Further dilution to 60 mM in PhI for the Heck coupling (as in previous experiments) led to comparable isolated yield (62%). Gratefully, the attempt to enhance the second step to 100 mM resulted in an improved overall yield (70%, entry 11).
Conclusions
The application of non-conventional media such as Deep Eutectic Solvents (DESs) or aqueous micellar solutions as a practical solution to set up two chemoenzymatic cascades has been studied. In particular, the BsPAD-catalyzed decarboxylation of p-hydroxycinnamic acids was coupled alternatively with two metal-catalyzed processes such as the metathesis of olefins and a Heck C-C coupling reaction. In the first process, both catalysts were compatible and the low overall yield was due to the ineffective activity of the Grubbs-II catalyst toward p-hydroxystyrenes in such eutectic mixtures. With regards to the second, the employment of an immobilized biocatalyst on the one hand or micellar solutions on the other was enough to confine the enzyme and avoid its inhibitory activity on the Pd catalyst, the target biaryl derivatives being obtained in moderate to good yields. Despite some limitations remained challenging in the processes reported herein, these examples contribute to expand the available toolbox for developing chemoenzymatic cascades. Future efforts must be led to improve the immobilization of BsPAD on solid materials to achieve irreversibly bound enzymes that do not interfere with the metal-catalyzed step and tolerate DES mixtures to assemble one-pot concurrent systems.
Data Availability Statement
The raw data supporting the conclusions of this article will be made available by the authors, without undue reservation, to any qualified researcher.
Author Contributions
NR-L and MR-Á carried out chemical synthesis and characterization of the products. FM contributed to the manuscript revision and writing. RK developed and supplied the enzyme Bacillus subtilis (BsPAD). NC and FL-G have designed and characterized the supported enzyme (BsPAD-TEA). JG-S and JG-Á designed and managed the study and also carried out manuscript writing. All the authors approved the publication of the work.
Funding
JG-Á thanks: the Fundación BBVA for the award of a Beca Leonardo a Investigadores y Creadores Culturales 201710; PhosAgro/UNESCO/IUPAC for the award of a Green Chemistry for Life Grant; and Spanish MINECO (Project CTQ2016-81797-REDC and CTQ2016-75986-P). FL-G and NC thank to IKERBASQUE Basque country for science.
Conflict of Interest
NR-L, FM, and JG-S were employed by the company Entrechem S.L.
The remaining authors declare that the research was conducted in the absence of any commercial or financial relationships that could be construed as a potential conflict of interest.
Supplementary Material
The Supplementary Material for this article can be found online at: https://www.frontiersin.org/articles/10.3389/fchem.2020.00139/full#supplementary-material
Footnotes
1. ^For selected recent examples, see: (Lohr and Marks, 2015; Verho and Bäckvall, 2015; El-Sepelgy et al., 2016; Palo-Nieto et al., 2016; Yang et al., 2016; Görbe et al., 2017).
2. ^For previous examples that described palladium-catalyzed Heck-type C-C coupling processes in DESs, see: (Illgen and König, 2009; Marset et al., 2017; Saavedra et al., 2019).
3. ^At this point, we would like to highlight that Ramón et al. have nicely studied (by DFT calculations and NMR techniques) the possible catalytically active Pd-species present in the Heck reaction in eutectic mixtures (see Marset et al., 2017).
4. ^The mechanism of the BsPAD-catalyzed decarboxylation of p-hydroxycinnamic acids starts by deprotonation of the p-hydroxy group and proceeds via a quinone methide intermediate, which then leads to the cleavage of CO2. For the specific case of Deep Eutectic Solvents, it has been reported that: “in view of the observed differing substrate preference dependent on the applied solvent, it is suggested that solvent effects play a crucial role in our studied reaction systems besides classical steric and electronic properties of the substrates.” For more information, see: (Rodríguez et al., 2010).
5. ^These experimental facts suggest that the catalytic reaction takes place “on the eutectic mixture” and not “in the eutectic mixture”. This “on DES” concept is closely related with the “on water” concept previously coined by K. B. Sharpless. These “on water” (or “on DES”) reactions are thought to occur at the organic/liquid water (or eutectic mixture) interface with water (or DES) insoluble reactants. For leading references, dealing with the “on water” concept, see: (Narayan et al., 2005; Chanda and Fokin, 2009; Butler and Coyne, 2015, 2016).
6. ^This mechanistic proposal indicates that the eutectic-phobic interactions in between both the insoluble styrenes and the catalyst permit an intimal contact between both reactants, thus increasing the overall observed catalytic rate of the process. This mechanistic effect is now well-established and has been previously observed by different research groups worldwide in the study of Grubbs-catalyzed “on water” metathesis processes. For outstanding articles and book chapters that reported previous examples of this mechanistic effect in Grubbs-catalyzed metathesis reactions see: (Gułajski et al., 2008; Diallo et al., 2010; Grela et al., 2015).
7. ^For previous examples dealing with the combination of Ru-catalyzed metathesis processes and different enzymatic transformations in aqueous media, see: (Tenbrink et al., 2011; Denard et al., 2014; Gómez Baraibar et al., 2016; Scalacci et al., 2017; Bojarra et al., 2018).
8. ^The technical specifications for the commercially available Kolliphor RH40® and Cremophor EL® report a critical micelle concentration (CMC) of 0.03% w/w at 37°C and 0.02% w/w at 25°C, respectively. With regards to the recently designed surfactants TPGS-750-M and SPGS-550-M, they exhibited a CMC of ca. 10−4 M and 2% w/w was found to be useful for most reactions studied to date: (Lipshutz and Ghorai, 2012).
9. ^The observed decrease in the catalytic activity when using TPG-750-M and SPGS-550M could be explained by bearing in mind the fact that their technical specifications assessed that: reactions can be run between 4 and 60°C. Above 70°C, however, particle shapes become unpredictable; e.g., reorganization from spherical particles to bilayer arrays may occur, and so the quality of reactions run in these modified particles is not predictable.
10. ^The Fundación BBVA accepts no responsibility for the opinions, statements, and contents included in the Project and/or the results thereof, which are entirely the responsibility of the authors.
References
Abbott, P., Boothby, D., Capper, G., Davies, D., and Rasheed, R. K. (2004). Deep eutectic solvents formed between choline chloride and carboxylic acids: versatile alternatives to ionic liquids. J. Am. Chem. Soc. 126, 9142–9147. doi: 10.1021/ja048266j
Abbott, P., Capper, G., Davies, D. L., Rasheed, R. K., and Tambyrajah, V. (2003). Novel solvent properties of choline chloride/urea mixtures. Chem. Commun. 70–71. doi: 10.1039/b210714g
Alonso, D. A., Baeza, A., Chinchilla, R., Guillena, G., Pastor, I. M., and Ramón, D. J. (2016). Deep eutectic solvents: the organic reaction medium of the century. Eur. J. Org. Chem. 2016, 612–632. doi: 10.1002/ejoc.201501197
Anastas, P. T. (2010). Handbook of Green Chemistry, Vol. 4, 5 and 6, Green Solvents: Volume 4: Supercritical Solvents (eds. Leitner, W., P. G. Jessop); Volume 5: Reactions in Water (ed. C.-J. Li); Volume 6: Ionic Liquids (eds. Wasserschied, P., A. Stark), Wiley-VCH, Weinheim. doi: 10.1002/9783527628698
Anastas, P. T., and Warner, J. C. (1998). Green Chemistry Theory and Practice. Oxford: Oxford University Press.
Aßmann, M., Mügge, C., Gaßmeyer, S. K., Enoki, J., Hilterhaus, L., Kourist, R., et al. (2017). Improvement of the process stability of arylmalonate decarboxylase by immobilization for biocatalytic profen synthesis. Front. Microbiol. 8:448. doi: 10.3389/fmicb.2017.00448
Benitez-Mateos, San Sebastian, E., Ríos-Lombardía, N., Morís, F., González-Sabín, J., and López-Gallego, F. (2017). Asymmetric reduction of prochiral ketones by using self-sufficient heterogeneous biocatalysts based on NADPH-dependent ketoreductases. Chem. Eur. J. 23, 16843–16852. doi: 10.1002/chem.201703475
Blusztajn, J. K. (1998). Choline, a vital amine. Science 284, 794–795. doi: 10.1126/science.281.5378.794
Bojarra, S., Reichert, D., Grote, M., Gómez Baraibar, A., Dennig, A., Nidetzky, B., et al. (2018). Bio-based α,ω-functionalized hydrocarbons from multi-step reaction sequences with bio- and metallo-catalysts based on the fatty acid decarboxylase OleTJE. ChemCatChem. 10, 1192–201. doi: 10.1002/cctc.201701804
Bornscheuer, U. T. (2015). Biocatalysis: successfully crossing boundaries. Angew. Chem. Int. Ed. 55, 4372–4373. doi: 10.1002/anie.201510042
Burtscher, D., and Grela, K. (2009). Aqueous olefin metathesis. Angew. Chem. Int. Ed. 48, 442–454. doi: 10.1002/anie.200801451
Butler, R. M., and Coyne, A. G. (2015). Understanding “on-water” catalysis of organic reactions. Effects of H+ and Li+ ions in the aqueous phase and nonreacting competitor h-bond acceptors in the organic phase: on H2O versus on D2O for huisgen cycloadditions. J. Org. Chem. 80, 1809–1817. doi: 10.1021/jo502732y
Butler, R. N., and Coyne, A. G. (2016). Organic synthesis reactions on-water at the organic-liquid water interface. Org. Biomol. Chem. 14, 9945–9960. doi: 10.1039/C6OB01724J
Carriazo, D., Serrano, M. C., Gutiérrez, M. C., Ferrer, M. L., and Del Monte, F. (2012). Deep-eutectic solvents playing multiple roles in the synthesis of polymers and related materials. Chem. Soc. Rev. 41, 4996–5014. doi: 10.1039/c2cs15353j
Chanda, A., and Fokin, V. V. (2009). Organic synthesis “on water”. Chem. Rev. 109, 725–748. doi: 10.1021/cr800448q
Cicco, L., Ríos-Lombardía, N., Rodríguez-Álvarez, M. J., Morís, F., Perna, F. M., Capriati, V., et al. (2018). Programming cascade reactions interfacing biocatalysis with transition-metal catalysis in Deep Eutectic Solvents as biorenewable reaction media. Green Chem. 20, 3468–3475. doi: 10.1039/C8GC00861B
Clark, J. H., and Tavener, S. J. (2007). Alternative solvents: shades of green. Org. Process Res. Dev. 11, 149–155. doi: 10.1021/op060160g
Constable, D. J. C., Jiménez-González, C., and Henderson, R. K. (2007). Perspective on solvent use in the pharmaceutical industry. Org. Process Res. Dev. 11, 133–137. doi: 10.1021/op060170h
Correia Cordeiro, R. S., Ríos-Lombardía, N., Morís, F., Kourist, R., and González-Sabín, J. (2019). One-pot transformation of ketoximes into optically active alcohols and amines by sequential action of laccases and ketoreductases or ω-transaminases. ChemCatChem. 11, 1272–1277. doi: 10.1002/cctc.201801900
Cortes-Clerget, M., Akporji, N., Zhou, J., Gao, F., Guo, P., Parmentier, M., et al. (2019). Bridging the gap between transition metal- and bio-catalysis via aqueous micellar catalysis. Nat. Commun. 10:2169. doi: 10.1038/s41467-019-09751-4
del Monte, F., Carriazo, D., Serrano, M. C., Gutiérrez, M. C., and Ferrer, M. L. (2014). Deep eutectic solvents in polymerizations: a greener alternative to conventional syntheses. ChemSusChem. 7, 999–1009. doi: 10.1002/cssc.201300864
Denard, C. A., Huang, H., Bartlett, M. J., Lu, L., Tan, Y., Zhao, H., et al. (2014). Cooperative tandem catalysis by an organometallic complex and, a metalloenzyme. Angew. Chem. Int. Ed. 53, 465–469. doi: 10.1002/anie.201305778
Diallo, K., Boisselier, E., Liang, L., Ruiz, J., and Astruc, D. (2010). Dendrimer-induced molecular catalysis in water: the example of olefin metathesis. Chem. Eur. J. 16, 11832–11835. doi: 10.1002/chem.201002014
Dixneuf, P. H., and Cadierno, V. (eds.). (2013). Metal-Catalyzed Reactions in Water. Weinheim: Wiley-VCH. doi: 10.1002/9783527656790
El-Sepelgy, O., Alandini, N., and Rueping, M. (2016). Merging iron catalysis and biocatalysis-iron carbonyl complexes as efficient hydrogen autotransfer catalysts in dynamic kinetic resolutions. Angew. Chem. Int. Ed. 55, 13602–13605. doi: 10.1002/anie.201606197
García-Álvarez, J. (2015). Deep eutectic mixtures: promising sustainable solvents for metal-catalysed and metal-mediated organic reactions. Eur. J. Inorg. Chem. 2015, 5147–5157. doi: 10.1002/ejic.201500892
García-Álvarez, J. (2019). “Deep eutectic solvents and their applications as new green and biorenewable reaction media,” in Handbook of Solvents, Vol. 2, 3rd Edn.: Use, Health, and Environment, ed G. Wypych (Toronto: ChemTec Publishing).
García-Álvarez, J., Hevia, E., and Capriati, V. (2018). The future of polar organometallic chemistry written in bio-based solvents and water. Chem. Eur. J. 24, 14854–14863. doi: 10.1002/chem.201802873
Ghinato, S., Dialuro, G., Perna, F. M., Capriati, V., Blangetti, M., and Prandi, C. (2019). Directed ortho-metalation–nucleophilic acyl substitution strategies in deep eutectic solvents: the organolithium base dictates the chemoselectivity. Chem. Commun. 55, 7741–7744. doi: 10.1039/C9CC03927A
Gómez Baraibar, A., Reichert, D., Mügge, C., Seger, S., Gröger, H., and Kourist, R. A. (2016). A one-pot cascade reaction combining an encapsulated decarboxylase with, a. metathesis. catalyst for the synthesis of bio-based antioxidants. Angew. Chem. Int. Ed. 55, 14823–14827. doi: 10.1002/anie.201607777
Görbe, T., Gustafson, KP J, Verho, O., Kervefors, G., Zheng, H., Zou, X., et al. (2017). Design of a Pd.(0)-CalB CLEA biohybrid catalyst and its application in a one-pot cascade reaction. ACS Catal. 7, 1601–1605. doi: 10.1021/acscatal.6b03481
Gotor-Fernández, V., and Paul, C. E. (2018). “Biotransformations in deep eutectic solvents,” in Sustainable Catalysis in Ionic Liquids, ed P. Lozano (Boca Raton: CRC Press), 137–172. doi: 10.1201/9781315148694-7
Grabner, B., Schweiger, A. K., Gavric, K., Kourist, R., and Gruber-Woelfler, H. (2020). A chemo-enzymatic tandem reaction in a mixture of deep eutectic solvent and water in continuous flow. React. Chem. Eng. 5, 263–269. doi: 10.1039/C9RE00467J
Grela, K. (2014). Olefin Metathesis: Theory and Practice. Hoboken: John Wiley & Sons, Inc. doi: 10.1002/9781118711613
Grela, K., Gułajski, Ł., and Skoerski, K. (2015). “Alkene metathesis in water,” in Water, Metal-Catalyzed Reactions in Water, eds P. H. Dixneuf and V. Cadierno (Weinheim: Wiley-VCH), 291–336.
Gröger, H., and Hummel, W. (2014). Combining the 'two worlds' of chemocatalysis and biocatalysis towards multi-step one-pot processes in aqueous media. Curr. Opin. Chem. Biol. 19, 171–179. doi: 10.1016/j.cbpa.2014.03.002
Grubbs, R. H. (2003). Handbook of Metathesis. Weinheim: Wiley-VCH Verlag GmbH & Co; KGaA. doi: 10.1002/9783527619481
Gułajski, Ł., Sledz, P., Lupa, A., and Grela, K. (2008). Olefin metathesis in water using acoustic emulsification. Green Chem. 10, 271–274. doi: 10.1039/b719493e
Hartwig, J. F. (2010). Organotransition Metal Chemistry: From Bonding to Catalysis. Sausalito: University Science Books.
Hayashi, Y. (2016). Pot economy and one-pot synthesis. Chem. Sci. 7, 866–880. doi: 10.1039/C5SC02913A
Hoveyda, A. H., and Zhugralin, A. R. (2007). The remarkable metal-catalysed olefin metathesis reaction. Nature. 450, 243–251. doi: 10.1038/nature06351
Illgen, F., and König, B. (2009). Organic reactions in low melting mixtures based on carbohydrates and L-carnitine-a comparison. Green Chem. 11, 848–854. doi: 10.1039/b816551c
Jessop, P. G. (2011). Searching for green solvents. Green Chem. 13, 1391–1398. doi: 10.1039/c0gc00797h
Lipshutz, B. H., and Ghorai, S. (2008). Transition-metal-catalyzed cross-couplings going green: in water at room temperature. Aldrichim. Acta. 41, 59–72. doi: 10.1002/chin.200941255
Lipshutz, B. H., and Ghorai, S. (2012). “Designer”-surfactant-enabled cross-couplings in water at room temperature. Aldrichim. Acta. 45, 3–16. doi: 10.1002/chin.201238257
Liu, P., Hao, J., Mo, L., and Zhang, Z. (2015). Recent advances in the application of deep eutectic solvents as sustainable media as well as catalysts in organic reactions. RSC Adv. 5, 48675–48704. doi: 10.1039/C5RA05746A
Lohr, T. L., and Marks, T. (2015). Orthogonol tandem catalysis. Nat. Chem. 7, 477–482. doi: 10.1038/nchem.2262
Mallardo, V., Rizzi, R., Sassone, F. C., Mansueto, R., Perna, F. M., et al. (2014). Regioselective desymmetrization of diaryltetrahydrofurans via directed ortho-lithiation: an unexpected help from green chemistry. Chem. Commun. 50, 8655–8658. doi: 10.1039/C4CC03149K
Marset, X., Khoshnood, A., Sotorríos, L., Gómez-Bengoa, E., Alonso, D. A., and Ramón, D. J. (2017). Deep eutectic solvent compatible metallic catalysts: cationic pyridiniophosphine ligands in palladium catalyzed cross-coupling reactions. ChemCatChem. 9, 1269–1275. doi: 10.1002/cctc.201601544
Millia, L., Dall'Asta, V., Ferrara, C., Berbenni, V., Quartarone, E., Perna, F. M., et al. (2018). Bio-inspired choline chloride-based deep eutectic solvents as electrolytes for lithium-ion batteries. Solid State Ion. 323, 44–48. doi: 10.1016/j.ssi.2018.05.016
Moity, L., Durand, M., Benazzouz, A., Pierlot, C., Molinier, V., and Aubry, J. M. (2012). Panorama of sustainable solvents using the COSMO-RS approach. Green Chem. 14, 1132–1145. doi: 10.1039/c2gc16515e
Mota-Morales, J. D., Sánchez-Leija, R. J., Carranza, A., Pojman, J. A., del Monte, F., and Luna-Bárcenas, G. (2018). Free-radical polymerizations of and in deep eutectic solvents: Green synthesis of functional materials. Prog. Polym. Sci. 18, 139–153. doi: 10.1016/j.progpolymsci.2017.09.005
Narayan, S., Muldoon, J., Finn, M. G., Fokin, V. V., Kolb, H. C., and Sharpless, K. B. (2005). “On water”: unique reactivity of organic compounds in aqueous suspension. Angew. Chem. Int. Ed. 44, 3275–3279. doi: 10.1002/anie.200462883
Palo-Nieto, C, Afewerki, S., Anderson, M., Tai, C. W., Berglund, P., and Córdova, A. (2016). Integrated heterogeneous metal/enzymatic multiple relay catalysis for eco-friendly and asymmetric synthesis. ACS Catal. 6, 3932–3940. doi: 10.1021/acscatal.6b01031
Paris, J., Ríos-Lombardía, N., Morís, F., Gröger, H., and González-Sabín, J. (2018). Novel insights into the combination of metal- and biocatalysis: cascade one-pot synthesis of enantiomerically pure biaryl alcohols in deep eutectic solvents. ChemCatChem. 10, 4417–4423. doi: 10.1002/cctc.201800768
Paris, J., Telzerow, A., Ríos-Lombardía, N., Steiner, K., Schwab, H., Morís, F., et al. (2019). Enantioselective one-pot synthesis of biaryl-substituted amines by combining palladium and enzyme catalysis in deep eutectic solvents. ACS Sustainable Chem. Eng. 7, 5486–5493. doi: 10.1021/acssuschemeng.8b06715
Poliakoff, M., Fitzpatric, J. M., Farren, T. R., and Anastas, P. T. (2002). Green chemistry: science and politics of change. Science 297, 807–810. doi: 10.1126/science.297.5582.807
Quirós-Montes, J., Carriedo, G. A., García-Álvarez, J., and Presa Soto, A. (2019). Deep eutectic solvents for Cu-catalysed ARGET ATRP under an air atmosphere: a sustainable and efficient route to poly(methyl methacrylate) using, a. recyclable. Cu(ii) metal–organic framework. Green Chem. 21, 5865–5875. doi: 10.1039/C9GC02624J
Reichardt, C., and Welton, T. (2010). “The previously mentioned solvent effect is usually connected with the inherent ability of solvents to: i) permit the fine-control of the heat flow in the reaction vessel; ii) reduce unwanted side reactions via dilution; and iii) stabilize reaction intermediates or catalysts,” in Solvents and Solvent Effects in Organic Chemistry, 4th edn. (Weinheim: Wiley-VCH), 1–5. doi: 10.1002/9783527632220
Ríos-Lombardía, N., García-Álvarez, J., and González-Sabín, J. (2018). One-pot combination of metal- and bio-catalysis in water for the synthesis of chiral molecules. Catalysts 8:75. doi: 10.3390/catal8020075
Roda, A., Matias, A. A., Paiva, A., and Duarte, A. R. C. (2019). Polymer science and engineering using deep eutectic solvents. Polymers 11:912. doi: 10.3390/polym11050912
Rodríguez, H., Angulo, I., de las Rivas, B., Campillo, N., Páez, J. A., Muñoz, R., et al. (2010). p-Coumaric acid decarboxylase from Lactobacillus plantarum: structural insights into the active site and decarboxylation catalytic mechanism. Proteins: Struct. Funct. Bioinf. 78, 1662–1676. doi: 10.2210/pdb2w2a/pdb
Rodríguez-Álvarez, M. J., García-Álvarez, J., Uzelac, M., Fairley, M., O'Hara, C. T., and Hevia, E. (2018). Introducing glycerol as, a. sustainable. solvent to organolithium chemistry: ultrafast chemoselective addition of aryllithium reagents to nitriles under air and at ambient temperature. Chem. Eur. J. 24, 1720–1725. doi: 10.1002/chem.201705577
Saavedra, B., González-Gallardo, N., Meli, A., and Ramón, D. J. (2019). A bipyridine-palladium derivative as general pre-catalyst for cross-coupling reactions in deep eutectic solvents. Adv. Synth. Catal. 361, 3868–3879. doi: 10.1002/adsc.201900472
Sánchez-Condado, A., Carriedo, G. A., Presa Soto, A., Rodríguez-Álvarez, M. J., García-Álvarez, J., and Hevia, E. (2019). Organolithium-initiated polymerization of olefins in deep eutectic solvents under aerobic conditions. ChemSusChem. 12, 3134–3143. doi: 10.1002/cssc.201900533
Sassone, F. C., Perna, F. M., Salomone, A., Florio, S., and Capriati, V. (2015). Unexpected lateral-lithiation-induced alkylative ring opening of tetrahydrofurans in deep eutectic solvents: synthesis of functionalised primary alcohols. Chem. Commun. 51, 9459–9462. doi: 10.1039/C5CC02884A
Scalacci, N., Black, G. W., Mattedi, G., Brown, N. L., Turner, N. J., and Castagnolo, D. (2017). Unveiling the biocatalytic aromatizing activity of monoamine oxidases MAO-N and 6-HDNO: development of chemoenzymatic cascades for the synthesis of pyrroles. ACS Catal. 7, 1295–1300. doi: 10.1021/acscatal.6b03081
Schmidt, S., Castiglione, K., and Kourist, R. (2018). Overcoming the incompatibility challenge in chemoenzymatic and multi-catalytic cascade reactions. Chem. Eur. J. 24, 1755–1768. doi: 10.1002/chem.201703353
Schweiger, K., Ríos-Lombardía, N., Winkler, C. K., Schmidt, S., Morís, F., Kroutil, W., et al. (2019). Using deep eutectic solvents to overcome limited substrate solubility in the enzymatic decarboxylation of bio-based phenolic acids. ACS Sustainable Chem. Eng. 7, 16364–16370. doi: 10.1021/acssuschemeng.9b03455
Sheldon, R. A., Arends, I. W. C. E., and Henefeld, U. (2007). Green Chemistry and Catalysis. Weinheim: Wiley-VCH. doi: 10.1002/9783527611003
Sheldon, R. A., and Pererira, P. C. (2017). Biocatalysis engineering: the big picture. Chem. Soc. Rev. 46, 2678–2691. doi: 10.1039/C6CS00854B
Smith, E. L., Abbott, A. P., and Ryder, K. S. (2014). Deep eutectic solvents (DESs) and their applications. Chem. Rev. 114, 11060–11082. doi: 10.1021/cr300162p
Tenbrink, K., Seßler, M., Schatz, J., and Gröger, H. (2011). Combination of olefin metathesis and enzymatic ester hydrolysis in aqueous media in a one-pot synthesis. Adv. Synth. Catal. 353, 2363–2367. doi: 10.1002/adsc.201100403
Tomasek, J., and Schatz, J. (2013). Olefin metathesis in aqueous media. Green Chem. 15, 2317–2338. doi: 10.1039/c3gc41042k
United Nations (2015). Transforming Our World: The 2030. Agenda for Sustainable Development. New York, NY: United Nations - Sustainable Development Knowledge Platform.
van Leeuwen, P. W. N. M. (eds). (2004). Homogeneous Catalysis: Understanding the Art. Amsterdam: Kluwer Academic Publishers. doi: 10.1007/1-4020-2000-7
Verho, O., and Bäckvall, J. E. (2015). Chemoenzymatic dynamic kinetic resolution: a powerful tool for the preparation of enantiomerically pure alcohols and amines. J. Am. Chem. Soc. 137, 3996–4009. doi: 10.1021/jacs.5b01031
Vidal, C., and García-Álvarez, J. (2019). “Metal-Promoted Organic Transformations in DES,” in Deep Eutectic Solvents: Synthesis, Properties and Applications, eds. D. J. Ramón, and G. Guillena (Weinheim: Wiley-VCH). doi: 10.1002/9783527818488.ch9
Vidal, C., García-Álvarez, J., Hernán-Gómez, A., Kennedy, A. R., and Hevia, E. (2014). Introducing deep eutectic solvents to polar organometallic chemistry: chemoselective addition of organolithium and Grignard reagents to ketones in air. Angew. Chem. Int. Ed. 53, 5969–5973. doi: 10.1002/anie.201400889
Vidal, C., García-Álvarez, J., Hernán-Gómez, A., Kennedy, A. R., and Hevia, E. (2016). Exploiting deep eutectic solvents and organolithium reagent partnerships: chemoselective ultrafast addition to imines and quinolines under aerobic ambient temperature conditions. Angew. Chem. Int. Ed. 55, 16145–16148. doi: 10.1002/anie.201609929
Keywords: Deep Eutectic Solvents, chemoenzymatic, metal-catalysis, biocatalysis, metathesis, phenolic acid decarboxylase, Heck reaction
Citation: Ríos-Lombardía N, Rodríguez-Álvarez MJ, Morís F, Kourist R, Comino N, López-Gallego F, González-Sabín J and García-Álvarez J (2020) DESign of Sustainable One-Pot Chemoenzymatic Organic Transformations in Deep Eutectic Solvents for the Synthesis of 1,2-Disubstituted Aromatic Olefins. Front. Chem. 8:139. doi: 10.3389/fchem.2020.00139
Received: 03 December 2019; Accepted: 14 February 2020;
Published: 06 March 2020.
Edited by:
Florent Allais, AgroParisTech Institut des Sciences et Industries du Vivant et de L'environnement, FranceReviewed by:
Christophe Len, University of Technology Compiegne, FranceSimonetta Antonaroli, University of Rome Tor Vergata, Italy
Sandrine Bouquillon, Université de Reims Champagne-Ardenne, France
Copyright © 2020 Ríos-Lombardía, Rodríguez-Álvarez, Morís, Kourist, Comino, López-Gallego, González-Sabín and García-Álvarez. This is an open-access article distributed under the terms of the Creative Commons Attribution License (CC BY). The use, distribution or reproduction in other forums is permitted, provided the original author(s) and the copyright owner(s) are credited and that the original publication in this journal is cited, in accordance with accepted academic practice. No use, distribution or reproduction is permitted which does not comply with these terms.
*Correspondence: Javier González-Sabín, amdzYWJpbkBlbnRyZWNoZW0uY29t; Joaquín García-Álvarez, Z2FyY2lham9hcXVpbkB1bmlvdmkuZXM=