- 1Department of Polymer Science and Engineering, Korea National University of Transportation, Chungju, South Korea
- 2Department of IT-Energy Convergence (BK21 FOUR), Chemical Industry Institute, Korea National University of Transportation, Chungju, South Korea
- 3Department of Energy Science and Engineering, Innovative Materials and Devices for Future Electronics/Power Sources (BK21 FOUR), Daegu Gyeongbuk Institute of Science and Technology (DGIST), Daegu, South Korea
Solar energy–driven carbon dioxide (CO2) reduction to valuable solar fuels/chemicals (e.g., methane, ethanol, and carbon monoxide) using particulate photocatalysts is regarded as one of the promising and effective approaches to deal with energy scarcity and global warming. The growth of nanotechnology plays an eminent role in improving CO2 reduction (CO2R) efficiencies by means of offering opportunities to tailor the morphology of photocatalysts at a nanoscale regime to achieve enhanced surface reactivity, solar light absorption, and charge separation, which are decisive factors for high CO2R efficiency. Notably, quantum dots (QDs), tiny pieces of semiconductors with sizes below 20 nm, offering a myriad of advantages including maximum surface atoms, very short charge migration lengths, size-dependent energy band positions, multiple exciton generation effect, and unique optical properties, have recently become a rising star in the CO2R application. In this review, we briefly summarized the progress so far achieved in QD-assisted CO2 photoreduction, highlighting the advantages of QDs prepared with diverse chemical compositions such as metal oxides, metal chalcogenides, carbon, metal halide perovskites, and MXenes.
Introduction
Carbon dioxide (CO2) is the major constituent of the global warming gases that are destroying the ozone layer of the Earth. Many researchers have been trying to capture and convert greenhouse gases, especially CO2, to make it as a pollution-free and recyclable energy source. In the present era, CO2 has been captured (Ibrahim et al., 2018; Fu et al., 2019; Omodolor et al., 2020; Dhoke et al., 2021; Lau et al., 2021), converted (Fu et al., 2019; Omodolor et al., 2020) and stored (Lau et al., 2021) by using different technologies. There are many methods and techniques that are studied for the conversion of CO2 into renewable energy sources; among them, photocatalytic CO2 reduction (CO2R) (Sorcar et al., 2018; Sorcar et al., 2019; Albero et al., 2020; Li et al., 2021), electrochemical CO2R (Jia et al., 2019; Liang et al., 2020), photo-biochemical CO2R (Kim et al., 2018), photo-electrochemical CO2R (Roy et al., 2016), and thermochemical CO2R (Maiti et al., 2018; Pullar et al., 2019) are well-known (He and Janáky, 2020). The photocatalysis process promotes the conversion reactions using clean solar energy, which is an eco-friendly CO2 conversion technology (shown in Figure 1A).
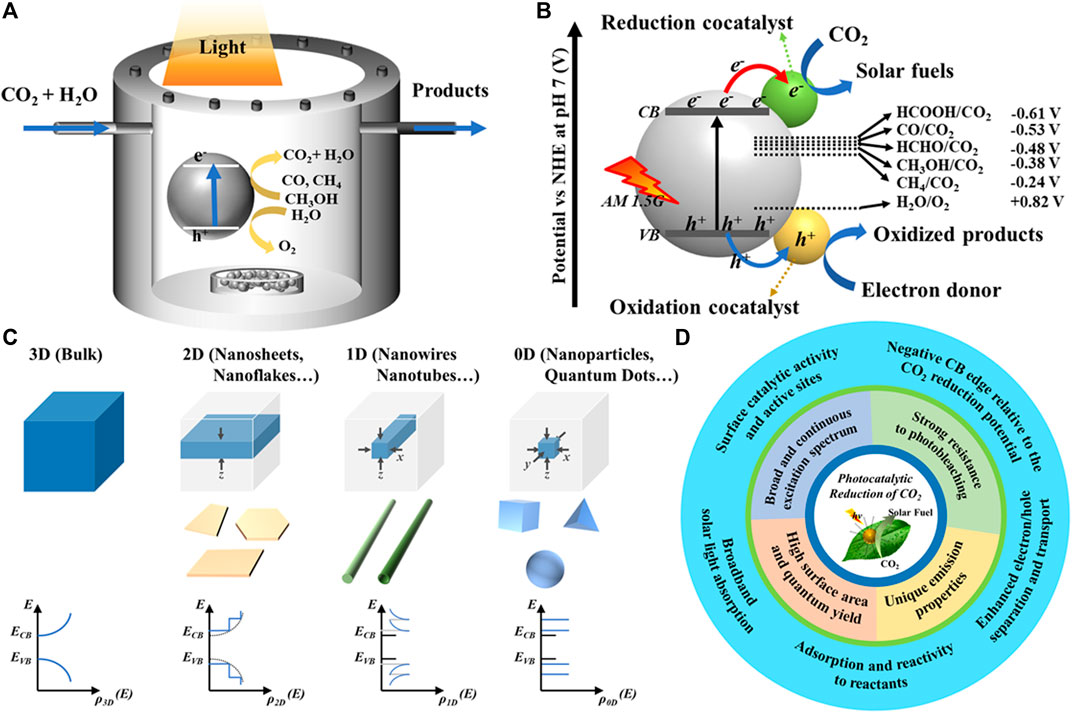
FIGURE 1. Schematic illustration of the (A) photocatalytic CO2 conversion method, (B) CO2 conversion by semiconducting photocatalyst, (C) density of states modification under different degrees of quantum confinement, and (D) the advantages of the QDs for the photocatalytic reduction of CO2.
The photocatalytic CO2R reaction comprises three primary steps: 1) the semiconductor photocatalyst absorbs the solar light energy and generates photocharge carriers, 2) photogenerated charge carriers were separated and transported to the surface of the semiconductor photocatalyst, and 3) oxidation and reduction reactions mediated by holes and electrons take place at the active sites on the photocatalyst surface, that is, electrons for CO2R and holes for oxidation of sacrificial agent or water, respectively (Xie et al., 2016; Wu et al., 2019) (Figure 1B). However, to exhibit the CO2 photoreduction, the photocatalyst should have the ability to adsorb CO2 and must possess its valence band (VB) at more positive potential than the water oxidation potential and conduction band (CB) at more negative potential than the CO2R potential (Xie et al., 2016). It should be noted that CO2R into CO2.─ radicals through single electrons transfer is unfavorable to occur because of the required high negative potential for the electrons in the CB of photocatalyst (−1.9 V vs NHE) (Shit et al., 2020). However, owing to relatively lower negative potential required for the conversion of CO2 into hydrocarbons, the proton-assisted multielectron transfer process is more favorable (Shit et al., 2020). Depending on the number of participated electrons, various gas and liquid phase hydrocarbons, such as carbon monoxide (CO), formic acid (CH2O2), oxalic acid (C2H2O4), formaldehyde (CH2O), acetaldehyde (C2H4O), methanol (CH3OH), methane (CH4), ethylene (C2H4), and ethanol (C2H5OH), are produced in the CO2R reaction (Shit et al., 2020) (Figure 1B). Major concerns existing for the efficient photocatalytic CO2R are 1) photocatalyst materials’ limited light absorption ability, 2) quick recombination of photogenerated charge carriers, and 3) poor adsorption of CO2 molecules on the photocatalyst surface. It was realized that crystalline phases, size, shape, and exposed facets of photocatalyst are crucially influencing the CO2R. Maneuvering semiconductors into distinct nanostructures results in significantly altered surfaces and electronic structures, which affect the surface reactivity and positions of energy bands, respectively (Figure 1C). Furthermore, the morphological features are also pivotal for the transfer of photocharge carriers. For instance, 2D nanosheet morphology having one dimension in the atomic thickness level facilitates shorter charge migration lengths, which is beneficial to avoid the quick recombination kinetics. Furthermore, their flat surface allows the facile heterojunction formation with other 2D, 1D, and 0D nanostructures. However, the quantum confinement in three dimension leaves 0D quantum dots (QDs) with very short charge migration lengths and maximum surface-exposed atoms (Figure 1C). The higher sensitivity of energy band positions to the size of QDs allows the precise tailoring of their VB and CB to the required positions in order to initiate the reduction of CO2 into selective hydrocarbons. Furthermore, the tiny size allows their easy grafting on other 2D and 1D nanostructures to frame the heterojunctions. Hence, QDs of a variety of semiconductors having several advantages garnered significant attention for the photocatalytic CO2R applications (Figure 1D).
Metal Oxide Quantum Dots
Metal oxide semiconductor materials such as MgO (Kohno et al., 2001), ZrO2 (Hengne et al., 2018; Miao et al., 2019), ZnO (Gokon et al., 2003), WO3 (Jin et al., 2015), and TiO2 (Kočí et al., 2009; Yu et al., 2014) have been studied as catalysts and co-catalysts for the photocatalytic reduction of CO2. In order to enhance the photocatalytic reduction of CO2, 0D metal oxide QDs (MOQDs) have been studied apart from their bulk counterparts due to their advantages like economical, eco-friendly, high surface area, good dispersibility, and well-maintained light absorption ability. For instance, the activity of CuO QDs is in good compatibility with Ti in the metal organic framework (MOF) MIL-125 coupled with g-C3N4 toward the efficient photocatalytic CO2R to form CO, CH3OH, CH3CHO, and C2H5OH (Li et al., 2020). The good compatibility between CuO QDs and active sites of Ti in MIL-125 the electrons generated by photocatalytic activity will easily transfer to CuO from MIL-125/g-C3N4. The combination of g-C3N4/CuO on MIL-125 has drastically improved the yield of CO, CH3OH, CH3CHO, and C2H5OH in the presence of water. However, most of the MOQDs have some unresolved technical issues such as the low yield of available electrons and large intrinsic bandgaps that are restricting the wide range applicability under visible light irradiation (Heng et al., 2021). Introduction of defects, doping, and heterojunction formation are the commonly practicing strategies to improve the CO2R efficiency of MOQDs.
Transition Metal Chalcogenide Quantum Dots
Transition metal chalcogenide (TMC) materials are formed by the combination of IV-VII transition metal elements (Mo, W, V, Nb, Ta, Ti, Zr, Hf, Tc, or Re) and chalcogens (S, Se, or Te). By the controlled synthesis of the TMCs from bulk to 2D nanosheets or 0D QDs, the bandgaps in TMCs can be tuned with respect to size and shape (Yao et al., 2019; Pandey et al., 2020). There are more than 40 kinds of TMCs available till date, which can be synthesized in large quantities by using synthesis techniques such as the CVD method (Bosi, 2015; Severs Millard et al., 2020), hydrothermal method (Chen and Fan, 2001), and Langmuir–Schaefer deposition method (Kalosi et al., 2019).
The TMCQDs and their composites such as CdS (Kuehnel et al., 2017), CdS/Ni (Wang et al., 2010), CdSe/TiO2 (Sarkar et al., 2016), PbS (Wang et al., 2011), ZnS/CuInS2 (Lian et al., 2018), and Mn:CdS/CdSeTe/TiO2 (Nie et al., 2018) have proven to be effective performing photocatalysts. Wang et al. reported the heterostructured catalyst CdSe/Pt/TiO2 for the photoreduction of CO2 under visible light in the presence of water (Wang et al., 2010). CdSe QD-sensitized TiO2 heterostructure materials are capable of catalyzing CO2R under visible light illumination (λ > 420 nm). The CdSe QD’s surface was modified by removing surfactant caps through annealing and using a hydrazine reducing agent, which enhanced the direct contact between CdSe QDs and TiO2. Although TMCQDs show good performance, they slowly become inactive after continuous exposure to the visible light illumination, which is a commonly observed issue in TMC(QD)s due to gradual oxidation of TMCs. The surface stoichiometry of the TMCQDs influences the exciton kinetics such as in CdSe QDs, the presence of a higher surface ratio of Se increases the possibility of electron–hole recombination at trap sites. The surface stoichiometry manipulation drives effective ways to improve the photocatalytic performance of TMCQDs.
Carbon Quantum Dots
Carbon QDs (CQDs), with their sizes in the range of 20 nm, have attracted much attention for their photoluminescence properties and co-catalyst role in different photocatalytic reactions. They exhibit low toxicity, good chemical stability, and exceptional water solubility compared to widely used semiconductor photocatalysts (CdS, TiO2, etc) (Murali et al., 2021). Importantly, CQDs possess upconversion photoluminescence property that allows the utilization of NIR light. All the aforementioned features and the high CO2 adsorption characteristics make CQDs an auspicious candidate for the photocatalytic CO2R application. Furthermore, surface functionalization with different organic functional groups tailors the semiconducting property and bandgap of CQDs to make them most suitable for CO2R. The functionalization of CQDs with 1,1′-bi(2-naphthylamine) enables the formation of intramolecular Z-scheme with a narrow bandgap for the efficient CO2R under visible light (Yan et al., 2018). Combining CQDs with other semiconductors is reported to enhance the CO2R efficiency by utilizing broad range of solar energy, where CQDs absorb visible light that enables the transfer of photogenerated charge carriers through the interface for efficient charge separation and improved CO2R. Specifically, heteroatom (N, B, S, Cl, etc.)-doped CQDs are more suitable to form the heterojunction owing to their enhanced light absorption, electron transport, chemical activity, and specific surface area properties. For instance, the N-rich CQDs/TiO2 composite showed an enhanced performance for the CO2R with CH4 and CO yield of 7.79 and 7.61 times higher than that of pristine TiO2 (Li et al., 2018).
Perovskite Quantum Dots
Metal halide perovskites are a class of semiconductors having ABX3 chemical stoichiometry, where A represents the alkali (e.g., Cs) or organic (e.g., formamidinium or methylammonium) cation; B denotes the divalent metal cation such as Pb, Bi, or Sn; and X stands for halide anions such as Cl, Br, or I. QDs of these materials are familiar for their excellent optical and electrical properties including strong light absorption, charge carrier’s high mobility and long diffusion lengths, and prolonged charge carrier lifetimes. The tuning of the cation/anion composition could facilitate the tailoring of the perovskite QD (PQD) absorption from UV to the NIR region (Shyamal and Pradhan, 2020). Furthermore, the favorable VB and CB positions of these CQDs enable the utilization of photogenerated charge carriers for CO2R prior to their recombination. However, selection of appropriate solvent for the photocatalytic CO2R over PQDs is a difficult task due to their instability upon exposure to polar solvents. Solvents like ethyl acetate have been selected because their mild polarity protects PQDs and CO2 is highly soluble in them (Xu et al., 2017). The addition of water to this solvent has been demonstrated to increase the selectivity of CO2R by minimizing H2 production (Shyamal and Pradhan, 2020). But an excessive amount of water addition will have negative impact on the stability of PQDs (Hou et al., 2017). However, the careful surface protection of cobalt-doped CsPbBr3/Cs4PbBr6 QDs with hexafluorobutyl methacrylate enabled the use of aqueous medium for the CO2R (Mu et al., 2019). Furthermore, to protect PQDs from contamination and to hinder their erosion by organic solvents, PQDs were encapsulated with metal oxides and MOFs while applying for CO2R (Zhang et al., 2016; Xu et al., 2018). The size optimization of PQDs is significant to accomplish the enhanced photocatalytic CO2R. The large size of PQDs decreases the surface area, while the smaller size leads to the aggregation, which will affect the optical absorption and charge carrier’s separation and transport properties. The CO2R performance of four different size (3.8, 6.1, 8.5, and 11.6 nm) CsPbBr3 PQDs in ethyl acetate/water medium under the solar illumination for 8 h concluded that PQDs with 8.5 nm size yielded more CH4, CO, and H2 products (Hou et al., 2017) (Figures 2A–F). The crystalline phase of PQDs influence the CO2R performance such as CsPbBr3 PQDs with the cubic phase are more active than the orthorhombic phase counterparts (Guo et al., 2019). The sluggish catalytic reaction dynamics of PQDs are dealt by employing a conducting material with high electron extraction efficiency (Xu et al., 2017; Pan et al., 2019).
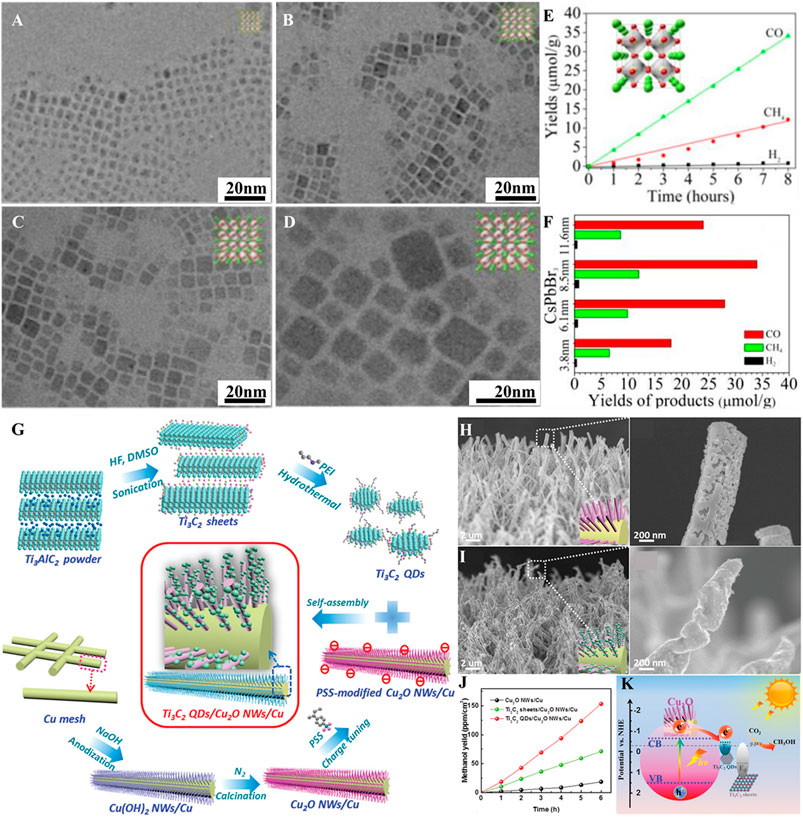
FIGURE 2. TEM images of CsPbBr3 QDs with particle sizes of (A) 3.8 nm, (B) 6.1 nm, (C) 8.5 nm, and (D) 11.6 nm (inset crystal structures). Photocatalytic CO2R for QDs with (E) 8.5 nm CsPbBr3 QDs and (F) CsPbBr3 QDs of different sizes (reproduced with permission from (Hou et al., 2017)). (G) Schematic illustration for synthesis of Ti3C2 QDs and Ti3C2 QDs/Cu2O NWs/Cu heterostructure, FE-SEM images of (H) Cu2O NWs/Cu, (i) Ti3C2 QDs/Cu2O NWs/Cu heterostructures, (J) CH3OH yield as a function of time, and (K) energy level diagram of Ti3C2 QDs/Cu2O NWs/Cu and Ti3C2 sheets/Cu2O NWs/Cu heterostructures (reproduced with permission from (Zeng et al., 2019)).
MXene Quantum Dots
MXenes, a set of 2D materials represented by a general formula of Mn+1XnTx (n = 1–4; X = C, N, and C/N; Tx = -O, -F, -OH, etc.), have exhibited a great potential in various applications owing to their exceptional electrical conductivity, metal-terminated surfaces, and hydrophilic characteristics (Lim et al., 2020; Tang et al., 2021). DFT calculations predicted that the chemisorption of CO2 is favorable compared to water on the MXene surface and higher electrical conductivity of MXene could cause the photocatalytic CO2R (Tahir et al., 2021). MXenes can be synthesized by the selective chemical etching of “A” layers from their sandwich-like parent MAX phase precursors, consisting of a stacked MXene nanosheets separated by the layers of A group elements. Recently, it has been demonstrated that appropriate experimental conditions could fragment the 2D MXenes into tiny pieces (≤10 nm), known as MXene QDs (MQDs). MQDs inherit all characteristics of their 2D counterparts and exhibit additional unique properties emanating from their high surface area and quantum size effects. MQDs absorb light in the range of UV to NIR and capable of effectively transforming the absorbed light energy into other forms, including chemical energy. Furthermore, the smaller size and hydrophilic/reactive surface functional groups permit easy grafting on other semiconductor nanostructures to make heterostructures. Recently, a facile incorporation of Ti3C2 MQDs onto Cu2O nanowires (NWs)/Cu mesh (Ti3C2 MQDs/Cu2O/Cu heterostructure) through a self-assembly approach was demonstrated to improve the CO2R (Zeng et al., 2019) (Figures 2G–K). The grafting of MQDs has improved the stability Cu2O NWs and led to significant enhancement in CO2R performance by improving light absorption and inhibiting the charge recombination. Furthermore, the CH3OH yield obtained with the Ti3C2 MQDs/Cu2O NWs/Cu photocatalyst is 8.25 and 2.15 times higher than Cu2O NWs/Cu and Ti3C2 sheets/Cu2O NWs/Cu photocatalysts, respectively. As the Fermi level (EF) of Ti3C2 MQDs is less negative than the CB of Cu2O, photogenerated charge carriers migrate from Cu2O to Ti3C2 MQDs and accumulate. The EF of MQDs is sufficiently negative to perform the reduction of CO2 to CH3OH, with accumulated electrons accelerating the CO2R. On the other hand, the EF of MXene nanosheets is positive, which is not suitable for accelerating the CO2R.
Conclusions and Perspectives
Photochemical CO2R is one of the efficient methods for the conversion of solar to fuel without releasing any toxic wastes into the environment. An ideal photocatalyst should have the qualities like a high surface area, more active sites, long-term stability, low cost, and easy to produce in industrial scale to commercialize. Several kinds of QDs such as MOQDs, TMCQDs, CQDs, PQDs, and MQDs have been studied so far for the photocatalytic CO2R. Overall, the research on QDs for CO2R is still in its infancy, and following aspects need to be addressed to reach further growth for the ease in applicability. The size control of most QDs involves complicated synthesis procedures. Developing a simple, cost-effective, size-controlled, and highly efficient synthesis approaches will lead to wide utilization of QDs for CO2R. Most of the TMCQDs and PQDs for CO2R are based on Cd- and Pb-containing compositions, respectively, which are not ideal in the perspective of safety and eco-friendliness. Hence, more research is needed for improving the stability and CO2R efficiency of Cd- and Pb-free QDs (such as InP, ZnSe, and ZnS) (Wang et al., 2019). The poor oxidation stability of the TMCQDs, PQDs, and MQDs in the presence of water and light are the major challenging aspects to be addressed immediately. The QD-based hybrids are mostly achieved by simple blending of QDs with other semiconductors, which does not generate a strong chemical interaction at the interfaces for efficient charge transfer process. Hence, in situ growth methodologies and/or external functionalization with different functional groups/molecules should be adopted to fully exploit the advantages of QDs. The full spectrum of solar light utilization by QDs for CO2R is not yet accomplished. More innovative technologies like making QDs comprising upconversion material as core should be investigated. At present, formic acid and CO are the main products of CO2R via two electron reduction. Hence, CO2R via four-, six-, and eight-electron reduction needs significant attention. Especially, methane production by eight-electron reduction can make a vital change in the application of QDs for CO2R. The H2 production via proton reduction that reduces the CO2R efficiency is another critical concern for the QD-based systems. Although the efficient properties of CQDs depends on the size, shape, surface defects, and heteroatom doping concentrations, a well-established method(s) for precise tuning is needed. Furthermore, the functional groups of CQDs are known to be reduced with the prolonged exposure of light during the photocatalytic reactions, which may influence their CO2R activity. Hence, improving the stability is a bottleneck concern in CQD-based CO2R research. The advancement in QDs stability under light, highly interactive interface with other materials, morphological control, and quick adoptability to the reaction environment will make them as futuristic materials for not only in CO2R but also in other interdisciplinary fields.
Author Contributions
All authors listed have made a substantial, direct, and intellectual contribution to the manuscript writing, editing, and reviewing work, and approved it for publication.
Funding
The authors acknowledge the support of the National research foundation of Korea (NRF) (2017R1E1A1A01074890, 2018R1A6A1A03023788, 2019R1A2C1010692 (MSIT), 2021R1I1A1A01055790, 2021R1A2C2009459, and 5199991614244 (BK21 FOUR)) and a Korea Institute for Advancement of Technology (KIAT) grant funded by the Korean government (MOTIE) (P00008500, the Competency Development Program for Industry Specialist).
Conflict of Interest
The authors declare that the research was conducted in the absence of any commercial or financial relationships that could be construed as a potential conflict of interest.
Publisher’s Note
All claims expressed in this article are solely those of the authors and do not necessarily represent those of their affiliated organizations, or those of the publisher, the editors and the reviewers. Any product that may be evaluated in this article, or claim that may be made by its manufacturer, is not guaranteed or endorsed by the publisher.
References
Albero, J., Peng, Y., and García, H. (2020). Photocatalytic CO2 Reduction to C2+ Products. ACS Catal. 10, 5734–5749. doi:10.1021/acscatal.0c00478
Bosi, M. (2015). Growth and Synthesis of Mono and Few-Layers Transition Metal Dichalcogenides by Vapour Techniques: A Review. RSC Adv. 5, 75500–75518. doi:10.1039/c5ra09356b
Chen, X., and Fan, R. (2001). Low-Temperature Hydrothermal Synthesis of Transition Metal Dichalcogenides. Chem. Mater. 13, 802–805. doi:10.1021/cm000517+
Dhoke, C., Zaabout, A., Cloete, S., and Amini, S. (2021). Review on Reactor Configurations for Adsorption-Based CO2 Capture. Ind. Eng. Chem. Res. 60, 3779–3798. doi:10.1021/acs.iecr.0c04547
Fu, H.-C., You, F., Li, H.-R., and He, L.-N. (2019). CO2 Capture and In Situ Catalytic Transformation. Front. Chem. 7, 525. doi:10.3389/fchem.2019.00525
Gokon, N., Hasegawa, N., Kaneko, H., Aoki, H., Tamaura, Y., and Kitamura, M. (2003). Photocatalytic Effect of ZnO on Carbon Gasification with CO2 for High Temperature Solar Thermochemistry. Solar Energ. Mater. Solar Cell 80, 335–341. doi:10.1016/j.solmat.2003.08.016
Guo, S.-H., Zhou, J., Zhao, X., Sun, C.-Y., You, S.-Q., Wang, X.-L., et al. (2019). Enhanced CO2 Photoreduction via Tuning Halides in Perovskites. J. Catal. 369, 201–208. doi:10.1016/j.jcat.2018.11.004
He, J., and Janáky, C. (2020). Recent Advances in Solar-Driven Carbon Dioxide Conversion: Expectations Versus Reality. ACS Energ. Lett. 5, 1996–2014. doi:10.1021/acsenergylett.0c00645
Heng, Z. W., Chong, W. C., Pang, Y. L., and Koo, C. H. (2021). An Overview of the Recent Advances of Carbon Quantum Dots/metal Oxides in the Application of Heterogeneous Photocatalysis in Photodegradation of Pollutants towards Visible-Light and Solar Energy Exploitation. J. Environ. Chem. Eng. 9, 105199. doi:10.1016/j.jece.2021.105199
Hengne, A. M., Samal, A. K., Enakonda, L. R., Harb, M., Gevers, L. E., Anjum, D. H., et al. (2018). Ni-Sn-Supported ZrO2 Catalysts Modified by Indium for Selective CO2 Hydrogenation to Methanol. ACS Omega 3, 3688–3701. doi:10.1021/acsomega.8b00211
Hou, J., Cao, S., Wu, Y., Gao, Z., Liang, F., Sun, Y., et al. (2017). Inorganic Colloidal Perovskite Quantum Dots for Robust Solar CO2 Reduction. Chem. Eur. J. 23, 9481–9485. doi:10.1002/chem.201702237
Ibrahim, M. H., El-Naas, M. H., Zhang, Z., and Van Der Bruggen, B. (2018). CO2 Capture Using Hollow Fiber Membranes: A Review of Membrane Wetting. Energy Fuels 32, 963–978. doi:10.1021/acs.energyfuels.7b03493
Jia, C., Dastafkan, K., Ren, W., Yang, W., and Zhao, C. (2019). Carbon-Based Catalysts for Electrochemical CO2 Reduction. Sustain. Energ. Fuels 3, 2890–2906. doi:10.1039/c9se00527g
Jin, J., Yu, J., Guo, D., Cui, C., and Ho, W. (2015). A Hierarchical Z-Scheme CdS-WO3 Photocatalyst with Enhanced CO2 Reduction Activity. Small 11, 5262–5271. doi:10.1002/smll.201500926
Kalosi, A., Demydenko, M., Bodik, M., Hagara, J., Kotlar, M., Kostiuk, D., et al. (2019). Tailored Langmuir-Schaefer Deposition of Few-Layer MoS2 Nanosheet Films for Electronic Applications. Langmuir 35, 9802–9808. doi:10.1021/acs.langmuir.9b01000
Kim, H.-W., Lee, K.-S., Razzaq, A., Lee, S. H., Grimes, C. A., and In, S.-I. (2018). Photocoupled Bioanode: A New Approach for Improved Microbial Fuel Cell Performance. Energy Technol. 6, 257–262. doi:10.1002/ente.201700177
Kočí, K., Obalová, L., Matějová, L., Plachá, D., Lacný, Z., Jirkovský, J., et al. (2009). Effect of TiO2 Particle Size on the Photocatalytic Reduction of CO2. Appl. Catal. B: Environ. 89, 494–502. doi:10.1016/j.apcatb.2009.01.010
Kohno, Y., Ishikawa, H., Tanaka, T., Funabiki, T., and Yoshida, S. (2001). Photoreduction of Carbon Dioxide by Hydrogen over Magnesium Oxide. Phys. Chem. Chem. Phys. 3, 1108–1113. doi:10.1039/b008887k
Kuehnel, M. F., Orchard, K. L., Dalle, K. E., and Reisner, E. (2017). Selective Photocatalytic CO2 Reduction in Water through Anchoring of a Molecular Ni Catalyst on CdS Nanocrystals. J. Am. Chem. Soc. 139, 7217–7223. doi:10.1021/jacs.7b00369
Lau, H. C., Ramakrishna, S., Zhang, K., and Radhamani, A. V. (2021). The Role of Carbon Capture and Storage in the Energy Transition. Energy Fuels 35, 7364–7386. doi:10.1021/acs.energyfuels.1c00032
Li, K., Teng, C., Wang, S., and Min, Q. (2021). Recent Advances in TiO2-Based Heterojunctions for Photocatalytic CO2 Reduction with Water Oxidation: A Review. Front. Chem. 9, 88. doi:10.3389/fchem.2021.637501
Li, M., Wang, M., Zhu, L., Li, Y., Yan, Z., Shen, Z., et al. (2018). Facile Microwave Assisted Synthesis of N-Rich Carbon Quantum Dots/Dual-Phase TiO2 Heterostructured Nanocomposites with High Activity in CO2 Photoreduction. Appl. Catal. B: Environ. 231, 269–276. doi:10.1016/j.apcatb.2018.03.027
Li, N., Liu, X., Zhou, J., Chen, W., and Liu, M. (2020). Encapsulating CuO Quantum Dots in MIL-125(Ti) Coupled with g-C3N4 for Efficient Photocatalytic CO2 Reduction. Chem. Eng. J. 399, 125782. doi:10.1016/j.cej.2020.125782
Lian, S., Kodaimati, M. S., and Weiss, E. A. (2018). Photocatalytically Active Superstructures of Quantum Dots and Iron Porphyrins for Reduction of CO2 to CO in Water. ACS Nano 12, 568–575. doi:10.1021/acsnano.7b07377
Liang, S., Altaf, N., Huang, L., Gao, Y., and Wang, Q. (2020). Electrolytic Cell Design for Electrochemical CO2 Reduction. J. CO2 Utilization 35, 90–105. doi:10.1016/j.jcou.2019.09.007
Lim, K. R. G., Handoko, A. D., Nemani, S. K., Wyatt, B., Jiang, H.-Y., Tang, J., et al. (2020). Rational Design of Two-Dimensional Transition Metal Carbide/Nitride (MXene) Hybrids and Nanocomposites for Catalytic Energy Storage and Conversion. ACS Nano 14, 10834–10864. doi:10.1021/acsnano.0c05482
Maiti, D., Hare, B. J., Daza, Y. A., Ramos, A. E., Kuhn, J. N., and Bhethanabotla, V. R. (2018). Earth Abundant Perovskite Oxides for Low Temperature CO2 Conversion. Energy Environ. Sci. 11, 648–659. doi:10.1039/c7ee03383d
Miao, Z., Hu, P., Nie, C., Xie, H., Fu, W., and Li, Q. (2019). ZrO2 Nanoparticles Anchored on Nitrogen-Doped Carbon Nanosheets as Efficient Catalyst for Electrochemical CO2 Reduction. J. Energ. Chem. 38, 114–118. doi:10.1016/j.jechem.2019.01.010
Mu, Y. F., Zhang, W., Guo, X. X., Dong, G. X., Zhang, M., and Lu, T. B. (2019). Water‐Tolerant Lead Halide Perovskite Nanocrystals as Efficient Photocatalysts for Visible‐Light‐Driven CO2 Reduction in Pure Water. ChemSusChem. 12, 4769–4774. doi:10.1002/cssc.201902192
Murali, G., Modigunta, J. K. R., Park, S., Lee, S., Lee, H., Yeon, J., et al. (2021). Enhancing Light Absorption and Prolonging Charge Separation in Carbon Quantum Dots via Cl-Doping for Visible-Light-Driven Photocharge-Transfer Reactions. ACS Appl. Mater. Inter. 13, 34648–34657. doi:10.1021/acsami.1c01879
Nie, R., Ma, W., Dong, Y., Xu, Y., Wang, J., Wang, J., et al. (2018). Artificial Photosynthesis of Methanol by Mn:CdS and CdSeTe Quantum Dot Cosensitized Titania Photocathode in Imine-Based Ionic Liquid Aqueous Solution. ChemCatChem. 10, 3342–3350. doi:10.1002/cctc.201800190
Omodolor, I. S., Otor, H. O., Andonegui, J. A., Allen, B. J., and Alba-Rubio, A. C. (2020). Dual-Function Materials for CO2 Capture and Conversion: A Review. Ind. Eng. Chem. Res. 59, 17612–17631. doi:10.1021/acs.iecr.0c02218
Pan, A., Ma, X., Huang, S., Wu, Y., Jia, M., Shi, Y., et al. (2019). CsPbBr3 Perovskite Nanocrystal Grown on MXene Nanosheets for Enhanced Photoelectric Detection and Photocatalytic CO2 Reduction. J. Phys. Chem. Lett. 10, 6590–6597. doi:10.1021/acs.jpclett.9b02605
Pandey, S. K., Das, R., and Mahadevan, P. (2020). Layer-Dependent Electronic Structure Changes in Transition Metal Dichalcogenides: The Microscopic Origin. ACS Omega 5, 15169–15176. doi:10.1021/acsomega.0c01138
Pullar, R. C., Novais, R. M., Caetano, A. P. F., Barreiros, M. A., Abanades, S., and Oliveira, F. A. C. (2019). A Review of Solar Thermochemical CO2 Splitting Using Ceria-Based Ceramics with Designed Morphologies and Microstructures. Front. Chem. 7, 601. doi:10.3389/fchem.2019.00601
Roy, N., Hirano, Y., Kuriyama, H., Sudhagar, P., Suzuki, N., Katsumata, K.-I., et al. (2016). Boron-Doped diamond Semiconductor Electrodes: Efficient Photoelectrochemical CO2 Reduction through Surface Modification. Sci. Rep. 6, 38010. doi:10.1038/srep38010
Sarkar, A., Gracia-Espino, E., Wågberg, T., Shchukarev, A., Mohl, M., Rautio, A.-R., et al. (2016). Photocatalytic Reduction of CO2 with H2O over Modified TiO2 Nanofibers: Understanding the Reduction Pathway. Nano Res. 9, 1956–1968. doi:10.1007/s12274-016-1087-9
Severs Millard, T., Genco, A., Alexeev, E. M., Randerson, S., Ahn, S., Jang, A.-R., et al. (2020). Large Area Chemical Vapour Deposition Grown Transition Metal Dichalcogenide Monolayers Automatically Characterized through Photoluminescence Imaging. npj 2D Mater. Appl. 4, 12. doi:10.1038/s41699-020-0146-y
Shit, S. C., Shown, I., Paul, R., Chen, K.-H., Mondal, J., and Chen, L.-C. (2020). Integrated Nano-Architectured Photocatalysts for Photochemical CO2 Reduction. Nanoscale 12, 23301–23332. doi:10.1039/d0nr05884j
Shyamal, S., and Pradhan, N. (2020). Halide Perovskite Nanocrystal Photocatalysts for CO2 Reduction: Successes and Challenges. J. Phys. Chem. Lett. 11, 6921–6934. doi:10.1021/acs.jpclett.0c00191
Sorcar, S., Hwang, Y., Lee, J., Kim, H., Grimes, K. M., Grimes, C. A., et al. (2019). CO2, Water, and Sunlight to Hydrocarbon Fuels: a Sustained Sunlight to Fuel (Joule-To-Joule) Photoconversion Efficiency of 1%. Energ. Environ. Sci. 12, 2685–2696. doi:10.1039/C9EE00734B
Sorcar, S., Thompson, J., Hwang, Y., Park, Y. H., Majima, T., Grimes, C. A., et al. (2018). High-rate Solar-Light Photoconversion of CO2 to Fuel: Controllable Transformation from C1 to C2 Products. Energ. Environ. Sci. 11, 3183–3193. doi:10.1039/c8ee00983j
Tahir, M., Ali Khan, A., Tasleem, S., Mansoor, R., and Fan, W. K. (2021). Titanium Carbide (Ti3C2) MXene as a Promising Co-Catalyst for Photocatalytic CO2 Conversion to Energy-Efficient Fuels: A Review. Energy & Fuels 35 (13), 10374–10404. doi:10.1021/acs.energyfuels.1c00958
Tang, X., Murali, G., Lee, H., Park, S., Lee, S., Oh, S. M., et al. (2021). Engineering Aggregation‐Resistant MXene Nanosheets as Highly Conductive and Stable Inks for All‐Printed Electronics. Adv. Funct. Mater. 31, 2010897. doi:10.1002/adfm.202010897
Wang, C., Thompson, R. L., Baltrus, J., and Matranga, C. (2010). Visible Light Photoreduction of CO2 Using CdSe/Pt/TiO2 Heterostructured Catalysts. J. Phys. Chem. Lett. 1, 48–53. doi:10.1021/jz9000032
Wang, C., Thompson, R. L., Ohodnicki, P., Baltrus, J., and Matranga, C. (2011). Size-Dependent Photocatalytic Reduction of CO2 with PbS Quantum Dot Sensitized TiO2 Heterostructured Photocatalysts. J. Mater. Chem. 21, 13452–13457. doi:10.1039/c1jm12367j
Wang, J., Xia, T., Wang, L., Zheng, X., Qi, Z., Gao, C., et al. (2018). Enabling Visible-Light-Driven Selective CO2 Reduction by Doping Quantum Dots: Trapping Electrons and Suppressing H2 Evolution. Angew. Chem. Int. Ed. 57, 16447–16451. doi:10.1002/anie.201810550
Wang, X.-D., Huang, Y.-H., Liao, J.-F., Jiang, Y., Zhou, L., Zhang, X.-Y., et al. (2019). In Situ Construction of a Cs2SnI6 Perovskite Nanocrystal/SnS2 Nanosheet Heterojunction with Boosted Interfacial Charge Transfer. J. Am. Chem. Soc. 141, 13434–13441. doi:10.1021/jacs.9b04482
Wu, H. L., Li, X. B., Tung, C. H., and Wu, L. Z. (2019). Semiconductor Quantum Dots: An Emerging Candidate for CO2 Photoreduction. Adv. Mater. 31, 1900709. doi:10.1002/adma.201900709
Xie, S., Zhang, Q., Liu, G., and Wang, Y. (2016). Photocatalytic and Photoelectrocatalytic Reduction of CO2 Using Heterogeneous Catalysts with Controlled Nanostructures. Chem. Commun. 52, 35–59. doi:10.1039/c5cc07613g
Xu, Y.-F., Yang, M.-Z., Chen, B.-X., Wang, X.-D., Chen, H.-Y., Kuang, D.-B., et al. (2017). A CsPbBr3 Perovskite Quantum Dot/Graphene Oxide Composite for Photocatalytic CO2 Reduction. J. Am. Chem. Soc. 139, 5660–5663. doi:10.1021/jacs.7b00489
Xu, Y. F., Wang, X. D., Liao, J. F., Chen, B. X., Chen, H. Y., and Kuang, D. B. (2018). Amorphous‐TiO2 ‐Encapsulated CsPbBr3 Nanocrystal Composite Photocatalyst with Enhanced Charge Separation and CO2 Fixation. Adv. Mater. Inter. 5, 1801015. doi:10.1002/admi.201801015
Yan, Y., Chen, J., Li, N., Tian, J., Li, K., Jiang, J., et al. (2018). Systematic Bandgap Engineering of Graphene Quantum Dots and Applications for Photocatalytic Water Splitting and CO2 Reduction. ACS Nano 12, 3523–3532. doi:10.1021/acsnano.8b00498
Yao, X., Wang, Y., Lang, X., Zhu, Y., and Jiang, Q. (2019). Thickness-Dependent Bandgap of Transition Metal Dichalcogenides Dominated by Interlayer van der Waals Interaction. Phys. E: Low-Dimens. Syst. Nanostructures 109, 11–16. doi:10.1016/j.physe.2018.12.037
Yu, J., Low, J., Xiao, W., Zhou, P., and Jaroniec, M. (2014). Enhanced Photocatalytic CO2-Reduction Activity of Anatase TiO2 by Coexposed {001} and {101} Facets. J. Am. Chem. Soc. 136, 8839–8842. doi:10.1021/ja5044787
Zeng, Z., Yan, Y., Chen, J., Zan, P., Tian, Q., and Chen, P. (2019). Boosting the Photocatalytic Ability of Cu2O Nanowires for CO2 Conversion by MXene Quantum Dots. Adv. Funct. Mater. 29, 1806500. doi:10.1002/adfm.201806500
Keywords: CO2 reduction, perovskite, MXene, quantum dots, carbon quantum dot, transition metal chalcogenide, metal oxide, photocatalyst
Citation: Park YH, Murali G, Modigunta JKR, In I and In S-I (2021) Recent Advances in Quantum Dots for Photocatalytic CO2 Reduction: A Mini-Review. Front. Chem. 9:734108. doi: 10.3389/fchem.2021.734108
Received: 30 June 2021; Accepted: 20 August 2021;
Published: 30 September 2021.
Edited by:
Jin Wang, Zhejiang Normal University, ChinaReviewed by:
Xubing Li, Technical Institute of Physics and Chemistry (CAS), ChinaMan Ou, Nanjing Tech University, China
Copyright © 2021 Park, Murali, Modigunta, In and In. This is an open-access article distributed under the terms of the Creative Commons Attribution License (CC BY). The use, distribution or reproduction in other forums is permitted, provided the original author(s) and the copyright owner(s) are credited and that the original publication in this journal is cited, in accordance with accepted academic practice. No use, distribution or reproduction is permitted which does not comply with these terms.
*Correspondence: Su-Il In, aW5zdWlsQGRnaXN0LmFjLmty