Targeting L-Proline Uptake as New Strategy for Anti-chagas Drug Development
- 1Facultad de Ciencias Bioquímicas y Farmacéuticas, Instituto de Química de Rosario (IQUIR), Universidad Nacional de Rosario, Rosario, Argentina
- 2Instituto de Biología Molecular y Celular de Rosario (IBR), Consejo Nacional de Investigaciones Científicas y Técnicas CONICET–Facultad de Ciencias Bioquímicas y Farmacéuticas, Universidad Nacional de Rosario, Rosario, Argentina
- 3Laboratory of Biochemistry of Tryps-LaBTryps, Departamento de Parasitologia, Instituto de Ciências Biomédicas, Universidade de São Paulo, Cidade Universitária, São Paulo, Brazil
- 4Departamento de Química Orgánica, Facultad de Ciencias Bioquímicas y Farmacéuticas, Universidad Nacional de Rosario, Rosario, Argentina
L-Proline is an important amino acid for the pathogenic protists belonging to Trypanosoma and Leishmania genera. In Trypanosoma cruzi, the etiological agent of Chagas disease, this amino acid is involved in fundamental biological processes such as ATP production, differentiation of the insect and intracellular stages, the host cell infection and the resistance to a variety of stresses. In this study, we explore the L-Proline uptake as a chemotherapeutic target for T. cruzi. Novel inhibitors have been proposed containing the amino acid with a linker and a variable region able to block the transporter. A series of sixteen 1,2,3-triazolyl-proline derivatives have been prepared for in vitro screening against T. cruzi epimastigotes and proline uptake assays. We successfully obtained inhibitors that interfere with the amino acid internalization, which validated our design targeting the metabolite's transport. The presented structures are one of few examples of amino acid transporter inhibitors. The unprecedent application of this strategy on the development of new chemotherapy against Chagas disease, opens a new horizon on antiparasitic drug development against parasitic diseases and other pathologies.
Introduction
Chagas disease is one of the most neglected infectious disease. It is endemic in the Americas, with 8–10 million people infected and 25 million people at risk (Nunes et al., 2013). The disease is divided in the acute and the chronic phase. The first have a noticeable parasitemia, the absence of humoral response and is largely asymptomatic. The chronic phase has a non-evident parasitemia and a robust IgG response being asymptomatic in 60–70% of the cases (Pérez-Molina and Molina, 2018). The chemotherapy against Chagas disease relies mainly on two drugs introduced more than 40 years ago: Nifurtimox (Nf) and Benznidazole (Bz) (Urbina, 2010). Both drugs are efficient on the acute phase, but in the chronic phase is controversial (Morillo et al., 2015). In addition, severe side effects due to toxicity and the emergence of resistance calls for urgent development of new drugs (Guedes et al., 2011).
Trypanosoma cruzi is a hemoflagellated parasite that causes Chagas disease. This parasite presents a complex life cycle among two kinds of hosts: mammals and reduviid insects, which transmit the infection. Along its life-cycle at least four stages were clearly identified, epimastigotes (replicative stage) and metacyclic trypomastigotes (infective, non-replicative stage) in the insect and blood stream trypomastigotes and amastigotes (intracellular replicative stage). Also, during its life-cycle the parasite faces different environments and it must adjust its “life-style” including its metabolism to changes in nutrients availability, temperature, together other environmental variables.
Among many other important metabolites, amino acids are particularly relevant for the biology of T. cruzi, besides protein synthesis (Marchese et al., 2018), playing fundamental roles in energy management (Pereira et al., 2000) and nitrogen metabolism (Crispim et al., 2018; Girard et al., 2018). When epimastigotes proliferation arrests, there is a metabolic switch from a carbohydrates to an amino acids based metabolism, with a consequent change in the protein expression profile (Barisón et al., 2017; Avila et al., 2018). In fact, it has been demonstrated that amino acids such as proline (Paes et al., 2013), histidine (Barison et al., 2016), and even alanine (Girard et al., 2018), as well as the proline oxidation product P5C (Mantilla et al., 2015), can fuel electrons to the respiratory chain, powering the mitochondrial ATP synthesis (Sylvester and Krassner, 1976; Martins et al., 2009; Paes et al., 2013; Barison et al., 2016). Some neutral amino acids can also function as osmolytes, serving to counteract volume perturbations following a shift in extracellular osmolarity (Rohloff et al., 2003; Silber et al., 2005; Avila et al., 2018).
Particularly, proline is involved in energization of the host-cells invasion by metacyclic trypomastigotes (Martins et al., 2009), as well as growth and differentiation of the insect (Contreras Vt et al., 1988; Tonelli et al., 2004; Silber et al., 2009) and the intracellular stages (Tonelli et al., 2004). Additionally, its accumulation in the parasite cytoplasm provides resistance to oxidative and thermal stress (Tonelli et al., 2004; Magdaleno et al., 2009; Paes et al., 2013; Sayé et al., 2014). The proline availability is mediated by an interplay of the biosynthesis degradation and uptake process (Sylvester and Krassner, 1976; Silber et al., 2002; Magdaleno et al., 2009; Paes et al., 2013; Sayé et al., 2014; Mantilla et al., 2017). In particular, the inhibition of proline uptake by competitive transporter interrupters, diminished the parasites ability to tolerate oxidative imbalance, nutritional stress and to complete the infection cycle (Magdaleno et al., 2009).
Taking the proline uptake as a novel drug target we decided to develop new transporter inhibitors and evaluate their antiproliferative activity against Trypanosoma cruzi. These new compounds were initially evaluated on T. cruzi epimastigotes, validating their action mechanism by proline transport experiments. A comprehensive analysis of the structure-activity relationship allowed a rational pipeline to design selective metabolite transporter inhibitors.
Materials and Methods
Chemistry
General Remarks
Chemical reagents were purchased from commercial suppliers and used without further purification, unless otherwise noted. Dry, deoxygenated diethyl ether (Et2O), tetrahydrofuran (THF), and dichloromethane (DCM) were obtained bypassing commercially available pre-dried, oxygen-free solvents through activated alumina columns. DMF was distilled from BaO. Reactions were monitored by thin-layer chromatography (TLC) performed on 0.2 mm Merck silica gel aluminum plates (60F-254) and visualized using ultraviolet light (254 nm) and by potassium permanganate and heat as developing reagents. All reactions were performed under an atmosphere of nitrogen using oven-dried glassware and standard syringe/septa techniques. Column chromatography was performed with silica gel 60 (230–400 mesh). Yields were calculated for material judged homogeneous by thin layer chromatography (TLC) and nuclear magnetic resonance (1H NMR).
1H and 13C NMR spectra were acquired on a Bruker Avance II 300 MHz (75.13 MHz) using CDCl3 as solvent. Chemical shifts (δ) were reported in ppm downfield from tetramethylsilane and coupling constants are in hertz (Hz). NMR spectra were obtained at 298 K unless otherwise stated and samples run as a dilute solution of the stated solvent. All NMR spectra were referenced to the residual undeuterated solvent as an internal reference. The following abbreviations were used to explain the multiplicities: s = singlet, d = doublet, t = triplet, q = quartet, m = multiplet, br = broad. Assignment of proton resonances was confirmed by correlated spectroscopy. Electrospray ionization high-resolution mass spectra (ESI-HRMS) were recorded on a Bruker MicroTOF II. IR spectra were obtained using an FT-IR Shimadzu spectrometer and only partial spectral data are listed. Melting points were measured on an Electrothermal 9100 apparatus and are uncorrected.
Experimental Procedures and Spectroscopic Data
Synthesis of N-Propargyl Methyl Prolinate (2)
To a solution of methyl prolinate (200 mg, 1.22 mmol) in 10 mL of Et2O(anh), NEt3 (439 mg, 4.3 mmol) and 80 % propargyl bromide in toluene (263 μL, 2.45 mmol) were added in this order and the reaction mixture was stirred at room temperature for 12 h. The solvent was evaporated, and the crude product was purified by column chromatography in silica gel with increasing ethyl acetate/hexane gradient to yield the expected product as a yellow oil (129 mg, 72 %).
1H NMR (300 MHz, CDCl3): δ 3.72 (s, 3H, OCH3), 3.61–3.55 (m, 2H, C5-H, C2-H), 3.45–3.39 (m, 1H, C6-H), 3.07–3.01 (m, 1H, C6-H), 2.75–2.67 (m, 1H, C5-H), 2.20−2.06 (m, 2H, C7-H and C3-H), 2.08–1.76 (m, 3H, C3-H, C4-H). 13C NMR (75 MHz, CDCl3): δ 173.9 (COO), 78.2 (C), 73.2 (CH), 62.4 (CH), 52.1 (CH2), 51.9 (OCH3), 41.1 (CH2), 29.5 (CH2), 23.2 (CH2).
General Procedure for the Cu(I) Mediated 1,3-Dipolar Cycloaddition
N-Propargyl methyl prolinate (1 eq) and the azide (1.1 eq) were suspended in 10 mL/eq of tBuOH:H2O (1:1) and then 1M CuSO4 solution (0.05 eq) and finally 1M sodium ascorbate solution (0.2 eq) and the mixture stirred overnight at room temperature. Brine was added, and the solution was extracted with dichloromethane. Combined organic extracts were dried over sodium sulfate and evaporated. Products were purified by column chromatography in silica gel with increasing hexanes/ethyl acetate/methanol gradients.
Methyl ((1-(2-Ethoxy-2-Oxoethyl)-1H-1,2,3-Triazol-4-yl)Methyl)-L-Prolinate (3a)
Compound 3a was prepared from 45 mg (0.27 mmol) of N-propargyl methyl prolinate 1, following the general procedure for the 1,3-dipolar cycloaddition, affording 66 mg of a yellowish oil in 80 % yield. 1H NMR (300 MHz, CDCl3): δ 7.62 (s, 1H, C7-H), 5.09 (d, J = 2.6 Hz, 2H, C8-H), 4.20 (q, J = 7.5 Hz, 2H, CH2CH3), 4.01 (d, J = 13.8 Hz, 1H, C6-H), 3.82 (d, J = 13.8 Hz, 1H, C6-H), 3.64 (s, 3H, OMe), 3.29 (dd, J = 8.7, 6.1 Hz, 1H, C2-H), 3.10–3.04 (m, 1H, C5-H), 2.50 (dt, J = 8.4 Hz, J = 8.2 Hz, 1H, C5-H), 2.07–1.74 (m, 4H, C3-H and C4-H), 1.23 (t, J = 7.5 Hz, 3H, CH2CH3). 13C NMR (75 MHz, CDCl3): δ 174.1 (COO), 166.3 (COO), 144.5 (C6'), 124.4 (C7), 64.4 (C2), 62.4 (CH2), 53.0 (C5), 52.0 (OCH3), 50.8 (C8), 48.2 (C6), 29.3 (C3), 23.0 (C4), 14.0 (CH3). IR (film): υmax 3458, 3439, 2954, 2357, 1732, 1643, 1444, 1217, 1051, 1024, 875, 798, 756 cm−1. ESI-HRMS m/z [M+K]+ calcd for C13H20KN4O4 335.1116, found 335.1113.
Methyl ((1-(5-Ethoxy-5-Oxopentyl)-1H-1,2,3-Triazol-4-yl)Methyl)-L-Prolinate (3b)
Compound 3b was prepared from 45 mg (0.27 mmol) of N-propargyl methyl prolinate 1, following the general procedure for the 1,3-dipolar cycloaddition, affording 63 mg of a yellowish oil in 69 % yield. 1H NMR (300 MHz, CDCl3): δ 7.50 (s, 1H, C7-H), 4.38 (d, J = 2.6 Hz, 2H, C8-H), 4.09 (q, J = 7.5 Hz, 2H, CH2CH3), 3.97 (d, J = 13.8 Hz, 1H, C6-H), 3.79 (d, J = 13.8 Hz, 1H, C6-H), 3.65 (3H, OMe), 3.28 (dd, J = 8.7, 6.1 Hz, 1H, C2-H), 3.12–3.07 (m, 1H, C5-H), 2.53–2.45 (m, 1H, C5-H), 2.32–2.08 (m, 6H, CH2), 1.91–1.76 (m, 4H, C3-H and C4-H), 1.20 (t, J = 7.5 Hz, 3H, CH2CH3). 13C NMR (75 MHz, CDCl3): δ 174.4 (C=O), 172.3 (COO), 144.5 (C6'), 122.8 (C7), 64.6 (C2), 60.6 (CH2CH3), 53.3 (C5), 51.8 (OCH3), 49.1 (C8), 48.5 (C6), 30.6 (CH2), 29.6 (CH2), 29.3 (C3), 25.4 (C4), 23.0 (CH2), 14.1 (CH2CH3). IR (film): υmax 3437, 3138, 2954, 2358, 1730, 1633, 1444, 1377, 1348, 1274, 1199, 1047, 1028, 854, 802 cm−1. ESI-HRMS m/z [M+H]+ calcd for C16H27N4O4 339.2027, found 339.2029.
Methyl ((1-Benzyl-1H-1,2,3-Triazol-4-yl)Methyl)-L-Prolinate (3c)
Compound 3c was prepared from 45 mg (0.27 mmol) of N-propargyl methyl prolinate 1, following the general procedure for the 1,3-dipolar cycloaddition, affording 66 mg of a yellowish oil in 80 % yield. 1H NMR (300 MHz, CDCl3): δ 7.42 (s, 1H, C7-H), 7.36–7.30 (m, 3H, Ph), 7.27–7.21 (m, 2H, Ph), 5.48 (d, J = 2.6 Hz, 2H, C8-H), 3.95 (d, J = 13.8 Hz, 1H, C6-H), 3.78 (d, J = 13.8 Hz, 1H, C6-H), 3.60 (s, 3H, OMe), 3.27 (dd, J = 8.7, 6.1 Hz, 1H, C2-H), 3.09 (m, 1H, C5-H), 2.48 (dt, J = 8.4, 8.2 Hz, 1H, C5-H), 2.18–1.66 (m, 4H, C3-H and C4-H). 13C NMR (75 MHz, CDCl3): δ 174.6 (COO), 145.1 (C6'), 134.8 (Ph, C), 129.2 (Ph, CH), 128.8 (Ph, CH), 128.3 (Ph, CH), 122.8 (C7), 64.9 (C2), 54.2 (C5), 53.5 (C8), 51.9 (OCH3), 48.9 (C6), 29.5 (C3), 23.2 (C4). IR (film): υmax 3493, 3140, 2951, 2850, 2359, 1745, 1732, 1556, 1496, 1454, 1359, 1284, 1049, 769, 725 cm−1. ESI-HRMS m/z [M+Na]+ calcd for C16H20N4NaO2 323.1478, found 323.1474.
Methyl ((1-Cyclohexyl-1H-1,2,3-Triazol-4-yl)Methyl)-L-Prolinate (3d)
Compound 3d was prepared from 45 mg (0.27 mmol) of N-propargyl methyl prolinate 1, following the general procedure for the 1,3-dipolar cycloaddition, affording 31 mg of a yellowish oil in 39% yield. 1H NMR (300 MHz, CDCl3): δ 7.51 (s, 1H, C7-H), 4.44 (d, J = 2.6 Hz, 2H, C8-H), 3.97 (d, J = 13.8 Hz, 1H, C6-H), 3.79 (d, J = 13.8 Hz, 1H, C6-H), 3.66 (s, 3H, OMe), 3.29 (dd, J = 8.7, 6.1 Hz, 1H, C2-H), 3.16–3.10 (m, 1H, C5-H), 2.46 (dt, J = 8.4, 8.2 Hz, 1H, C5-H), 2.20–1.63 (m, 12H, C3-H and cyclohexyl), 1.51–1.21 (m, 3H, C4-H and cyclohexyl). 13C NMR (75 MHz, CDCl3): δ 174.5 (COO), 144.0 (C6'), 120.3 (C7), 64.8 (C2), 59.7 (C5), 53.4 (C8), 51.85 (OCH3), 48.9 (C6), 33.5 (CH2), 32.4 (C3), 29.4 (CH2), 25.2 (CH2), 23.0 (C4). IR (film): υmax 3417, 3142, 2935, 2856, 2362, 1737, 1732, 1633, 1450, 1371, 1276, 1201, 1049, 997, 894, 823, 777 cm−1. ESI-HRMS m/z [M+H]+ calcd for C15H25N4O2 293.1972, found 293.1970.
Methyl ((1-(3-Phenylpropyl)-1H-1,2,3-Triazol-4-yl)Methyl)-L-Prolinate (3e)
Compound 3e was prepared from 45 mg (0.27 mmol) of N-propargyl methyl prolinate 1, following the general procedure for the 1,3-dipolar cycloaddition, affording 73 mg of a yellowish oil in 82% yield. 1H NMR (300 MHz, CDCl3): δ 7.43 (s, 1H, C7-H), 7.24–7.19 (m, 2H, Ph), 7.18–7.08 (m, 3H, Ph), 4.21 (d, J = 2.6 Hz, 2H, C8-H), 3.93 (d, J = 13.8 Hz, 1H, C6-H), 3.76 (d, J = 13.8 Hz, 1H, C6-H), 3.60 (s, 3H, OMe), 3.24 (dd, J = 8.7, 6.1 Hz, 1H, C2-H), 3.06 (m, 1H, C5-H), 2.53–2.44 (m, 5H), 2.23–1.61 (m, 4H, C3-H and C4-H). 13C NMR (75 MHz, CDCl3): δ 174.5 (COO), 144.6 (C6'), 140.3 (Ph), 128.7 (Ph), 128.5 (Ph), 126.4 (Ph), 122.8 (C7), 64.7 (C2), 53.4 (C5), 51.9 (OCH3), 49.5 (C8), 48.7 (C6), 32.5 (CH2), 31.7 (C3), 29.5 (CH2), 23.5 (C4). IR (film): υmax 3626, 3458, 3138, 2949, 2854, 2358, 1732, 1602, 1496, 1444, 1354, 1278, 1172, 1085, 1049, 1004, 785, 748, 702 cm−1. ESI-HRMS m/z [M+Na]+ calcd for C18H24N4NaO2 351.1791, found 351.1790.
Methyl ((1-Cinnamyl-1H-1,2,3-Triazol-4-yl)Methyl)-L-Prolinate (3f)
Compound 3f was prepared from 45 mg (0.27 mmol) of N-propargyl methyl prolinate 1, following the general procedure for the 1,3-dipolar cycloaddition, affording 53 mg of a yellowish oil in 60% yield. 1H NMR (300 MHz, CDCl3): δ 7.58 (s, 1H, C7-H), 7.43–7.21 (m, 5H, Ph), 6.65 (d, J = 15.8 Hz, 1H, C9-H), 6.33 (m, 1H, C10-H), 5.11 (d, J = 2.6 Hz, 2H, C8-H), 4.00 (d, J = 13.8 Hz, 1H, C6-H), 3.82 (d, J = 13.8 Hz, 1H, C6-H), 3.66 (s, 3H, OMe), 3.31 (dd, J = 8.7, 6.1 Hz, 1H, C2-H), 3.14 (m, 1H, C5-H), 2.52 (dt, J = 8.4, 8.2 Hz, 1H, C5-H), 2.20–2.04 (m, 1H, C3-H), 2.00–1.71 (m, 3H, C3-H, C4-H2). 13C NMR (75 MHz, CDCl3): δ 174.5 (COO), 144.9 (C6'), 135.3 (Ph), 135.3 (C9-H), 128.7 (Ph), 128.5 (CH), 126.7 (Ph), 122.5 (C10-H), 121.9 (C7), 64.7 (C2), 53.4 (C5), 52.3 (OCH3), 51.8 (C8), 48.7 (C6), 29.4 (C3), 23.0 (C4). IR (film): υmax 3541, 3138, 2951, 2845, 2358, 1741, 1732, 1552, 1448, 1359, 1278, 1203, 1174, 1128, 1047, 970, 756, 694 cm−1. ESI-HRMS m/z [M+Na]+ calcd for C18H22N4NaO2 349.1635, found 349.1625.
Methyl ((1-(Naphthalen-2-Ylmethyl)-1H-1,2,3-Triazol-4-yl)Methyl)-L-Prolinate (3g)
Compound 3g was prepared from 20 mg (0.12 mmol) of N-propargyl methyl prolinate 1, following the general procedure for the 1,3-dipolar cycloaddition, affording 34 mg of a light orange solid in 81% yield. M.p. 64.0-64.9 °C. 1H NMR (300 MHz, CDCl3): δ 7.85–7.82 (m, 3H, naphtyl), 7.74 (s, 1H, C-H), 7.52–7.49 (m, 2H, naphtyl), 7.46 (s, 1H, C7-H), 7.37–7.33 (m, 1H, naphtyl), 5.66 (d, J = 3.6 Hz, 2H, C8-H), 3.97 (d, J = 13.9 Hz, 1H, C6-H), 3.80 (d, J = 13.9 Hz, 1H, C6-H), 3.60 (s, 3H, OMe), 3.26 (dd, J = 8.7, 6.1 Hz, 1H, C2-H), 3.06 (m, 1H, C5-H), 2.46 (dt, J = 8.4, 8.2 Hz, 1H, C5-H), 2.15–1.65 (m, 4H, C3-H and C4-H). 13C NMR (75 MHz, CDCl3): δ 174.4 (COO), 145.1 (C6'), 133.2 (C), 133.1 (C), 131.9 (C), 129.1 (CH), 127.9 (CH), 127.7 (CH), 127.4 (CH), 126.7 (CH), 125.3 (C7), 122.7 (CH), 122.7 (CH), 64.7 (C2), 54.3 (C5), 53.4 (C8), 51.8 (OCH3), 43.7 (C6), 29.4 (C3), 23.0 (C4). IR (KBr): υmax 3500, 3132, 2949, 2818, 2358, 1732, 1600, 1548, 1508, 1435, 1338, 1273, 1203, 1172, 1126, 1047, 891, 771 cm−1. ESI-HRMS m/z [M+H]+ calcd for C20H23N4O2 351.1815, found 351.1826.
Methyl ((1-Octyl-1H-1,2,3-Triazol-4-yl)Methyl)-L-Prolinate (3h)
Compound 3h was prepared from 20 mg (0.12 mmol) of N-propargyl methyl prolinate 1, following the general procedure for the 1,3-dipolar cycloaddition, affording 31 mg of a yellow oil in 81% yield. 1H NMR (300 MHz, CDCl3): δ 7.44 (s, 1H, C7-H), 4.24 (t, J = 7.2 Hz, 2H, C8-H), 3.93 (d, J = 13.8 Hz, 1H, C6-H), 3.75 (d, J = 13.8 Hz, 1H, C6-H), 3.61 (s, 3H, OMe), 3.23 (dd, J = 8.6, 6.0 Hz, 1H, C2-H), 3.08–3.02 (m, 1H, C5-H), 2.49–2.41 (dt, 1H, J = 8.4, 8.1 Hz, C5-H), 2.03–1.71 (m, 5H), 1.22–1.17 (m, 11H), 0.79 (t, J= 6.5 Hz, 3H, CH3). 13C NMR (75 MHz, CDCl3): δ 174.4 (COO), 144.4 (C6'), 122.5 (C7), 64.6 (C2), 53.2 (C5), 51.8 (OCH3), 50.2 (C8), 48.6 (C6), 31.6 (CH2), 30.2 CH2), 29.4 (C3), 29.0 (CH2), 28.9 (CH2), 26.4 (CH2), 23.0 (C4), 22.5 (CH2), 14.0 (CH3). IR (film): υmax 3604, 3458, 3136, 2926, 2357, 1345, 1645, 1444, 1354, 1172, 1047, 771 cm−1. ESI-HRMS m/z [M+Na]+ calcd for C17H30N4NaO2 345.2261, found 345.2261.
Methyl ((1-Decyl-1H-1,2,3-Triazol-4-yl)Methyl)-L-Prolinate (3i)
Compound 3i was prepared from 20 mg (0.12 mmol) of N-propargyl methyl prolinate 1, following the general procedure for the 1,3-dipolar cycloaddition, affording 32 mg of a yellow oil in 75% yield. 1H NMR (300 MHz, CDCl3): δ 7.49 (s, 1H, C7-H), 4.32 (t, J = 7.2 Hz, 2H, C8-H), 4.02 (d, J = 13.8 Hz, 1H, C6-H), 3.83 (d, J = 13.8 Hz, 1H, C6-H), 3.66 (s, 3H, OMe), 3.33 (dd, J = 8.7, 6.1 Hz, 1H, C2-H), 3.17–3.08 (m, 1H, C5-H), 2.58–2.50 (dt, J = 8.4, 8.2 Hz, 1H, C5-H), 2.11–1.77 (m, 4H, C3-H and C4-H), 1.27–1.21 (m, 16H), 0.84 (t, J = 6.6 Hz, 3H, CH3). 13C NMR (75 MHz, CDCl3): δ 174.5 (COO), 144.4 (C6'), 122.7 (C7), 64.7 (C2), 53.4 (C5), 51.9 (OCH3), 50.4 (C8), 48.7 (C6), 31.9 (CH2), 30.4 (CH2), 29.5 (C3), 29.5 (CH2), 29.3 (CH2), 29.1 (CH2), 29.1 (CH2), 26.5 (CH2), 23.1 (C4), 22.7 (CH2), 14.2 (CH3). IR (film): υmax 3458, 2926, 2854, 2358, 1745, 1732, 1651, 1444, 1373, 1278, 1199, 1172, 1047, 891, 783, 721 cm−1. ESI-HRMS m/z [M+H]+ calcd for C19H35N4O2 351.2754, found 351.2746.
Methyl ((1-Hexadecyl-1H-1,2,3-Triazol-4-yl)Methyl)-L-Prolinate (3j)
Compound 3j was prepared from 20 mg (0.12 mmol) of N-propargyl methyl prolinate 1, following the general procedure for the 1,3-dipolar cycloaddition, affording 22 mg of a white solid in 42 % yield. M.p. 60-60.9 °C. 1H NMR (300 MHz, CDCl3): δ 7.47 (s, 1H, C7-H), 4.29 (t, J = 7.2 Hz, 2H, C8-H), 3.99 (d, J = 13.8 Hz, 1H, C6-H), 3.80 (d, J = 13.8 Hz, 1H, C6-H), 3.67 (s, 3H, OMe), 3.30 (dd, J = 8.7, 6.1 Hz, 1H, C2-H), 3.16–3.11 (m, 1H, C5-H), 2.57–2.48 (dt, J = 8.9, 7.8 Hz, 1H, C5- H), 1.91–1.84 (m, 4H), 1.28–1.23 (m, 28H), 0.85 (t, J = 6.6 Hz, 3H, CH3). 13C NMR (75 MHz, CDCl3): δ 174.7 (COO), 144.7 (C6'), 122.6 (C7), 64.6 (C2), 53.2 (C5), 52.0 (OCH3), 50.5 (C8), 48.9 (C6), 32.1 (CH2), 30.5 (CH2), 29.9 (CH2), 29.8 (CH2), 29.8 (CH2), 29.7 (CH2), 29.6 (CH2), 29.6 (CH2), 29.6 (CH2), 29.4 (C3), 29.3 (CH2), 29.2 (CH2), 28.9 (CH2), 26.4 (CH2), 23.0 (C4), 22.6 (CH2), 14.0 (CH3). IR (KBr): υmax 3124, 2914, 2357, 1745, 1728, 1556, 1444, 1336, 1269, 1197, 1053, 848, 790, 719 cm−1. ESI-HRMS m/z [M+Na]+ calcd for C25H46N4NaO2 457.3513, found 457.3512.
Methyl (Z)-((1-(Octadec-9-en-1-yl)-1H-1,2,3-Triazol-4-yl)Methyl)-L-Prolinate (3k)
Compound 3k was prepared from 20 mg (0.12 mmol) of N-propargyl methyl prolinate 1, following the general procedure for the 1,3-dipolar cycloaddition, affording 33 mg of a light-yellow oil in 59 % yield. 1H NMR (300 MHz, CDCl3): δ 7.49 (s, 1H, C7-H), 5.35–5.31 (m, CH=CH, 2H), 4.30 (t, J = 7.2 Hz, 2H, C8-H), 4.00 (d, J = 13.8 Hz, 1H, C6-H), 3.81 (d, J = 13.8 Hz, 1H, C6-H), 3.68 (s, 3H, OMe), 3.30 (dd, J = 8.8, 6.1 Hz, 1H, C2-H), 3.15–3.10 (m, 1H, C5-H), 2.56–2.48 (dt, J = 8.5, 8.1 Hz, 1H, C5-H), 2.16–1.79 (m, 6H), 1.27–1.25 (m, 26H), 0.87 (t, J = 6.7 Hz, 3H, CH3). 13C NMR (75 MHz, CDCl3): δ 174.4 (COO), 144.4 (C6'), 130.0 (CH=), 129.7 (CH=), 122.5 (C7), 64.7 (C2), 53.3 (C5), 51.8 (OCH3), 50.3 (C8), 48.6 (C6), 31.9 (CH2), 30.3 (CH2), 29.7 (CH2), 29.7 (CH2), 29.5 (C3), 29.4 (CH2), 29.4 (CH2), 29.3 (CH2), 29.1 (CH2), 29.0 (CH2), 29.0 (CH2), 27.2 (CH2), 27.1 (CH2), 26.5 (CH2), 23.0 (C4), 22.6 (CH2), 14.1 (CH3). IR (film): υmax 3564, 3477, 3136, 2924, 2852, 2358, 2096, 1745, 1556, 1444, 1373, 1276, 1199, 1172, 1047, 968, 891, 775, 723 cm−1. ESI-HRMS m/z [M+K]+ calcd for C27H48 KN4O2 499.3408, found 499.3412.
Methyl ((1-Icosyl-1H-1,2,3-Triazol-4-yl)Methyl)-L-Prolinate (3l)
Compound 3l was prepared from 20 mg (0.12 mmol) of N-propargyl methyl prolinate 1, following the general procedure for the 1,3-dipolar cycloaddition, affording 34 mg of a white solid in 58 % yield. M.p. 72.7–73.7 °C. 1H NMR (300 MHz, CDCl3): δ 7.49 (s, 1H, C7-H), 4.31 (t, J = 7.2 Hz, 2H, C8-H), 4.01 (d, J = 13.8 Hz, 1H, C6-H), 3.82 (d, J = 13.8 Hz, 1H, C6-H), 3.69 (s, 3H, OMe), 3.30 (dd, J = 8.5, 5.7 Hz, 1H, C2-H), 3.14–3.1 (m, 1H, C5-H), 2.54–2.46 (dt, J = 8.5, 7.9 Hz, 1H, C5- H), 1.91–1.84 (m, 4H), 1.28–1.23 (m, 36H), 0.87 (t, J = 6.7 Hz, 3H, CH3). 13C NMR (75 MHz, CDCl3): δ 174.5 (COO), 144.4 (C6'), 122.4 (C7), 64.7 (C2), 53.3 (C5), 51.8 (OMe), 50.2 (C8), 48.6 (CH2), 31.9 (CH2), 30.2 (CH2), 29.6 (CH2), 29.5 (CH2), 29.3 (CH2), 29.0 (CH2), 26.4 (CH2), 23.0 (CH2), 22.6 (CH2), 14.1 (CH3). IR (KBr): υmax 3649, 3124, 3076, 2916, 2846, 2358, 1743, 1462, 1338, 1271, 1211, 1055, 1037, 852, 771, 719 cm−1. ESI-HRMS m/z [M+Na]+ calcd for C29H54NaN4O2 513.4139, found 513.4125.
Methyl ((1-(3,7-Dimethylocta-2,6-Dien-1-yl)-1H-1,2,3-Triazol-4-yl)Methyl)-L-Prolinate (3m)
Compound 3m was prepared from 30 mg (0.18 mmol) of N-propargyl methyl prolinate 1, following the general procedure for the 1,3-dipolar cycloaddition, affording 47 mg of a yellow oil in 87 % yield. 1H NMR (300 MHz, CDCl3): δ 7.46 (s, 1H, C7-H), 5.41 (t, J = 7.2 Hz, 1H, C9-H), 5.08–5.01 (m, 1H, C13-H), 4.90 (t, J = 7.2 Hz, 2H, C8-H), 4.01 (d, J = 13.6 Hz, 1H, C6-H), 3.82 (d, J = 13.6 Hz, 1H, C6-H), 3.66 (s, 3H, OMe), 3.30 (dd, J = 8.1, 6.0 Hz, 1H, C2-H), 3.12–3.1 (m, 1H, C5-H), 2.54–2.46 (dt, J = 8.4, 8.2 Hz, 2H, C5-H), 1.7 (m, 6H, C3-H and C4-H), 1.65 (s, 3H, CH3), 1.63 (m, 6H, CH3), 1.55 (m, 3H, CH3). 13C NMR (75 MHz, CDCl3): δ 174.5 (COO), 144.4 (C6'), 122.7 (C7), 64.7 (C2), 53.4 (C5), 51.9 (OCH3), 50.4 (C8), 48.7 (C6), 31.9 CH2), 30.4 (CH2), 29.5 (C3), 29.3 (CH2), 29.1 (CH2), 26.5 (CH2), 23.1 (C4), 22.7 (CH2), 17.6 (CH3), 16.5 (CH3), 16.0 (CH3). IR (film): υmax 3564, 3140, 2926, 2358, 1867, 1747, 1732, 1506, 1435, 1373, 1217, 1174, 1124, 1047, 844, 771 cm−1. ESI-HRMS m/z [M+H]+ calcd for C19H35N4O2 351.2754, found 351.2746.
Methyl ((1-((2E,6E)-3,7,11-Trimethyldodeca-2,6,10-Trien-1-yl)-1H-1,2,3-Triazol-4-yl)Methyl)-L-Prolinate (3n) and Methyl ((1-((2E,6Z)-3,7,11-Trimethyldodeca-2,6,10-Trien-1-yl)-1H-1,2,3-Triazol-4-yl)Methyl)-L-Prolinate (3o)
Compound 3n and 3o were prepared from 50 mg (0.30 mmol) of N-propargyl methyl prolinate 1, following the general procedure for the 1,3-dipolar cycloaddition, affording 53 mg of 3n and 28 mg of 3o as yellowish oils in 43 % and 23% yield, respectively.
3n-1H NMR (300 MHz, CDCl3): δ 7.47 (s, 1H, C7-H), 5.42 (t, J = 7.2 Hz, 1H, C9-H), 5.08–5.06 (m, 2H, C13-H and C16-H), 4.95 (t, J = 7.2 Hz, 2H, C8-H), 4.00 (d, J = 13.7 Hz, 1H, C6-H), 3.80 (d, J = 13.7 Hz, 1H, C6-H), 3.69 (s, 3H, OMe), 3.31 (dd, J = 8.7, 6.1 Hz, 1H, C2-H), 3.16–3.10 (m, 1H,C5-H), 2.61–2.48 (dt, J = 8.4, 8.2 Hz, 1H, C5-H), 1.70 (m, 4H, C3-H and C4-H), 2.12–1.91 (m, 8H), 1.78 (s, 3H, CH3), 1.65 (s, 3H, CH3), 1.63 (m, 3H, CH3), 1.55 (m, 3H, CH3). 13C NMR (75 MHz, CDCl3): δ 174.4 (COO), 144.4 (C6'), 143.1 (C), 135.7 (C), 131.3 (C), 124.2 (CH), 123.3 (CH), 122.0 (C7-H), 116.9 (CH), 64.7 (C2), 53.2 (C5), 51.8 (OCH3), 48.6 (C8), 47.8 (C6), 39.6 (CH2), 39.4 (CH2), 29.3 (C3), 26.6 (CH2), 26.1 (CH2), 25.6 (CH2), 22.9 (CH3), 17.6 (CH3), 16.5 (CH3), 16.0 (CH3). IR (film): υmax 3417, 3124, 2992, 2358, 1867, 1747, 1732, 1539, 1456, 1317, 1271, 1122, 1047, 773 cm−1. ESI-HRMS m/z [M+H]+ calcd for C24H39N4O2 415.3067, found 415.3067.
3o-1H NMR (300 MHz, CDCl3): δ 7.47 (s, 1H, C7-H), 5.42 (t, J = 7.2 Hz, 1H, C9-H), 5.08–5.06 (m, 2H, C13-H and C16-H), 4.95 (t, J = 7.2 Hz, 2H, C8-H), 4.00 (d, J = 13.7 Hz, 1H, C6-H), 3.80 (d, J = 13.7 Hz, 1H, C6-H), 3.69 (s, 3H, OMe), 3.31 (dd, J = 8.7, 6.1 Hz, 1H, C2-H), 3.16–3.10 (m, 1H, C5-H), 2.61–2.48 (dt, J = 8.4, 8.2 Hz, 1H, C5-H), 1.7 (m, 4H, C3-H and C4-H), 2.12–1.91 (m, 8H), 1.78 (s, 3H, CH3), 1.65 (s, 3H, CH3), 1.63 (m, 3H, CH3), 1.55 (m, 3H, CH3). 13C NMR (75 MHz, CDCl3): δ 174.4 (COO), 144.4 (C6'), 143.1 (C), 135.7 (C), 131.3 (C), 124.2 (CH), 123.3 (CH), 122.0 (C7-H), 116.9 (CH), 64.7 (C2), 53.2 (C5), 51.8 (OCH3), 48.6 (C8), 47.8 (C6), 39.6 (CH2), 39.4 (CH2), 29.3 (C3), 26.6 (CH2), 26.1 (CH2), 25.6 (CH2), 22.9 (CH3), 17.6 (CH3), 16.5 (CH3), 16.0 (CH3). IR (film): υmax 3417, 3124, 2962, 2924, 2341, 1745, 1732, 1625, 1446, 1435, 1377, 1215, 1172, 1047, 775 cm−1. ESI-HRMS m/z [M+H]+ calcd for C24H39N4O2 415.3067, found 415.3067.
Methyl ((1-((E)-3,7,11,15-Tetramethylhexadec-2-en-1-yl)-1H-1,2,3-Triazol-4-yl)Methyl) -L-Prolinate (3p) and Methyl ((1-(3,7,11,15-Tetramethylhexadec-2-en-1-yl)-1H-1,2,3-Triazol-4-yl)Methyl)-L-Prolinate (3q)
Compound 3p and 3q were prepared from 50 mg (0.30 mmol) of N-propargyl methyl prolinate 1, following the general procedure for the 1,3-dipolar cycloaddition, affording 22 mg of 3p (E-isomer) and 23 mg of 3q (mixture E:Z) as yellowish oils in 38 % and 39 % yield, respectively.
3p-1H NMR (300 MHz, CDCl3): δ 7.47 (s, 1H, C7-H), 5.39 (t, J = 7.2 Hz, 1H, C9-H), 4.91 (t, J = 7.2 Hz, 2H, C8-H), 3.98 (d, J = 13.7 Hz, 1H, C6-H), 3.78 (d, J = 13.7 Hz, 1H, C6-H), 3.68 (s, 3H, OMe), 3.29 (dd, J = 8.7, 6.1 Hz, 1H, C2-H), 3.14–3.09 (m, 1H, C5-H), 2.54–2.46 (dt, J = 8.4, 8.2 Hz, 1H, C5-H), 2.11 (s, 3H, C10-CH3), 2.02–1.84 (m, 4H, C3-H and C4-H), 1.77–1.75 (m, 3H), 1.36–1.06 (m, 18H), 0.86–0.81 (m, 12H, CH3). 13C NMR (75 MHz, CDCl3): δ 174.4 (COO), 144.4 (C6'), 143.7 (C10), 122.1 (C7), 117.4 (C9), 64.8 (C2), 53.7 (C5), 51.9 (OCH3), 48.7 (C6), 39.8 (C8), 39.4 (CH2), 37.4 (CH2), 37.3 (CH2), 37.0 (CH2), 36.9 (CH2), 36.7 (CH2), 32.7 (CH2), 32.3 (CH2), 29.7 (C3), 29.4 (C4), 28.0 (CH2), 25.5 (CH2), 25.0 (CH2), 24.8 (CH2), 24.5 (CH3), 23.4 (CH3), 23.0 (CH3), 22.7 (CH3), 22.6 (CH3). IR (film): υmax 3500, 3140, 2926, 2358, 1747, 1506, 1456, 1377, 1172, 1047, 933, 862, 775 cm−1. ESI-HRMS: mass calculated for C29H52N4NaO2 (M+Na)+, 511.3982, found 511.3970.
3q-1H NMR (300 MHz, CDCl3): δ 7.47 (s, 1H, C7-H), 5.39 (t, J = 7.2 Hz, 1H, CH), 4.91 (t, J = 7.2 Hz, 2H, C8-H), 3.98 (d, J = 13.7 Hz, 1H, C6-H), 3.78 (d, J = 13.7 Hz, 1H, C6-H), 3.68 (s, 3H, OMe), 3.29 (dd, J = 8.7, 6.1 Hz, 1H, C2-H), 3.14–3.09 (m, 1H, C5-H), 2.54–2.46 (dt, J = 8.4 Hz, J = 8.2 Hz, 1H, C5-H), 2.11 (s, 3H, CH3), 2.02–1.84 (m, 4H, C3-H and C4-H), 1.77–1.75 (m, 3H), 1.36–1.06 (m, 18H), 0.86–0.81 (m, 12H, CH3). 13C NMR (75 MHz, CDCl3): δ 174.4 (COO), 144.4 (C6'), 143.7 (C10), 122.1 (C7), 117.4 (C9), 64.8 (C2), 53.7 (C5), 51.9 (OCH3), 48.7 (C6), 39.8 (C8), 39.4 (CH2), 37.4 (CH2), 37.3 (CH2), 37.0 (CH2), 36.9 (CH2), 36.7 (CH2), 32.7 (CH2), 32.3 (CH2), 29.7 (C3), 29.4 (C4), 28.0 (CH2), 25.5 (CH2), 25.0 (CH2), 24.8 (CH2), 24.5 (CH3), 23.4 (CH3), 23.0 (CH3), 22.7 (CH3), 22.6 (CH3). IR (film): υmax 3500, 3140, 2926, 2358, 1747, 1506, 1456, 1377, 1172, 1047, 933, 862, 775 cm−1. ESI-HRMS m/z [M+Na]+ calcd for C29H52N4NaO2 511.3982, found 511.3970.
Biology
Reagents
All reagents were purchased from Sigma-Aldrich (St. Louis, MO, USA). Culture medium and fetal calf serum (FCS) were purchased from Cultilab (Campinas, SP, Brazil).
Cells and Parasites
T. cruzi CL strain clone 14 epimastigotes (Brener and Chiari, 1965) were maintained in the exponential growth phase by subculturing every 48 h in Liver Infusion Tryptose (LIT) medium supplemented with 10% FCS (Vitrocell, Campinas, São Paulo, Brazil) at 28 °C.
Growth Inhibition Assays
T. cruzi epimastigotes in the exponential growth phase (5.0-6.0 x 107 cells mL−1) were cultured in fresh LIT medium. The cells were treated with different concentrations of drugs or not treated (negative control). A combination of Rotenone (60 μM) and Antimycin (0.5 μM) was used as a positive control for inhibition as previously described. (Magdaleno et al., 2009) The cells (2.5 x 106 mL−1) were transferred to 96-well culture plates and incubated at 28 °C. Cell proliferation was quantified by reading the optical density (OD) at 620 nm for 8 days. The OD was converted to cell density values (cells per mL) using a linear regression equation previously obtained under the same conditions. The concentration of compounds that inhibited 50% of parasite proliferation (IC50) was determined during the exponential growth phase (4 days) by fitting the data to a typical sigmoidal dose-response curve using OriginPro8. The compounds were evaluated in quadruplicate in each experiment. The results shown here correspond to three independent experiments. As a cell growth inhibition control, growth curves in which 200 mM rotenone and 0.5 mM antimycin were added to the culture medium were run in parallel for all experiments.
Cytotoxicity Assay
To evaluate the analogs toxicity, Vero cells previously plated on 96 multi-well plate in DMEM 2% FBS and incubated for 48 h at 37 °C in a humid atmosphere containing 5% CO2, were incubated with 700 μL of DMEM 2% FBS supplemented with each analog for 48 h. The concentration (μM) was different with each analog:
◦ 3i: 20 μM, 40 μM, 60 μM, 80 μM, 100 μM and 120 μM.
◦ 3k: 5 μM, 10 μM, 15 μM, 20 μM, 25 μM and 30 μM.
◦ 3n: 5 μM, 10 μM, 15 μM, 20 μM, 25 μM and 30 μM.
◦ DMSO: 5 μM, 25 μM, 50 μM and 100 μM.
◦ Benznidazole: 10 μM, 100 μM, 200 μM and 300 μM.
The viability of cells was measured by MTT dye (3-(4,5-Dimethylthiazol-2-yl)-2,5-diphenyltratazolium bromide, Sigma-Aldrich) colorimetric method. Benznidazole and DMSO were used as positive and negative controls, respectively. Data are expressed as means ± SD of the results of three independent assays of each condition.
L-Proline Transport Inhibition Assay
Parasites in exponential growth phase were washed three times by centrifugation and resuspended in phosphate buffered saline (PBS), pH 7.4. Cells were counted in a Neubauer chamber, adjusted with the same buffer to a final density of 200 × 106 cells/mL and distributed in aliquots of 100 μL (containing 20 × 106 cells each). Transport assays were initiated by the addition to the assay tubes of 100 μL of the desired dilution of L-proline in PBS in the presence of 0.5 mCi of L-[3H] proline. Unless otherwise specified, V0 was measured at 28 °C for 30 s by incorporation of 0.75 and 3 mM L-proline traced with 1 μCi of U-14C-L-Pro (Perkin Elmer). In all cases, proline transport was stopped by addition of 800 mL of cold 50 mM proline in PBS (pH 7.4), and rapid washing by centrifugation at 10,000x g for 2 min. Background values in each experiment were measured by simultaneous addition of radiolabeled L-proline and stop solution as previously described (Silber et al., 2002).
Competition Assay
For the competition assays, the transport experiments were performed as described above using the L-Pro concentration corresponding to the Km (0.31 mM). Those conditions were chosen considering an inhibitory activity by structurally related compounds should be competitive, and so, should be evidenced by a change in the Vmax at L-Pro concentrations close to the Km. Non-competitive inhibitors, if any, should diminish Vmax, which is measured at 10 x Km L-Pro (3.1 mM) (Silber et al., 2002).
Statistical Analysis
One-way ANOVA followed by the Tukey test was used for statistical analysis. The T-test was used to analyze differences between groups. P < 0.05 was considered statistically significant.
Results
Synthesis of L-Proline Transport Inhibitors
A proper uptake blocker needs to be recognized by the transporter, but not being able to go through, by adding a bulky substituent or a membrane interacting portion that prevents its transport (Figure 1).
This model will require a compound holding a recognition motif, a linker and a membrane anchor or a voluminous substituent to block the L-proline incorporation. The recognition portion will have L-proline to specifically interact with the transporter, the membrane anchor should be a non-polar group and both parts will be connected by a 1,2,3-triazole (Figure 2).
To validate the proposed model, we proceeded to make a small library following the synthetic strategy shown on Scheme 1. The synthesis started from commercial L-proline, preparing the key intermediate in two steps, including an esterification to produce the methyl prolinate 1 followed by an N-alkylation with propargyl bromide. Once the required key propargyl methyl prolinate 2 intermediate was prepared, a pool of different azides covering a variety of steric moieties including, aryl, alkyl and isoprenyl substituents was selected to explore their capacity to interact with the membrane. Azides were prepared by direct substitution of bromide with sodium azide on DMF except for geranyl-, farnesyl-, and phytylazides, which were synthesized from geraniol, farnesol and phytol, respectively, using diphenylphosphorylazide (DPPA) following Thompson's procedure (Thompson et al., 1993). Phytylazide was found to be a mixture of three chemical entities in equilibrium: tertiary azide, E and Z isomers of the primary azide, following the same behavior previously observed on geranyl-, and farnesylazide (Porta et al., 2014). Allylic azides can be obtained as a mixture, because they exist as equilibrating mixtures of regioisomers due to the [3,3] sigmatropic rearrangement (Winstein rearrangement) (Gagneuz et al., 1969). That was the case of geranyl, farnesyl and phytylazide, a mixture of primary:tertiary (8:1), being the primary as 1.6:1 (E:Z) ratio (Porta et al., 2017b).
Having prepared the pool of azides, we continued with the synthesis of a focused library of inhibitors through click chemistry. Reactions were conducted in a parallel solution synthesis setup under copper (II) sulfate catalytic conditions in water:t-BuOH (1:1), using sodium ascorbate as a reductant (Rostovtsev et al., 2002; Labadie et al., 2011; Porta et al., 2017a). In general, reactions needed an excess of azides for completion and a reaction time was 18 h. All the products have 1,4-substitution on the 1,2,3,-triazol as expected, based on the original description of this methodology and our previous work (Porta et al., 2014, 2017a). Reactions with aliphatic and benzylic azides produced a single product, with yields that are slightly better for the last ones. The reaction with the mixture of geranyl azides generated 3i, which was identified as an inseparable mixture of E and Z isomers (1H NMR, 1.5:1), in accordance with our previous results (Porta et al., 2017a). When farnesyl azide was used, a mixture of regioisomers was also obtained with the same ratio, but in this case they were separable (Figure 3). When phytylazide was used the same regioisomers were isolated after the reaction with N-propargyl methyl prolinate 1, presenting a higher E:Z ratio (1.8:1). Extensive purification work allowed only the isolation of the E-isomer of a pure compound (3p-E, Figure 3), leaving the remaining product 3q as a mixture E:Z.
Final products 3a-3q, presented in Table 1, were obtained with an average 67 % yield after purification and were completely characterized by 1D and 2D NMR and ESI-HRMS.
In order to evaluate the biological activity of the prepared collection we decided to initially determine the activity on T. cruzi epimastigotes. Then, to validate the L-proline transporter as the molecular target, the intracellular concentration of the amino acid was measured in competition assays with compounds that shown the best antiparasitic activity. Finally, the cytotoxicity of the selected candidates was evaluated in African green monkey kidney epithelial (VERO) cells to estimate the selectivity toward the parasite.
In vitro Activity Against T. cruzi Epimastigotes
The compounds collection was assayed against T. cruzi epimastigotes (CL strain clone 14) (Brener and Chiari, 1965) at a maximum concentration of 100 μM. Eight compounds of the total list did not affect the parasite growth at that concentration (3a, 3b, 3c, 3d, 3e, 3f, 3h, 3m), being considered inactive. (Table 1) The naphtyl derivative 3g and the eicosanyl analog 3l have an IC50 of 100 μM. A second group of active members of the collection includes the remaining prenyl derivatives 3n, 3o, 3p and 3p with IC50s 48.32, 58.60, 69.75, 48.27 μM, respectively. (Table 1) The remaining group contains the aliphatic derivatives 3i, 3j and 3k. Those compounds have IC50s below 40 μM, being the most active members the collection. The decyl and the oleyl derivatives 3i and 3k have similar activity (IC50 of 38.97 and 35.06 μM, respectively), while the cetyl analog 3j has a IC50 of 24.07 μM, considerably slower than the other two (Table 1).
In vitro Cytotoxicity Assay on Vero Cells
Vero cells are well established model to test cytotoxicity in vitro because it is an aneuploid and a continuous cell linage. Initially the library was screened at a fix concentration of 4.75 μg/mL and none of the analogs showed cytotoxic activity. The most active analogs of the series, compounds 3i, 3j, 3k, and 3n, were submitted to a further analysis to determine their IC50. Compound 3j was not soluble at 50 μM which made not possible to calculate its IC50. Analogs 3i, 3k, and 3n were soluble in a concentration range allowing to perform the assay, showing IC50 of 43 μM, 17 μM and 14 μM, respectively.
Proline Transport Assay
In order to obtain a deeper insight into the molecular mechanism of the most active compounds, we performed a proline transport competition assay. The compounds with an IC50 lower than 50 μM (3i, 3j, 3k, and 3n) were selected to perform the proline uptake assay aiming to determine the transport inhibition (Magdaleno et al., 2009). The analogs were assayed on T. cruzi epimastigotes incubated with proline at the transporter Km concentration (0.31 mM), and the analogs were assayed at concentration 10-fold higher (3.1 mM). Surprisingly, we observed that the compounds with higher activity on T. cruzi epimastigotes (lower IC50 values) showed inhibition but they were not strong enough when compared to analogs with lower antiparasitic activity (higher IC50 values). As can be seen on Figure 4, compounds 3i (T. cruzi epimastigotes IC50= 38 μM) and 3n (T. cruzi epimastigotes IC50= 49 μM) showed a proline transport inhibition higher than 75% being more active than the analog 3j that only produced an inhibition of 20%. The oleyl derivate (3k), with an unsaturated fatty tail, has a similar IC50 on T. cruzi epimastigotes compared to the cetyl analog (3j) showing no inhibition in terms of proline uptake (Table 2).
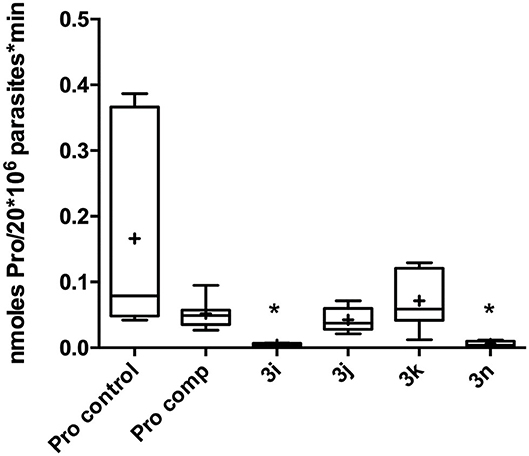
Figure 4. Proline uptake assay. Pro control = Proline control, Pro comp = L-Proline as competitor. 3i-k and 3n = proline level incubated (*p < 0.05) with selected analogs. Pro control (0.31 mM L-proline, [3H] proline, PBS). Pro Comp (3.1 mM L-proline, [3H] proline, PBS). Compounds 3i-k and 3n (0.31 mM L-proline, 3.1 mM analog 3x, [3H] proline, PBS). Stop solution: 50 mM L-proline added after 30 s.
Discussion
The selective inhibition of transporters has been proposed as a valuable target to develop new medications against different pathologies including neurological disorders (Qosa et al., 2016) and parasitic diseases (Sayé et al., 2019). The specific inhibition of neuronal glycine, alanine, serine, and cysteine transporters have been studied as molecular targets for new treatment of schizophrenia (Pinard et al., 2010; Schneider et al., 2012; Carland et al., 2014). A high-affinity transporter for proline has been identified, providing an important evidence for proline as a neurotransmitter (Hauptmann et al., 1983). A high throughput screening campaign for high affinity proline transporter inhibitors resulted in the identification of the selective inhibitor LP-403812 (Yu et al., 2009). Summarizing, the participation of metabolites uptake in critical processes in health and diseases has been well demonstrated.
A systematic design pipeline for molecules targeting molecular transporters has not been properly explored. The discovery of most of transporters inhibitors happened by chance or through large HTS campaigns. Burns et al. (2009) pioneered a work in this sense proposing a polyamine-fatty acid conjugate as a polyamine transporter inhibitor. In their rational, the polyamine portion is recognized by the transporter and the fatty acid interacts with the membrane, blocking the polyamine entrance. The newly designed inhibitors (L-Lyz(C18-Acyl-spermine) combined with DMFO display selective antitumoral activity. All the inhibitors mentioned before contained an amino acid portion, or an amino acid mimic, that is recognized by the transporter as a common feature (Figure 5).
With those precedents in mind, we proposed/presented here a general model for aminoacid transporter inhibitors. Our model included an aminoacid portion for recognition, linked to a variable region with different lipophilic and bulk substituents.
We identified the 1,2,3-triazole as a proper linker for our transport inhibitors. The neutral nature of this heterocycle has properly suited the requirements for bioconjugation, protein labeling and immobilization (Gauchet et al., 2006; Mckay and Finn, 2014) and for combining different pharmacophores to make hybrid compounds or chimeras. The Cu(I) azide-alkyne reaction, the quintessence of click chemistry, had a strong impact in many research areas including Medicinal Chemistry (Tron et al., 2008; Agalave et al., 2011). The easy reaction conditions has made this methodology very useful to rapidly prepare libraries of compounds, including antiparasitic drug candidates (Carvalho et al., 2010; Hamann et al., 2014; Porta et al., 2017a).
Using 1,2,3-triazole as linker, we prepared products introducing substituents with proper membrane anchor properties. Fatty acids and isoprenyl chains are selectively introduced in proteins by post translational modifications and served as a mediator for membrane association increasing their molecular hydrophobicity (Hannoush and Sun, 2010). Looking to produce similar anchoring properties, isoprenyl and linear long alkyl chain were selected as some of the 1,2,3-triazole substituents, introduced as azide on the heterocycle. Additionally, azides with bulky substituent were also used looking to obstruct the transporter. A library of 17 compounds was finally prepared including two alkyl ester, five alicyclic and aryl, five alkyl and five prenyl derivatives.
The proline uptake systems have similar biochemical characteristics in epimastigotes and the mammalian T. cruzi stages (Silber et al., 2002; Tonelli et al., 2004). It has been demonstrated that these stages are sensitive to proline availability (Tonelli et al., 2004) and uptake (Magdaleno et al., 2009). With those precedents in mind, we decided to evaluate the activity on epimastigotes. The results of the inhibition on T. cruzi epimastigotes shown that half of the compounds prepared did not show activity at the maximum concentration tested of 100 μM. Between the inactive analogs, 3a and 3b holds the shortest substituents of the library and have esters on the side-chain. Being the most polar substituent of the series and susceptible to hydrolysis, seems to be a possible explanation for the lack of activity. Analogs 3c, 3d, 3e, and 3f, contain non-polar cyclic substituents either arylic or alicyclic, were also inactive. Interestingly, the naphtyl derivative 3g, the bulkier member of the collection, has an IC50 of 100 μM. Together those results shown that polycyclic bulky substituents could be required to improve the activity. Nevertheless, the fact that the aromatic analogs were inactive discouraged the idea that π-stacking interactions are involved on the binding to the molecular target.
As was mentioned before, fatty acids and isoprenyl chains contribute as mediators for protein membrane anchoring, therefore, ten analogs were included with those substituents. The hypothesis that this kind of substituents contribute to improve the activity, seems to be validated because only the analogs holding the shorter substituents, 3h (R=octyl) and 3m (R=geranyl) were inactive. A detailed look at the aliphatic derivatives' activities did not show a direct correlation between the IC50 and the chain length. The activity increases from octyl 3i to decyl 3h derivative (IC50 > 100 and 38.27 mM, respectively), being the cetyl analog 3j, the most active (IC50 24.54 mM). Then, the activity decreases to 35.06 mM for the oleyl derivative 3k and 100 mM for the eicosanyl analog 3l. A similar behavior is observed for the prenyl derivatives, but in this case the difference is less pronounced. Moving from the geranyl derivative 3h to the farnesyl analogs the activity increase to 48.32 mM for the E-isomer 3n that is slightly more active than the Z-isomer 3o (59.60 mM). Then, as happened with the alkylated analogs, the activity decreases for the longer member of the family, the phytyl derivatives 3p (E-isomer) and 3q (E/Z mixture), with IC50s 69.75 and 48.27 mM, respectively.
A tendency can be visualized when the log IC50 was plotted against the carbon chain length and the aliphatic and the prenylated are separately correlated (Figure 6). One interesting outcome of this chart is that both curves have their minimum around 14 carbon atoms, that appears to be the optimal chain length for the activity.
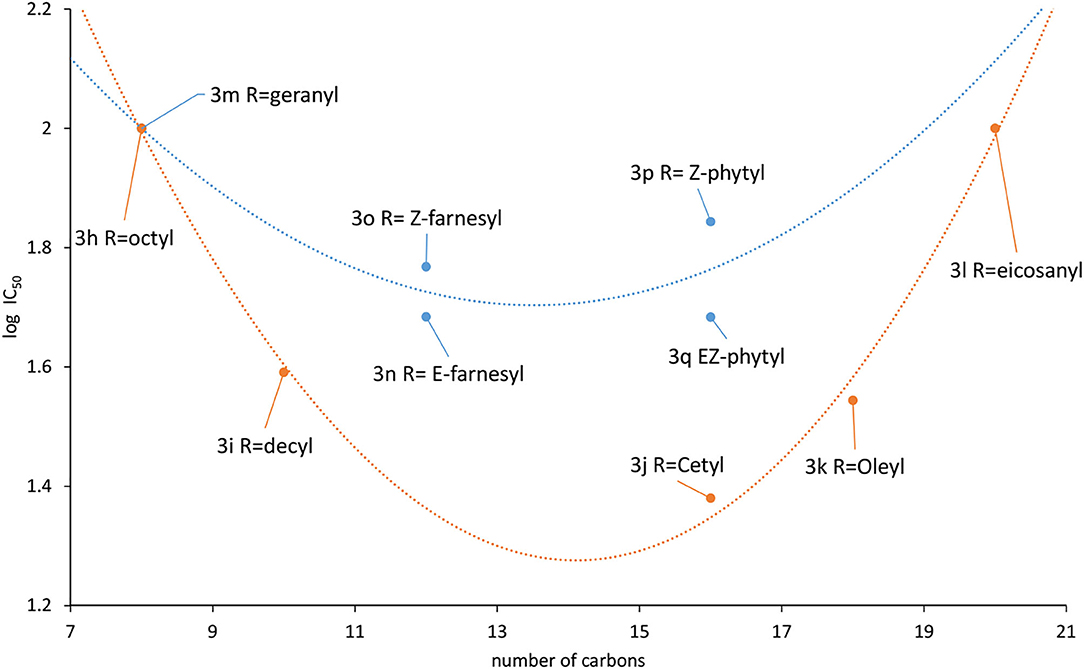
Figure 6. Correlation between activity and substituents chain length (blue: prenyl, orange: aliphatic).
These comparisons of the compounds have shown that most of them are considerable more active than L-thiazolidine-4-carboxylic acid (T4C), the only reported antichagasic proline derivative, with an IC50 of 890 μM on T. cruzi epimastigotes (Magdaleno et al., 2009). Analogs 3j, 3i, 3k, 3n were 36, 23, 22, and 18 times more active than T4C. That marked difference on the activity highlight the importance of the proline ring decoration on the antiparasitic activity.
The cytotoxicity of most active compounds (analogs 3i, 3k, and 3n) were tested in cultured monkey kidney Vero cells to estimate the selectivity toward the parasite. The IC50 were 43 μM, 17 μM and 14 μM, respectively, being an adequate concentration window for future studies in intracellular stages. The selectivity index, (calculated as IC50Vero cells / IC50 T. cruzi epimastigotes) were 1.13, 0.43 and 0.25 for compounds 3i, 3k, and 3n, respectively. This numbers shown a similar susceptibility to the mentioned compounds. The toxicity displayed may be linked to the proline transport inhibition, but that hypothesis must be validated. These results are not promising at this point to propose that compounds of our collection could be good candidates for anti-chagasic drug development. Nevertheless, the applied strategy settles the basis to design inhibitors against T. cruzi biological targets in both, the insect, and the mammalian stages.
Finally, to validate the L-proline transporter as the molecular target, the intracellular concentration of the amino acid was measured in competition assays with compounds that shown the best antiparasitic activity. Analogs 3i, 3k, and 3n were assayed. Interestingly, the proline uptake inhibition of those compounds did not follow the antiparasitic activity. The first hypothesis to explain that behavior was based on the presence of unsaturations on fatty tail. The difference between 3i and 3k relay on the unsaturation on the oleyl chain of the last. The structure of 3k shows a twisted conformation produced by the C9–double bond that also restricts the rotation around the neighbor bonds. Furthermore, the tail length of 3i and 3k differ in 6 carbon and the most active is the decyl analog (3i) in terms of transport inhibition, matching the behavior displayed in Figure 6. The E-farnesyl derivative 3n has a trimethyl substituted side-chain that is twelve carbon long, with three double bonds. Interestingly, this analog produced a complete inhibition of the proline uptake but is the less active of this series against T. cruzi. (Table 2) The fact that this analog was a superior inhibitor of the transporter could be the result of a better binding. Also, it could be attributed to the markedly different conformation of the analog due to the restricted rotation of the isoprenyl chain. Those restrictions should contribute to block the transporter once the proline region is recognized (Sayé et al., 2017). The inhibition of proline uptake by analogs 3i and 3n, resulted considerably more active than that inhibition by T4C (Magdaleno et al., 2009). Those differences were clearly related with the N1-alylated-1,2,3-triazolyl chain introduced on the proline.
Conclusions
In the present study, a strategy to design amino acids transport inhibitors was proposed. The uptake blocker is composed by a recognition motif, a linker and a bulky substituent or a membrane interacting portion. A set of seventeen 1,5-subtituted-1,2,3-triazole derivatives of methyl prolinate were prepared to validate the design. They were initially assayed against T. cruzi epimastigotes showing comparable potency than the control drug benznidazole. The antiparasitic activity profile of the series allowed us to establish a well-defined structural-activity relationship were the nature of the side-chain play a critical role. In order to validate the design, the inhibition of the proline uptake was studied with the analogs 3i, 3j, 3k, and 3n that displayed the best antiparasitic activity. The analogs with 3i (R=decyl) and 3n (R=E-farnesyl) produced a markedly reduction of the internalized proline. Those studies are strong evidence to validate our design of the transporter inhibitor that also linked the antiparasitic activity with the proline uptake.
The proline uptake has been explored as target for Chagas disease by drug repurposing. Using that approach, crystal violet has been identified as an interesting candidate (Sayé et al., 2020). Our approach is the first report of new compounds that have been design, prepared and validated as proline uptake blockers with antiparasitic activity. Unfortunately, the most active products displayed low selectivity toward the parasite, not being good candidates for future development as antichagasic drugs. Nevertheless, a complete study on the other T. cruzi life cycle stages could confirm or discard that hypothesis. The validated design of aminoacid transport inhibitor should open new applications on the study of physiological role of amino acid transporters in the central nervous system.
Data Availability Statement
The raw data supporting the conclusions of this article will be made available by the authors, without undue reservation.
Author Contributions
LF and EP-Z were responsible for the synthesis, purification and structural characterization of all the products. LF and MB performed the antiproliferative assays against Trypanosoma cruzi, carried out the proline transport inhibition assays, and interpreted the results. LF and LP performed the cytotoxicity assays on Vero cells. GL wrote most of the manuscript and supervised the study. GL, AS, and JC were the project leaders organizing and guiding experiments. All authors contributed to refining the manuscript and approved the final manuscript.
Funding
The authors wish to express their gratitude to UNR (Universidad Nacional de Rosario, Bio503), Agencia Nacional de Promoción Científica y Tecnológica (ANPCyT PICT- 2017-2096 awarded to GL) and Fundação de Amparo à Pesquisa do Estado de São Paulo grants 2016/06034-2 (awarded to AS) and Conselho Nacional de Pesquisas Científicas e Tecnológicas (CNPq) grants 404769/2018-7 and 308351/2013-4 (awarded to AS). The research leading to these results has, in part, received funding from the UK Research and Innovation via the Global Challenges Research Fund under grant agreement A Global Network for Neglected Tropical Diseases grant number MR/P027989/1. GL and JC are members of the Research Career of the Consejo Nacional de Investigaciones Científicas y Técnicas of Argentina (CONICET). LF, EP-Z, and LP thanks CONICET for the award of a Fellowship.
Conflict of Interest
The authors declare that the research was conducted in the absence of any commercial or financial relationships that could be construed as a potential conflict of interest.
Acknowledgments
This manuscript has been released as a pre-print at ChemRxiv (Fargnoli et al., 2019).
Supplementary Material
The Supplementary Material for this article can be found online at: https://www.frontiersin.org/articles/10.3389/fchem.2020.00696/full#supplementary-material
References
Agalave, S. G., Maujan, S. R., and Pore, V. S. (2011). Click Chemistry: 1,2,3-Triazoles as Pharmacophores. Chem. Asian J. 6, 2696–2718. doi: 10.1002/asia.201100432
Avila, C. C., Mule, S. N., Rosa-Fernandes, L., Viner, R., Barison, M. J., Costa-Martins, A. G., et al. (2018). Proteome-wide analysis of Trypanosoma cruzi exponential and stationary growth phases reveals a subcellular compartment-specific regulation. Genes 9:413. doi: 10.3390/genes9080413
Barison, M. J., Damasceno, F. S., Mantilla, B. S., and Silber, A. M. (2016). The active transport of histidine and its role in ATP production in Trypanosoma cruzi. J. Bioenerg. Biomembr. 48, 437–449. doi: 10.1007/s10863-016-9665-9
Barisón, M. J., Rapado, L. N., Merino, E. F., Pral, E. M. F., Mantilla, B. S., Marchese, L., et al. (2017). Metabolomics profiling reveals a finely tuned, starvation-induced metabolic switch in Trypanosoma cruzi epimastigotes. J. Biol. Chem. 292, 8964–8977. doi: 10.1074/jbc.M117.778522
Brener, Z., and Chiari, E. (1965). Aspects of early growth of different Trypanosoma cruzi strains in culture medium. J. Parasitol. 51, 922–926. doi: 10.2307/3275869
Burns, M. R., Graminski, G. F., Weeks, R. S., Chen, Y., and O'brien, T. G. (2009). Lipophilic lysine–spermine conjugates are potent polyamine transport inhibitors for use in combination with a polyamine biosynthesis inhibitor. J. Med. Chem. 52, 1983–1993. doi: 10.1021/jm801580w
Carland, J. E., Handford, C. A., Ryan, R. M., and Vandenberg, R. J. (2014). Lipid inhibitors of high affinity glycine transporters: Identification of a novel class of analgesics. Neurochem. Int. 73, 211–216. doi: 10.1016/j.neuint.2013.08.012
Carvalho, I., Andrade, P., Campo, V. L., Guedes, P. M., Sesti-Costa, R., Silva, J. S., et al. (2010). ‘Click chemistry’ synthesis of a library of 1,2,3-triazole-substituted galactose derivatives and their evaluation against Trypanosoma cruzi and its cell surface trans-sialidase. Bioorg. Med. Chem. 18, 2412–2427. doi: 10.1016/j.bmc.2010.02.053
Contreras Vt, A.-J. T., Bonaldo, M.c, Thomaz, N., Barbosa, H.s, Meirelles Mde, N., and Goldenberg, S. (1988). Biological aspects of the Dm 28c clone of Trypanosoma cruzi after metacyclogenesis in chemically defined media. Mem. Inst. Oswaldo Cruz. 83, 123–133. doi: 10.1590/S0074-02761988000100016
Crispim, M., Damasceno, F. S., Hernández, A., Barisón, M. J., Pretto Sauter, I., Souza Pavani, R., et al. (2018). The glutamine synthetase of Trypanosoma cruzi is required for its resistance to ammonium accumulation and evasion of the parasitophorous vacuole during host-cell infection. PLoS Negl. Trop. Dis. 12:e0006170. doi: 10.1371/journal.pntd.0006170
Fargnoli, L., Panozzo-Zénere, E. A., Pagura, L., Barisón, M. J., Cricco, J. A., Silber, A. M., et al. (2019). New L-proline uptake inhibitors with anti-Trypanosoma cruzi activity. ChemRxiv. doi: 10.26434/chemrxiv.7564991. [Epub ahead of print].
Gagneuz, A., Winstein, S., and Young, W. G. (1969). Rearrangement of allyl azides. J. Org. Chem. 1, 5956–5957. doi: 10.1021/ja01507a045
Gauchet, C., Labadie, G. R., and Poulter, C. D. (2006). Regio- and chemoselective covalent immobilization of proteins through unnatural amino acids. J. Am. Chem. Soc. 128, 9274–9275. doi: 10.1021/ja061131o
Girard, R. M. B. M., Crispim, M., Alencar, M. B., and Silber, A. M. (2018). Uptake of L-Alanine and Its Distinct Roles in the Bioenergetics of Trypanosoma cruzi. mSphere 3, e00338–e00318. doi: 10.1128/mSphereDirect.00338-18
Guedes, P. M. M., Silva, G. K., Gutierrez, F. R. S., and Silva, J. S. (2011). Current status of Chagas disease chemotherapy. Expert Rev. Anti Infect. Ther. 9, 609–620. doi: 10.1586/eri.11.31
Hamann, A. R., De Kock, C., Smith, P. J., Van Otterlo, W. A., and Blackie, M. A. (2014). Synthesis of novel triazole-linked mefloquine derivatives: biological evaluation against Plasmodium falciparum. Bioorg. Med. Chem. Lett. 24, 5466–5469. doi: 10.1016/j.bmcl.2014.10.015
Hannoush, R. N., and Sun, J. (2010). The chemical toolbox for monitoring protein fatty acylation and prenylation. Nat. Chem. Biol. 6, 498–506. doi: 10.1038/nchembio.388
Hauptmann, M., Wilson, D. F., and Erecinska, M. (1983). High affinity proline uptake in rat brain synaptosomes. FEBS Lett. 161, 301–305. doi: 10.1016/0014-5793(83)81029-1
Labadie, G. R., De La Iglesia, A., and Morbidoni, H. R. (2011). Targeting tuberculosis through a small focused library of 1,2,3-triazoles. Mol. Divers. 15, 1017–1024. doi: 10.1007/s11030-011-9319-0
Magdaleno, A., Ahn, I. Y., Paes, L. S., and Silber, A. M. (2009). Actions of a proline analogue, L-thiazolidine-4-carboxylic acid (T4C), on Trypanosoma cruzi. PLoS ONE 4:e4534. doi: 10.1371/journal.pone.0004534
Mantilla, B. S., Marchese, L., Casas-Sánchez, A., Dyer, N. A., Ejeh, N., Biran, M., et al. (2017). Proline metabolism is essential for trypanosoma brucei brucei survival in the tsetse vector. PLoS Path. 13:e1006158. doi: 10.1371/journal.ppat.1006158
Mantilla, B. S., Paes, L. S., Pral, E. M. F., Martil, D. E., Thiemann, O. H., Fernández-Silva, P., et al. (2015). Role of Δ1-Pyrroline-5-carboxylate dehydrogenase supports mitochondrial metabolism and host-cell invasion of Trypanosoma cruzi. J. Biol. Chem. 290, 7767–7790. doi: 10.1074/jbc.M114.574525
Marchese, L., Nascimento, J., Damasceno, F., Bringaud, F., Michels, P., and Silber, A. (2018). The uptake and metabolism of amino acids, and their unique role in the biology of pathogenic trypanosomatids. Pathogens 7:36. doi: 10.3390/pathogens7020036
Martins, R. M., Covarrubias, C., Rojas, R. G., Silber, A. M., and Yoshida, N. (2009). Use of L-proline and ATP production by Trypanosoma cruzi metacyclic forms as requirements for host cell invasion. Infect. Immun. 77, 3023–3032. doi: 10.1128/IAI.00138-09
Mckay, C. S., and Finn, M. G. (2014). Click chemistry in complex mixtures: bioorthogonal bioconjugation. Chem. Biol. 21, 1075–1101. doi: 10.1016/j.chembiol.2014.09.002
Morillo, C. A., Marin-Neto, J. A., Avezum, A., Sosa-Estani, S., Rassi, A. Jr., Rosas, F., et al. (2015). Randomized trial of benznidazole for chronic chagas' cardiomyopathy. New Engl. J. Med. 373, 1295–1306. doi: 10.1056/NEJMoa1507574
Nunes, M. C. P., Dones, W., Morillo, C. A., Encina, J. J., and Ribeiro, A. L. (2013). Chagas disease: an overview of clinical and epidemiological aspects. J. Am. Coll. Cardiol. 62, 767–776. doi: 10.1016/j.jacc.2013.05.046
Paes, L. S., Suárez Mantilla, B., Zimbres, F. M., Pral, E. M. F., Diogo De Melo, P., Tahara, E. B., et al. (2013). Proline dehydrogenase regulates redox state and respiratory metabolism in Trypanosoma cruzi. PLoS ONE 8:e69419. doi: 10.1371/journal.pone.0069419
Pereira, C. A., Alonso, G. D., Paveto, M. C., Iribarren, A., Cabanas, M. L., Torres, H. N., et al. (2000). Trypanosoma cruzi arginine kinase characterization and cloning: a novel energetic pathway in protozoan parasites. J. Biol. Chem. 275, 1495–1501. doi: 10.1074/jbc.275.2.1495
Pérez-Molina, J. A., and Molina, I. (2018). Chagas disease. Lancet 391, 82–94. doi: 10.1016/S0140-6736(17)31612-4
Pinard, E., Alanine, A., Alberati, D., Bender, M., Borroni, E., Bourdeaux, P., et al. (2010). Selective GlyT1 inhibitors: discovery of [4-(3-Fluoro-5-trifluoromethylpyridin-2-yl)piperazin-1-yl][5-methanesulfonyl-2-((S)-2,2,2-trifluoro-1-methylethoxy)phenyl]methanone (RG1678), a promising novel medicine to treat schizophrenia. J. Med. Chem. 53, 4603–4614. doi: 10.1021/jm100210p
Porta, E. O., Carvalho, P. B., Avery, M. A., Tekwani, B. L., and Labadie, G. R. (2014). Click chemistry decoration of amino sterols as promising strategy to developed new leishmanicidal drugs. Steroids 79, 28–36. doi: 10.1016/j.steroids.2013.10.010
Porta, E. O. J., Jäger, S. N., Nocito, I., Lepesheva, G. I., Serra, E. C., Tekwani, B. L., et al. (2017a). Antitrypanosomal and antileishmanial activity of prenyl-1,2,3-triazoles. Med. Chem. Comm. 8, 1015–1021. doi: 10.1039/C7MD00008A
Porta, E. O. J., Vallejos, M. M., Bracca, A. B. J., and Labadie, G. R. (2017b). Experimental and theoretical studies of the [3,3]-sigmatropic rearrangement of prenyl azides. RSC Adv. 7, 47527–47538. doi: 10.1039/C7RA09759J
Qosa, H., Mohamed, L. A., Alqahtani, S., Abuasal, B. S., Hill, R. A., and Kaddoumi, A. (2016). Transporters as drug targets in neurological diseases. Clin. Pharmacol. Ther. 100, 441–453. doi: 10.1002/cpt.435
Rohloff, P., Rodrigues, C. O., and Docampo, R. (2003). Regulatory volume decrease in Trypanosoma cruzi involves amino acid efflux and changes in intracellular calcium. Mol. Biochem. Parasitol. 126, 219–230. doi: 10.1016/S0166-6851(02)00277-3
Rostovtsev, V. V., Green, L. G., Fokin, V. V., and Sharpless, K. B. (2002). A stepwise huisgen cycloaddition process: copper(I)-catalyzed regioselective “ligation” of azides and terminal alkynes. Angew. Chem. Int. Ed. Engl. 41, 2596–2599. doi: 10.1002/1521-3773(20020715)41:14<2596::AID-ANIE2596>3.0.CO;2-4
Sayé, M., Fargnoli, L., Reigada, C., Labadie, G. R., and Pereira, C. A. (2017). Evaluation of proline analogs as trypanocidal agents through the inhibition of a Trypanosoma cruzi proline transporter. Biochim. Biophys. Acta-Gen. Subj. 1861, 2913–2921. doi: 10.1016/j.bbagen.2017.08.015
Sayé, M., Gauna, L., Valera-Vera, E., Reigada, C., Miranda, M. R., and Pereira, C. A. (2020). Crystal violet structural analogues identified by in silico drug repositioning present anti-Trypanosoma cruzi activity through inhibition of proline transporter TcAAAP069. PLoS Negl. Trop. Dis. 14:e0007481. doi: 10.1371/journal.pntd.0007481
Sayé, M., Miranda, M. R., Di Girolamo, F., De Los Milagros Cámara, M., and Pereira, C. A. (2014). Proline modulates the trypanosoma cruzi resistance to reactive oxygen species and drugs through a novel D, L-proline transporter. PLoS ONE 9:e92028. doi: 10.1371/journal.pone.0092028
Sayé, M., Reigada, C., Gauna, L., Valera-Vera, E. A., Pereira, C. A., and Miranda, M. R. (2019). Amino acid and polyamine membrane transporters in Trypanosoma cruzi: biological function and evaluation as drug targets. Curr. Med. Chem. 26, 6636–6651. doi: 10.2174/0929867326666190620094710
Schneider, C. A., Rasband, W. S., and Eliceiri, K. W. (2012). NIH image to imageJ: 25 years of image analysis. Nat. Methods 9, 671–675. doi: 10.1038/nmeth.2089
Silber, A. M., Colli, W., Ulrich, H., Alves, M. J., and Pereira, C. A. (2005). Amino acid metabolic routes in Trypanosoma cruzi: possible therapeutic targets against Chagas' disease. Curr. Drug Targets Infect. Disord. 5, 53–64. doi: 10.2174/1568005053174636
Silber, A. M., Tonelli, R. R., Lopes, C. G., Cunha-E-Silva, N., Torrecilhas, A. C. T., Schumacher, R. I., et al. (2009). Glucose uptake in the mammalian stages of Trypanosoma cruzi. Mol. Biochem. Parasitol. 168, 102–108. doi: 10.1016/j.molbiopara.2009.07.006
Silber, A. M., Tonelli, R. R., Martinelli, M., Colli, W., and Alves, M. J. M. (2002). Active transport of L-proline in trypanosoma cruzi. J. Eukaryot. Microbiol. 49, 441–446. doi: 10.1111/j.1550-7408.2002.tb00225.x
Sylvester, D., and Krassner, S. M. (1976). Proline metabolism in Trypanosoma cruzi epimastigotes. Comp. Biochem. Physiol. B: Biochem. Mol. Biol. 55, 443–447. doi: 10.1016/0305-0491(76)90318-7
Thompson, A. S., Humphrey, G. R., Demarco, A. M., Mathre, D. J., and Grabowski, E. J. J. (1993). Direct conversion of activated alcohols to azides using diphenyl phosphorazidate. A practical alternative to Mitsunobu conditions. J. Org. Chem. 58, 5886–5888. doi: 10.1021/jo00074a008
Tonelli, R. R., Silber, A. M., Almeida-De-Faria, M., Hirata, I. Y., Colli, W., and Alves, M. J. M. (2004). l-Proline is essential for the intracellular differentiation of Trypanosoma cruzi. Cell. Microbiol. 6, 733–741. doi: 10.1111/j.1462-5822.2004.00397.x
Tron, G. C., Pirali, T., Billington, R. A., Canonico, P. L., Sorba, G., and Genazzani, A. A. (2008). Click chemistry reactions in medicinal chemistry: applications of the 1,3-dipolar cycloaddition between azides and alkynes. Med. Res. Rev. 28, 278–308. doi: 10.1002/med.20107
Urbina, J. A. (2010). Specific chemotherapy of Chagas disease: Relevance, current limitations and new approaches. Acta Trop. 115, 55–68. doi: 10.1016/j.actatropica.2009.10.023
Keywords: Chagas disease, proline uptake, T. cruzi epimastigotes, cytotoxicity, target validation
Citation: Fargnoli L, Panozzo-Zénere EA, Pagura L, Barisón MJ, Cricco JA, Silber AM and Labadie GR (2020) Targeting L-Proline Uptake as New Strategy for Anti-chagas Drug Development. Front. Chem. 8:696. doi: 10.3389/fchem.2020.00696
Received: 02 April 2020; Accepted: 06 July 2020;
Published: 25 August 2020.
Edited by:
Gildardo Rivera, National Polytechnic Institute of Mexico (IPN), MexicoReviewed by:
Claudia Masini d'Avila-Levy, Oswaldo Cruz Foundation (Fiocruz), BrazilJuan Diego Maya, University of Chile, Chile
Copyright © 2020 Fargnoli, Panozzo-Zénere, Pagura, Barisón, Cricco, Silber and Labadie. This is an open-access article distributed under the terms of the Creative Commons Attribution License (CC BY). The use, distribution or reproduction in other forums is permitted, provided the original author(s) and the copyright owner(s) are credited and that the original publication in this journal is cited, in accordance with accepted academic practice. No use, distribution or reproduction is permitted which does not comply with these terms.
*Correspondence: Guillermo R. Labadie, labadie@iquir-conicet.gov.ar