- 1School of Chemistry and Chemical Engineering, Southwest Petroleum University, Chengdu, China
- 2State Key Laboratory of Oil and Gas Geology and Exploitation, Chengdu University of Technology, Chengdu, China
- 3State Key Laboratory of Polymer Molecular Engineering, Fudan University, Shanghai, China
- 4Key Laboratory of Oilfield Chemistry (KLOC), CNPC, Beijing, China
- 5Engineer Technology Research Institute, CNPC Xibu Drilling Engineering Company Limited, Ürümqi, China
- 6China National Offshore Oil Corporation (CNOOC) Energy Development Company Limited, Tianjin, China
To improve CO2 adsorption performance of nanoparticle absorbents, a novel tertiary amine functionalized nano–SiO2 (NS–NR2) was synthesized based on the 3–aminopropyltrimethoxysilane (KH540) modified nano–SiO2 (NS–NH2) via methylation. The chemical structure and performances of the NS–NR2 were characterized through a series of experiments, which revealed that NS–NR2 can react with CO2 in water and nanofluid with low viscosity revealed better CO2 capture. The CO2 capture mechanism of NS–NR2 was studied by kinetic models. From the correlation coefficient, the pseudo second order model was found to fit well with the experiment data. The influencing factors were investigated, including temperature, dispersants, and cycling numbers. Results has shown the additional surfactant to greatly promote the CO2 adsorption performance of NS–NR2 because of the better dispersity of nanoparticles. This work proved that NS–NR2 yields low viscosity, high capacity for CO2 capture, and good regenerability in water. NS–NR2 with high CO2 capture will play a role in storing CO2 to enhanced oil recovery in CO2 flooding.
Introduction
In recent years, humans have been endangered by greenhouse effect leading to global warming. Carbon dioxide (CO2) emission source from the burn of fossil fuels catches much attention because of it is a major factor to the greenhouse effect (Sarkodie and Strezov, 2019). One method to assuage the greenhouse effect is to capture CO2 from emission sources and then save it in stratum or apply it for enhanced oil recovery in low permeability reservoirs. Therefore, a feasible approach called carbon capture and storage (CCS) technologies have developed, including membranes, cryogenic distillation, gas adsorption with liquids or solids, and others (Benson and Orr, 2011; Bui et al., 2018). However, membrane–based separation is not a suitable way for CO2 capture because perfection wants to be made in the areas of CO2 selectivity, permeability, cost, and performance depletion over time (all caused by a variety of factors). Moreover, because of the high energy costs involved, cryogenic distillation is not optimally suitable. Solvents and solid sorbents have been reported for CO2 capture, such as basic solvents, supported amine, and ammonium materials, as the primary classes of chemical sorbents (Heydari-Gorji et al., 2011; Darunte et al., 2016; Hahn et al., 2016; Sanz-Perez et al., 2016; Kong et al., 2019). The adsorption of CO2 by solvents is a commercially available method, but the regeneration process of the solvents is highly energy intensive and expensive (Rochelle, 2009). The adsorption of CO2 by solid sorbents has recently attracted much attention in the study of CO2 capture (Lee and Park, 2015).
Nano silica (SiO2) solid sorbents have been investigated for CO2 capture given their high pore volume, large surface, and ease of functionality (Liu et al., 2016; Lai et al., 2017, 2019; Wang et al., 2019). Jiao et al. (2015) prepared mesoporous silica (MSU–J) with a wormhole framework texture, the surface of MSU–J was modified with triethylenetetramine (TETA) for CO2 capture. Hahn et al. (2016) reported the primary amine, secondary amine, and bibasic amine species functionalized porous SiO2 and discussed the mechanism of CO2 adsorption on the SiO2. Bae (2017) showed that the 3–(2–aminoethylamino) propyldimethoxymetylsilane modified silica can be used as an adsorbent to improve CO2 capture performance and obtained capture CO2 capacity of 2.24 mmol/g. The surface of SiO2 usually has been functionalized with primary and secondary amines for CO2 capture. Amine modified SiO2 reacted with CO2 to form carbamate or bicarbonate species based on the acid–based chemical interaction for improved CO2 adsorption (Huang et al., 2003). Without the presence of water, the amine groups reacted with CO2 molecules to create the carbamates group. As another specific, the presence of water impairs this amino group adsorption (Ma et al., 2017). Therefore, the functionalized nano–SiO2 with water impede is needed to further investigated in future development.
It is difficult to destroy the steady carbon–nitrogen bond of carbamate that is formed in primary amine and secondary amines reaction with CO2. Also, fascinating tertiary amines as absorbents generate bicarbonates to replace carbamates when tertiary amines reacting with CO2 (Crooks and Donnellan, 1990; Vaidya and Kenig, 2007) thereby leading to low energy for regeneration of absorbents. Therefore, tertiary amine as an energy saving absorbent is appropriate comparing with primary amines and secondary amines (Gao et al., 2017). Particularly, the rate of primary amines and secondary amines with CO2 is faster than tertiary amines (Liu et al., 2019a). However, the solubility of CO2 is higher in tertiary amines solution than one in primary amines and secondary amines solution due to different reaction mechanisms. For example, the reaction molar ratio of tertiary amine and CO2 is 1:1 to formed bicarbonate structure, while the reaction molar ratio of primary amine or secondary amine and CO2 is 0.5:1 to formed carbamate structure (Sartorl and Savage, 1983). Tertiary amine can be able to generate a bicarbonate due to no hydrogen on nitrogen when reacted with CO2 and H2O, resulting in a better CO2 adsorption and lower energy depletion for regeneration (Xiao et al., 2016, 2019). Moreover, kinetics is important since it can explain the dynamic adsorption of the sorbent, a lot of kinetic models are applied to the CO2 adsorption property of tertiary amine (Liu et al., 2019b).
Therefore, this study aimed to develop a sorbent to avert the limits of aqueous amine solutions and take advantage of tertiary amines for CO2 capture. The tertiary amine loaded nano–SiO2 was synthesized, and the CO2 capture performance was studied in the presence of water. The CO2 adsorption mechanism was investigated by kinetics, and the viscosity of the absorbent dispersion was measured before and after CO2 adsorption. Finally, tertiary amine functionalized nano–SiO2 (NS–NR2) was investigated further in terms of temperature, dispersants, and cycling numbers.
Experimental Section
Materials
Methylbenzene(C7H8), 3–(trimethoxysilyl)−1–propanamine (KH−540), ethanol (C2H5OH), formic acid (HCOOH), formaldehyde (HCHO), hydrochloric acid (HCl), N, N–dimethylformamide (DMF), and sodium hydroxide (NaOH) were purchased from Chengdu Kelong Chemical Reagent Factory (Sichuan, China). Nano–SiO2 (10–20 nm) was obtained from Aladdin Chemistry Co. (Shanghai, China). All chemical reagents were analytical–grade. CO2 (g) and N2 (g) were purchased from Jingli Gas Company (Chengdu, China). Water was double deionized with a Millipore Milli Q system to produce the 18 MM deionized water.
Synthesis and Characterization of Tertiary Amine Functionalized Nano–SiO2
The nano–SiO2 loaded with primary amines (NS–NH2) was prepared first using 3–Aminopropyltrimethoxysilane (KH540) as modifiers, and then it was used as the matrix material to synthesize branched nanomaterials with a tertiary amine group on its surface (NS–NR2) via methylation of primary amines based on formic acid and formaldehyde. The mechanism is shown in Figure 1.
The specific reaction conditions of NS–NH2, NS–NR2, and the preparation methods of the nanofluid are shown in Supplementary Materials. It is worth noting that add anhydride to promote unreacted primary amine groups undergo acetylation. The optimum reaction conditions of NS–NR2 are displayed in Table 1.
Fourier transform–Infrared (FT–IR) spectra were acquired by the KBr pellet method using a WQF520 spectrometer. Thermogravimetric analysis (TGA) was conducted on a synchronous comprehensive thermal analyzer (Netzsch Scientific Instruments). The microtopography of NS–NR2 was characterized using an electron microscope (ZEISS Libra 200 FE). The carbon and nitrogen contents were detected by elemental analysis using a Var10EL III instrument. The hydrodynamic diameter and proportion of the nanoparticles were received by a BI 200SM wide-angle dynamic light scattering (DLS) instrument. The rheological property of NS–NR2 dispersion was measured with a HAAKE MARS III rheometer at 25°C to assess the viscosity.
CO2 Adsorption and Desorption
NS–NR2 dispersion was introduced into a gas adsorption bottle. The gas adsorption bottle was put in a constant temperature water bath. The gas flow of CO2 was controlled at 1 L/min by a gas flow controller, and CO2 was bubbling into the high concentrated sulfuric acid to adsorb microscale water in CO2 gas in a hermetic wild-mouth bottle. After that, the dry CO2 was flowed through the gas adsorption bottle to reacted with absorbent in the water. The mass change of dispersion was confirmed by an accurate analytical balance (±0.1 mg) until the weight has no change. The amount of adsorption CO2 on nanoparticles could be calculated under a control test (no nanoparticles). As shown in Figure S1, most of the tests were implemented to assure repeatability of the method. The CO2 adsorption capacity of NS–NR2 was measured using a gas adsorption system, as shown in Figure S2. The CO2 desorption experiment was simply carried out by bubbling N2 around 1 L/min instead of injecting CO2 and keeping the system temperature at 25°C to avoid the huge energy depletion.
The mechanism studies of CO2 adsorption into NS–NR2 are often executed using kinetic models including pseudo first order, pseudo second order, and intraparticle diffusion model. The parameter R2 and relative error (ε) were applied to evaluate the reliability of kinetic models in predicting adsorption capacity, as defined in Equation (1). Based on Equation (1), qe, cal is the predicted date acquired from the model analysis, and qe, exp is the experiment date.
The pseudo first order model that introduced by Langergren in 1898 year (Langergren, 1898) is shown in Equation (2):
where qt is the adsorption capacity at a special time, qe is the adsorption capacity at equilibrium, k1 is the constant of pseudo first order with a unit of 1/min. The pseudo second order model (Ho and McKay, 1999) is represented by Equations (3) and (4):
where qt is the adsorption capacity at a special time, qe is the adsorption capacity at equilibrium, k2 is the constant of pseudo second order with a unit of g/mmol min. The intraparticle diffusion model offers the diffusion mechanism of matter in adsorption process as defined in Equation (5):
Where qt is the adsorption capacity at a special time (mmol/g), k is the rate constant of intraparticle diffusion (mmol/g min1/2) and C (mmol/g) is as the thickness of the boundary layer; the intercept is positively correlated with the boundary layer. (Hameed et al., 2008; Yousef et al., 2011). Mass transfer of adsorbate to the adsorbent surface (bulk diffusion) and film diffusion into the internal sites (intraparticle diffusion) and other steps occur in the process of adsorption.
Results and Discussion
Characterization
The FT–IR spectrum of the nanoparticles is shown in Figure 2. Figure 2A shows the strong adsorption peaks at around 3,450 and 1,646 cm−1, suggest the stretching vibration of the –O–H bonds on the surface of silica. The adsorption peaks near 1,106 and 812 cm−1 are the adsorption peaks of the Si–O–Si group, which are characteristic adsorption peaks of SiO2. In the FT–IR spectra of KH540, the adsorption peaks around 3,380 and 1,594 cm−1 are the –N–H stretching and NH2 deformation of hydrogen bonded amino groups (Jiao et al., 2015). The adsorption peak at 1,477 cm−1 is C–N, and the peaks at 2,954 and 2,842 cm−1 are feature adsorption peak of –CH3 and –CH2-, respectively. The peak at 694 cm−1 is the adsorption peak of Si–C (Titinchi et al., 2014). In the FT–IR spectra of NS–NH2, the 2,954 cm−1 peak of –CH3 disappeared, elucidating that the primary amine was grafted on the surface of SiO2. In Figure 2D, the adsorption peak of –CH3 is shown to appear, indicating that –(CH2)3NH2 reacted to –(CH2)3N(CH3)2 on the surface of nano–SiO2.
The microscopic structure of the nanoparticles is shown in Figure 3 as observed from the TEM morphology. The diameter of NS–NR2 was shown to be approximately 15 nm. The nanoparticles aggregated slightly because of the particle size being in the nanometer scale (Zhao et al., 2014). The evidence from DLS analysis (Figures 4A,B) shown the hydrodynamic diameter of NS–NR2 to be approximately 123.6 nm with uniform size distribution. Moreover, it was found that the diameter distribution of nude particles was wider and the agglomeration was more serious than that of the modified nanoparticles.
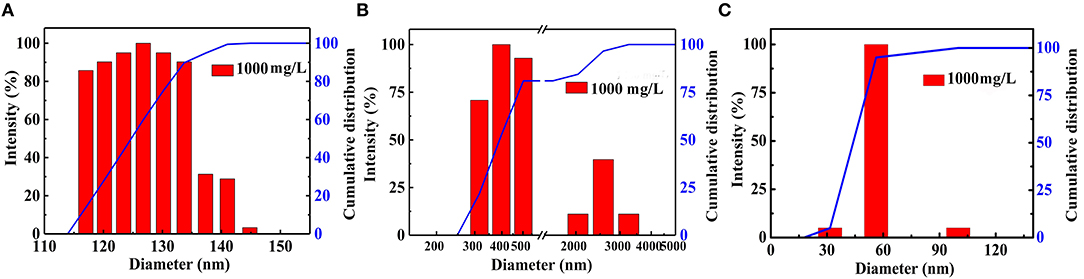
Figure 4. Diameter distribution of (A) NS–NR2 and (B) nude nanoparticles in water; (C) Diameter distribution of NS–NR2 in PEG−400 solution (1,000 mg/L).
The element contents of raw SiO2, NS–NH2, and NS–NR2 are shown in Table 2. Carbon and nitrogen contents are present in raw SiO2 because many SiO2 materials are often synthesized in an aqueous organic solvent, resulting in remaining carbon and nitrogen in such materials. The contents of carbon and nitrogen for NS–NH2 were shown to be 5.22 and 1.65 mmol/g, respectively, and for NS–NR2 were shown to be 7.14 and 1.39 mmol/g, respectively. The contents of these elements were much higher than raw SiO2. The nitrogen amount in NS–NR2 was lower than that of NS–NH2 because when the molar amount of carbon increases, the molar amount of nitrogen decreases in fixed mass products. The molar ratio of carbon to nitrogen is 3.16, 5.13 in NS–NH2 (SiO2-C3H8N) and NS–NR2 (SiO2-C5H10N), respectively. Here, the molar ratio of carbon to nitrogen was adopted to further confirm the grafting of tertiary amines on nano–SiO2.
The successful synthesis of NS–NR2 could also be proved by thermos gravimetric analysis (TGA). Based on the TGA curves in Figure 5, the weight retention of raw nano-SiO2, NS–NH2 and NS–NR2 at 900°C under the air atmosphere were 96.86, 89.38, and 87.06%, respectively. For raw nano-SiO2, the mass depletion is attributed to the surface dihydroxylation. In terms of the structure of NS–NH2 and NS–NR2, when the temperature up to 900°C, the primary amine and tertiary amine chains were grafted on the nano-SiO2 has decomposed, respectively. Therefore, compared with the TGA curve of raw nano-SiO2, the surface of nano-SiO2 was modified to primary amine. The different weight loss between in the NS–NH2 and NS–NR2 indicated that tertiary amine was synthesized from primary amine successfully.
CO2 Adsorption and Kinetic Studies
Any amine with a pKa value >5 can react with CO2 in the presence of water (Field and Grolimund, 1988). According to Figure S3A, the pH value of NS–NR2 dispersion decreased gradually with the addition of diluted hydrochloric acid (0.01 mol/L). The second derivative of the VHCl-pH curve was obtained (Figure S3B), with the zero point of the second derivative corresponding to the extreme point of the curve in Figure S3A. As a result, the pKa value was 7.08. The pKa value of 7.08 indicates that NS–NR2 dispersion can complete adsorption of CO2. Along with the aeration of CO2 at 1 L/min, the pH value of NS–NR2 dispersion dropped rapidly in the first 5 min, after which there were no changes in pH value for 20 min, as shown in Figure S3C, which means that NS–NR2 dispersion (0.1 wt.%) completely saturated CO2 at 1 L/min CO2 gas velocity in 20 min.
It is well-known that CO2 capture is significant influenced by the viscosity of absorbent (Xiao et al., 2019). Figure 6 demonstrates the rheological property of NS–NR2 dispersion before and after adsorption CO2. A rheological plateau in the shear rate region was found for NS–NR2 dispersion. Before CO2 adsorption, the nanofluid viscosity was 2.23 mPa·s. After saturation with CO2, there was a nanofluid viscosity increase to 2.96 mPa·s. This viscosity change is consistent with the ionic liquids in Xiao et al.'s work (Xiao et al., 2019). A possible conclusion is the increase of electrostatic interaction of chains on the NS–NR2 surface due to CO2 adsorption (Figure 7), resulting in the higher viscosity. However, unlike the high viscosity of ionic liquids, the nanofluid viscosity was very low. The CO2 capture was not influenced by the increased viscosity.
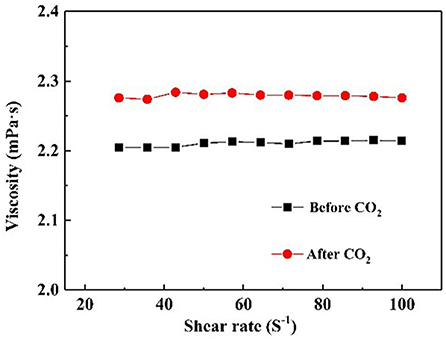
Figure 6. Viscosity of NS–NR2 dispersion before and after adsorption of CO2 as a function of shear rate.
The CO2 adsorption capacity of NS–NR2 was 25 mmol CO2/g NS–NR2 in water (0.1 wt.%) at 25°C, as shown in Table 3. Compared with other materials (with CO2 adsorption capacity of 0.1–21.45 mmol/g) (Yu et al., 2012), NS–NR2 in water has a better CO2 adsorption capacity.
Kinetic models, such as pseudo first order, pseudo second order, and intraparticle diffusion model, were applied to model the test data. The pseudo first order model is mostly appropriated to describe purely physisorption process without considering the any chemical reaction between CO2 molecules and sorbent. The pseudo second order model is mostly appropriated to describe purely chemisorption processes with stable chemical bonds between CO2 molecules and the sorbent. The comparison between the test curves and simulative curves are shown in Figure 8. The model parameters of kinetic at different temperatures and their corresponding coefficients are shown in Table 4. The R2 parameters of pseudo second order and pseudo first order models with experimental data were 0.99 and 0.97, respectively. The relative error for the pseudo second order model, ε, was lower than pseudo first order model. Compared with the R2, ε parameters, the pseudo second order model was found to fit well with the experiment data. Therefore, chemisorption of CO2 on nanoparticles plays a dominant role in CO2 capture.
The pseudo first order model and pseudo second order model provide interaction insight into the actual mechanism of CO2 adsorption. The surface of the particles is grafted with functional groups to adsorb CO2. This surface adsorption was further verified by the intraparticle diffusion. The model parameters of intraparticle diffusion are revealed in Table 4. It is notable that no linear curve can pass through the origin point that is thought to be caused by intraparticle diffusion, suggesting that intraparticle diffusion is not the only factor controlling CO2 adsorption rate at all tested temperatures (Rashidi et al., 2013). Therefore, the adsorption process is not completely controlled by intraparticle diffusion, surface diffusion also plays a role in the whole CO2 adsorption process.
Effect of Some Factors on CO2 Adsorption With NS–NR2
Effect of Temperature
A CO2 capture test was implemented using 0.1 wt.% nanofluid at contrast temperatures of 25, 30, 40, 50, and 60°C, respectively. The CO2 adsorption- temperature curve is revealed in Figure 9A. It is obvious that the maximum CO2 loading on the nanoparticles decreased at higher temperatures. Higher temperatures go against CO2 adsorption that is an exothermic reaction. The CO2 adsorption of NS–NR2 compared with that of MSU–J modified with TETA (Jiao et al., 2015) at higher temperatures is displayed in Figure 9B. The relative comparison presents a view of the advantages of the proposed nanoparticles although a slight difference in the experimental conditions. For the NS–NR2 absorbent in this work, the CO2 loading at 60°C decreased to 48% of the CO2 loading at 25°C, while the CO2 loading of MSU–J at 55°C decreased to 73% of the CO2 loading at 25°C. This result can be ascribed to the different mechanisms of CO2 adsorption. For modified MSU–J absorbent, huge energy was used to generate carbamate group with CO2. But adsorption of CO2 loaded nanoparticles enabled bicarbonate formation much easier because of the physical adsorption and chemical adsorption. This result indicates that the NS–NR2 in this work can capture more CO2 at higher temperatures.
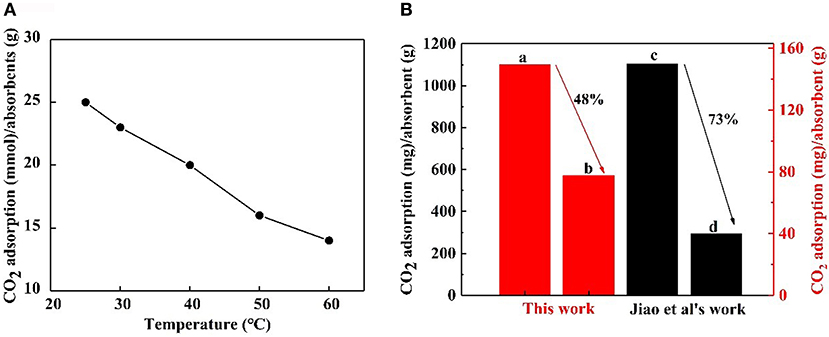
Figure 9. (A) CO2 adsorption of NS-NR2 in water at different temperatures. (B) CO2 adsorption of NS–NR2 at (A) 25°C and (B) 60°C; CO2 adsorption of MSU–J at (C) 25°C and (D)55°C.
Effect of Dispersants
CO2 adsorption capacity in different dispersant agents were investigated. According to the previous test is shown in Figure S3C, it was not necessary to perform the experiment for longer than 20 min. Hence, CO2 adsorption with NS–NR2 was performed at a temperature of 30°C in different dispersants during this period of 15 min. CO2 adsorption curves are drawn in Figure 10. The maximum CO2 loading of NS–NR2 was changed in water or PEG−400 dispersants. The maximum CO2 loadings of NS–NR2 in water and PEG−400 solution was 23.16 and 105.34 mmol/g, respectively. This result can be explained by the diameter distribution of nanoparticles, where the diameter distribution in water was 123.6 nm and in PEG−400 solution was 56.7 nm (Figure 4C). The tertiary amine groups as specific sites for CO2 adsorption grafted on the surface of nano–SiO2 to endow the adsorbents with CO2 adsorption. Therefore, the more specific sites were exposed on the surface of nanoparticles because of better dispersibility. The dispersibility of nanoparticles is certified by the Derjaguin Landau Verwey Overbeek (DLVO) theory on interparticle interaction potential (Ilyas et al., 2014). The interaction among nanoparticles is caused by electrostatic repulsion and steric resistance in the PEG−400 solution. In addition, using PEG−400 as the dispersant is inexpensive and has lower surfactivity. Surfactants with similar properties can also be used to disperse the nanoparticles for CO2 capture. Therefore, adding the surfactant improved CO2 adsorption by enhancing the dispersibility of nanoparticles.
Effect of Cycling Numbers
It is crucial that an absorbent is reusable and retains an efficient CO2 adsorption capacity (Ma et al., 2017). Therefore, the regeneration tests of the NS–NR2 absorbent was carried out at 25°C. After CO2 adsorption in the nanofluid for 20 min, the nanofluid was shifted to CO2 desorption for regeneration. The CO2 desorption test was executed for another 20 min by bubbling N2 around 1 L/min before the absorbent was used for the next round of adsorption. As illustrated in Figure 11, five cycles of adsorption were implemented and the initial CO2 adsorption of absorbent was set as the 100 % baseline. After five cycles, NS–NR2 adsorbent shown favorable regeneration capacity with a slight decrease of 14.3% in water for NS–NR2 compared to the initial capacity. The results shown regeneration and efficient CO2 adsorption capacity of nanoparticles adsorbent. Considering cycling capacity, NS–NR2 material shows intriguing regeneration ability for CO2 adsorption.
Conclusion
This work synthesized tertiary amino functionalized nano–SiO2 successfully. The measurements of pKa value and nanofluid viscosity change proved that NS-NR2 can react with CO2 in water and nanofluid has a low viscosity. NS–NR2 shown better CO2 adsorption capacity, and adsorption kinetics revealed the pseudo second order model was found to fit well with the experiment data. The influence of factors such as temperature, dispersants, and cycling numbers on CO2 adsorption was investigated. Results indicated higher temperature to work against CO2 adsorption of NS–NR2. The CO2 adsorption performance of NS–NR2 was greatly promoted because of a better dispersity of nanoparticles with added surfactant. After recycling of absorbent, the NS–NR2 maintained an efficient CO2 capture and shown favorable regeneration capacity. The measurements of NS–NR2 properties on the bases of viscosity, kinetic models, CO2 capture, and regeneration manifests that NS–NR2 exhibits satisfying performance to capture CO2. NS–NR2 with high CO2 capture will play a role in storing CO2 to enhanced oil recovery in CO2 flooding.
Data Availability Statement
All datasets generated for this study are included in the article/Supplementary Material.
Author Contributions
NL, QZ, and LT conceived the idea. QZ, WH, TY, and YC implemented the preparation, characterization, and measurement of NS–NR2. QZ wrote the manuscript. DQ and KC came up with ideas for the manuscript. QZ, LT, and DW discussed and analyzed the experiment data. LT and DW revised the manuscript. NL supervised the whole research work.
Funding
This work was supported by the National Natural Science Foundation of China (No. 51674208); Nanchong Science and Technology Project (NC17SY4017); State Key Laboratory of Molecular Engineering of Polymers (Fudan University) (No. K2017-25); PetroChina Innovation Foundation (No. 2018D−5007–0207); Open Foundation of State Key Laboratory of Oil and Gas Geology and Exploitation (Cheng Du University of Technology) (No. PLC20180103); Basic Research Programs of Sichuan Province (No. 2018JY0515); and the Undergraduate Extracurricular Open Experiment of Southwest Petroleum University (KSZ18440, KSZ18441, KSZ18442).
Conflict of Interest
DQ was employed by the Engineer Technology Research Institute, CNPC Xibu Drilling Engineering Company Limited company.
The remaining authors declare that the research was conducted in the absence of any commercial or financial relationships that could be construed as a potential conflict of interest.
Supplementary Material
The Supplementary Material for this article can be found online at: https://www.frontiersin.org/articles/10.3389/fchem.2020.00146/full#supplementary-material
References
Bae, J. Y. (2017). CO2 capture by amine-functionalized mesoporous hollow silica. J. Nanosci. Nanotechno. 17, 7418–7422. doi: 10.1166/jnn.2017.14778
Benson, S. M., and Orr, F. M. (2011). Carbon dioxide capture and storage. MRS Bull. 33, 303–305. doi: 10.1557/mrs2008.63
Bui, M., Adjiman, C. S., Bardow, A., Anthony, E. J., Boston, A., Brown, S., et al. (2018). Carbon capture and storage (CCS): the way forward. Energy Environ. Sci. 11, 1062–1176. doi: 10.1039/C7EE02342A
Crooks, J. E., and Donnellan, J. P. (1990). Kinetics of the reaction between carbon dioxide and tertiary amines. J. Org. Chem. 55, 1372–1374. doi: 10.1021/jo00291a056
Darunte, L. A., Walton, K. S., Sholl, D. S., and Jones, C. W. (2016). CO2 capture via adsorption in amine-functionalized sorbents. Curr. Opin. Chem. Eng. 12, 82–90. doi: 10.1016/j.coche.2016.03.002
Field, S. M., and Grolimund, K. (1988). Basicity limits for solutes in supercritical fluid chromatography with a carbon dioxide mobile phase. J. High Res. Chrom. 11, 727–729. doi: 10.1002/jhrc.1240111010
Gao, H., Xu, B., Han, L., Luo, X., and Liang, Z. (2017). Mass transfer performance and correlations for CO2 absorption into aqueous blended of DEEA/MEA in a random packed column. AIChE J. 63, 3048–3057. doi: 10.1002/aic.15673
Hahn, M. W., Jelic, J., Berger, E., Reuter, K., Jentys, A., and Lercher, J. A. (2016). Role of amine functionality for CO2 chemisorption on silica. J. Phys. Chem. B 120, 1988–1995. doi: 10.1021/acs.jpcb.5b10012
Hameed, B. H., Tan, I. A. W., and Ahmad, A. L. (2008). Adsorption isotherm, kinetic modeling and mechanism of 2,4,6-trichlorophenol on coconut husk-based activated carbon. Chem. Eng. J. 144, 235–244. doi: 10.1016/j.cej.2008.01.028
Heydari-Gorji, A., Belmabkhout, Y., and Sayari, A. (2011). Polyethylenimine-impregnated mesoporous silica: effect of amine loading and surface alkyl chains on CO2 adsorption. Langmuir 27, 12411–12416. doi: 10.1021/la202972t
Ho, Y. S., and McKay, G. (1999). Pseudo-second order model for sorption processes. Process Biochem. 34, 451–465. doi: 10.1016/S0032-9592(98)00112-5
Huang, H. Y., Yang, R. T., Chinn, D., and Munson, C. L. (2003). Amine-grafted MCM-48 and silica xerogel as superior sorbents for acidic gas removal from natural gas. Ind. Eng. Chem. Res. 42, 2427–2433. doi: 10.1021/ie020440u
Ilyas, S. U., Pendyala, R., and Marneni, N. (2014). Preparation, sedimentation, and agglomeration of nanofluids. Chem. Eng. Technol. 37, 2011–2021. doi: 10.1002/ceat.201400268
Jiao, J., Cao, J., and Lv, P. P. (2015). Amine-immobilized three-dimensional wormhole mesostructured MSU-J silica for CO2 adsorption: effect of amine loading and temperature on the adsorption capacity. Chem. Lett. 44, 928–930. doi: 10.1246/cl.141151
Kong, X., Li, S., Stromme, M., and Xu, C. (2019). Synthesis of porous organic polymers with tunable amine loadings for CO2 capture: balanced physisorption and chemisorption. Nanomaterials 9:E1020. doi: 10.3390/nano9071020
Lai, N., Li, S., Liu, L., Li, Y., Li, J., and Zhao, M. (2017). Synthesis and rheological property of various modified nano-SiO2/AM/AA hyperbranched polymers for oil displacement. Russ. J. Appl. Chem. 90, 480–491. doi: 10.1134/S1070427217030235
Lai, N., Tang, L., Jia, N., Qiao, D., Chen, J., Wang, Y., et al. (2019). Feasibility study of applying modified nano-SiO2 hyperbranched copolymers for enhanced oil recovery in low-mid permeability reservoirs. Polymers. 11:E1483. doi: 10.3390/polym11091483
Langergren, S. (1898). Zur theorie der sogenannten adsorption gelster stoffe. Kungliga Svenska Vetenskapsakademiens. Handlingar 24, 1–39.
Lee, Y., and Park, J. (2015). A review on solid adsorbents for carbon dioxide capture. J. Ind. Eng. Chem. 23, 1–11. doi: 10.1016/j.jiec.2014.09.001
Liu, H., Chan, C., Tontiwachwuthikul, P., and Idem, R. (2019a). Analysis of CO2 equilibrium solubility of seven tertiary amine solvents using thermodynamic and ANN models. Fuel 249, 61–72. doi: 10.1016/j.fuel.2019.02.088
Liu, H., Idem, R., and Tontiwachwuthikul, P. (2019b). Novel models for correlation of solubility constant and diffusivity of N2O in aqueous 1-dimethylamino-2-propanol. Chem. Eng. Sci. 203, 86–103. doi: 10.1016/j.ces.2019.03.073
Liu, Y., Huang, N., Yu, Y., Zheng, C., Deng, N., and Liu, J. (2016). Bioactive SiO2@Ru nanoparticles for osteogenic differentiation of mesenchymal stem cells via activation of Akt signaling pathways. J. Mater. Chem. B 4, 4389–4401. doi: 10.1039/C5TB01898F
Ma, Y., Wang, Z., Xu, X., and Wang, J. (2017). Review on porous nanomaterials for adsorption and photocatalytic conversion of CO2. Chinese J. Catal. 38, 1956–1969. doi: 10.1016/S1872-2067(17)62955-3
Rashidi, N. A., Yusup, S., and Hameed, B. H. (2013). Kinetic studies on carbon dioxide capture using lignocellulosic based activated carbon. Energy 61, 440–446. doi: 10.1016/j.energy.2013.08.050
Rochelle, G. T. (2009). Amine scrubbing for CO2 capture. Science 325, 1652–1654. doi: 10.1126/science.1176731
Sanz-Perez, E. S., Murdock, C. R., Didas, S. A., and Jones, C. W. (2016). Direct capture of CO2 from ambient air. Chem. Rev. 116, 11840–11876. doi: 10.1021/acs.chemrev.6b00173
Sarkodie, S. A., and Strezov, V. (2019). Effect of foreign direct investments, economic development and energy consumption on greenhouse gas emissions in developing countries. Sci. Total Environ. 646, 862–871. doi: 10.1016/j.scitotenv.2018.07.365
Sartorl, G., and Savage, D. W. (1983). Sterically hindered amines for CO2 removal from gases. Ind. Eng. Chem. Fundam. 22, 239–249. doi: 10.1021/i100010a016
Titinchi, S. J. J., Piet, M., Abbo, H. S., Bolland, O., and Schwieger, W. (2014). Chemically modified solid adsorbents for CO2 capture. Energy Proc. 63, 8153–8160. doi: 10.1016/j.egypro.2015.12.337
Vaidya, P. D., and Kenig, E. Y. (2007). CO2-alkanolamine reaction kinetics: a review of recent studies. Chem. Eng. Technol. 30, 1467–1474. doi: 10.1002/ceat.200700268
Wang, F., Gao, S., Pan, J., Li, X., and Liu, J. (2019). Short-chain modified SiO2 with high absorption of organic PCM for thermal protection. Nanomaterials 9:E657. doi: 10.3390/nano9040657
Xiao, M., Liu, H., Gao, H., Olson, W., and Liang, Z. (2019). CO2 capture with hybrid absorbents of low viscosity imidazolium-based ionic liquids and amine. Appl. Energy 235, 311–319. doi: 10.1016/j.apenergy.2018.10.103
Xiao, M., Liu, H., Idem, R., Tontiwachwuthikul, P., and Liang, Z. (2016). A study of structure–activity relationships of commercial tertiary amines for post-combustion CO2 capture. Appl. Energy 184, 219–229. doi: 10.1016/j.apenergy.2016.10.006
Yousef, I., ElEswed, B., and AlMuhtaseb, A. A. H. (2011). Adsorption characteristics of natural zeolites as solid adsorbents for phenol removal from aqueous solutions: kinetics, mechanism, and thermodynamics studies. Chem. Eng. J. 171, 1143–1149. doi: 10.1016/j.cej.2011.05.012
Yu, C. H., Huang, C. H., and Tan, C. S. (2012). A review of CO2 capture by absorption and adsorption. Aerosol Air Qual. Res. 12, 745–769. doi: 10.4209/aaqr.2012.05.0132
Keywords: nano–SiO2, tertiary amine, CO2 capture, low viscosity, regenerability
Citation: Lai N, Zhu Q, Qiao D, Chen K, Tang L, Wang D, He W, Chen Y and Yu T (2020) CO2 Capture With Absorbents of Tertiary Amine Functionalized Nano–SiO2. Front. Chem. 8:146. doi: 10.3389/fchem.2020.00146
Received: 11 November 2019; Accepted: 17 February 2020;
Published: 28 February 2020.
Edited by:
Qing You, China University of Geosciences, ChinaReviewed by:
Helei Liu, University of British Columbia, CanadaGuillermo Javier Copello, National Council for Scientific and Technical Research (CONICET), Argentina
Copyright © 2020 Lai, Zhu, Qiao, Chen, Tang, Wang, He, Chen and Yu. This is an open-access article distributed under the terms of the Creative Commons Attribution License (CC BY). The use, distribution or reproduction in other forums is permitted, provided the original author(s) and the copyright owner(s) are credited and that the original publication in this journal is cited, in accordance with accepted academic practice. No use, distribution or reproduction is permitted which does not comply with these terms.
*Correspondence: Nanjun Lai, bGFpbmFuanVuQDEyNi5jb20=